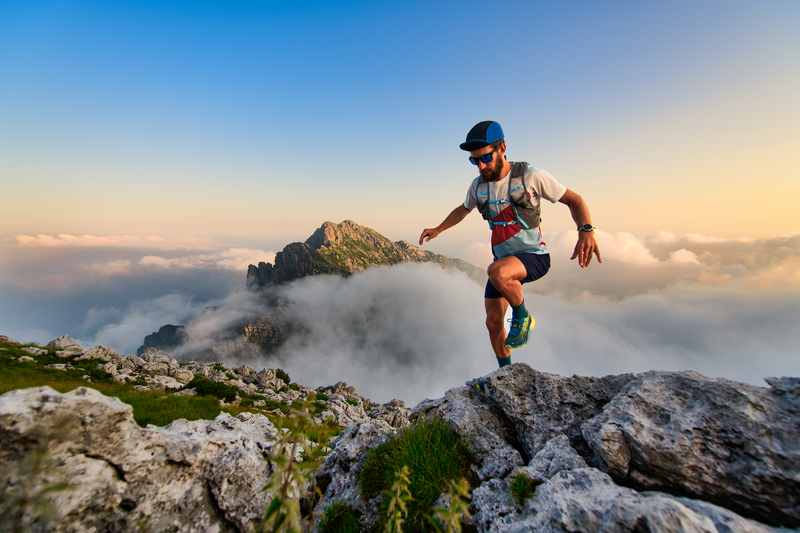
95% of researchers rate our articles as excellent or good
Learn more about the work of our research integrity team to safeguard the quality of each article we publish.
Find out more
ORIGINAL RESEARCH article
Front. Plant Sci. , 18 April 2017
Sec. Plant Breeding
Volume 8 - 2017 | https://doi.org/10.3389/fpls.2017.00544
This article is part of the Research Topic Optimizing Miscanthus for the sustainable bioeconomy: from genes to products View all 17 articles
Grasses such as Miscanthus × giganteus and Panicum virgatum (switchgrass) can potentially be used to produce bioenergy on a large scale in the Midwestern USA. The biomass productivity of these warm-season perennial grasses, particularly M. × giganteus, can be substantial, even when grown with limited inputs. The literature, however, varies regarding the nitrogen requirements for M. × giganteus biomass production. In addition, there is a lack of information that identifies the yield-component(s) (including total tiller number, tiller weight, total tiller diameter, total tiller height, phytomer number, reproductive tiller number, vegetative tiller number, reproductive tiller height, vegetative tiller height, reproductive tiller diameter, vegetative tiller diameter, and reproductive tiller phytomer number) that contributes to M. × giganteus biomass yields. Thus, the objective of this study was to examine the effects of fertilization on biomass yield and individual M. × giganteus plant-yield components. Plots of M. × giganteus were planted in 2008 in Urbana, IL, USA, and received annual applications of 0, 60, or 120 kg N ha-1. M. × giganteus productivity increased when nitrogen was applied; between 2011 and 2014, nitrogen applications of 60 or 120 kg N ha-1 produced average annual yields of 22.0 dry Mg ha-1 compared to 11.8 dry Mg ha-1 for unfertilized M. × giganteus. Both the total number of tillers per m2 and the tiller weight also increased as N-application rates increased. Our results indicate that increased reproductive tiller density and tiller weight with increased N fertilization increased M. × giganteus biomass yield.
When growing crops for cellulosic bioenergy, efficient production of high-yielding biomass feedstocks is a primary goal. In the U.S. Midwest, Miscanthus × giganteus Greef et Deu ex. Hodkinson et Renvoize (hereafter M. × giganteus), a sterile, warm-season, perennial grass, shows potential as a bioenergy crop due to its great biomass production (Heaton et al., 2008). M. × giganteus is a rhizomatous grass native to East Asia that was first cultivated as an energy crop in Europe in the early 1980s (Lewandowski et al., 2000). It is believed to be a cross between the fertile species M. sinensis and M. sacchariflorus (Hodkinson et al., 2002). As it is sterile, M. × giganteus must be propagated vegetatively using rhizome cuttings, rhizome-derived plugs, or in vitro micro propagation (Lewandowski, 1998; Anderson et al., 2011). Rhizome propagation has produced more robust plants than in vitro propagation (Lewandowski, 1998).
M. × giganteus has high yield potential. In Europe, M. × giganteus has produced 25 to 30 Mg ha-1 (Lewandowski et al., 2000). In the U.S., M. × giganteus biomass productivity from University of Illinois bioenergy studies has ranged between 15 and 30 Mg ha-1 in several Illinois field studies (Heaton et al., 2004, 2008; Maughan et al., 2012).
Nitrogen applications to M. × giganteus have had variable productivity results. Two long-termed M. × giganteus fertility studies in Europe found no productivity response to N fertilization over many years (Himken et al., 1997; Christian et al., 2008), while a third study reported a N response of biomass as the plot aged beyond 10 years (Clifton-Brown et al., 2007). The Illinois M. × giganteus studies were initially designed to compare M. × giganteus yields with those of switchgrass (Panicum virgatum L.) with no added fertility (Heaton et al., 2008). As the stands aged, M. × giganteus yields declined (Arundale et al., 2014a). However, when nitrogen was applied to the aged plots, previously unfertilized, M. × giganteus productivity increased as the N rates increased (Arundale et al., 2014b).
Grass phenotypic traits such as tiller density, tiller length, the number of phytomers per tiller [phytomers are vegetative units of grass shoots that include an internode, leaf, a portion of the node at the upper end, and a vegetative bud and portion of the node at the lower end (Beard and Beard, 2005)], the reproductive-to-vegetative tiller ratio, and tiller weight all play a role in determining productivity in herbaceous bioenergy crops. To date, these yield components have been evaluated and correlated with biomass productivity in switchgrass and prairie cordgrass (Spartina pectinata Link).
A study of three switchgrass cultivars showed strong correlation with increasing yield and both tiller density and phytomer mass, and weak correlation with the number of phytomers per tiller (Boe and Beck, 2008). Similar studies also found that the number of reproductive tillers per m2 and the number of phytomers per tiller were good selection criteria for increased biomass production of switchgrass (Boe, 2007). In addition, Boe (2007) also reported that switchgrass plants with greater numbers of large, reproductive stems tended toward higher yields (Boe, 2007). Das et al. (2004) reported a positive correlation between yield and tiller density. Much of the overall variation in switchgrass yield, therefore, results from genetic variability among cultivars (Boe and Beck, 2008). In prairie cordgrass, another warm-season rhizomatous perennial grass, Guo et al. (2015) found that tiller mass, tiller density, heading date, plant height, and phytomer number were all positively correlated with yield in some manner, but also found that much of the phenotypic variation was from the genetic diversity of the germplasm. With respect to the yield effect of nitrogen, Muir et al. (2001) reported that switchgrass tiller mass and tiller density responded positively to increased N fertilization and that tiller mass was more important than tiller density for biomass production. Similarly, Sanderson and Reed (2000) described that high N input increased individual switchgrass tiller weight, which increased biomass production.
There are conflicting results regarding M. × giganteus yield response to nitrogen fertilization, and the yield components that contribute to M. × giganteus biomass productivity are not well understood. Moreover, there are no reports of M. × giganteus yield components, N-fertilizer effects on yield components, and the yield component and N fertility roles on biomass productivity. Our central hypothesis was that N fertilization will increase one or more yield components and those components will contribute to M. × giganteus biomass. Therefore, the objective of this study was to examine the effects of fertilization on biomass yield and individual yield components in M. × giganteus.
The study site was located near Urbana, IL, USA, at the University of Illinois Energy Farm (40.0624 N, -88.1915 W) in Dana silt loam soil (fine-silty, mixed, superactive, mesic Oxyaquic Argiudolls). Before field planting in this study, M. × giganteus rhizomes (approximately 25 g) were collected from a field nursery at the University of Illinois Landscape Horticulture Research Center (Urbana, IL, USA) in 2007, and planted into pots (9 cm × 9 cm × 12 cm) using Sunshine Metro-Mix950®(Sun Gro Horticulture Distribution Inc., Hadley, MA, USA) as the growing medium. The potted M. × giganteus plants were grown in the University of Illinois greenhouse (Urbana, IL, USA) maintained at 27°C/16°C day/night temperature with 14 h photoperiod providing 400 μmol m-2s-1 photon flux at plant canopy level. In July 2008, potted M. × giganteus were planted by hand on one-meter spacing in twelve, 10 m × 10 m plots (100 plants per plot) with three nitrogen fertility treatments applied annually in early spring at or near the time of emergence at 0, 60, and 120 kg N ha-1 using urea as the N source (Maughan et al., 2012). Due to winterkill during the 2008–2009 winter the site was partially replanted in spring 2009 to fill plots to 100 plants each. The study was planted using a randomized complete block design with four replications, each comprised of the three N-application levels (Maughan et al., 2012).
This study reports on 2011–2014 growing-season findings. Biomass yields in 2010 were minimal (<3 Mg ha-1) and data were not included in this study. From 2011 to 2014, the study was harvested post-senescence after each growing season, between mid-December and March, which is the agronomic harvest timing for M. × giganteus grown as a bioenergy grass in Central Illinois. Biomass was cut by hand in 1-m2 quadrats with five replications per plot in senesced biomass harvests. Quadrats were selected throughout the plots in an attempt to produce samples representative of the plot as a whole and were not selected from border rows. Stems were cut at 10 cm and each quadrat was bundled individually. The biomass from each quadrat was measured for total plant fresh weight, subsample wet and oven-dry weights, vegetative tiller number (tiller m-2) and reproductive tiller number (tiller m-2). Five vegetative and five reproductive tillers were randomly selected from each of five replications per plot for yield components including tiller weight (g tiller-1), reproductive and vegetative tiller diameter (mm), reproductive and vegetative tiller height (cm), and reproductive and vegetative tiller phytomer number. Tiller diameter was measured at the midpoint of the lowest complete phytomer. Tiller height was measured to the top node of vegetative stems and to the base of the flower in reproductive stems. Dry biomass weight (PB, Mg ha-1) was determined by drying a 1.0 kg of subsample to 60°C for up to 72 h until dry weight was constant. Finally, we calculated nitrogen use efficiency (NUE) according to Delogu et al. (1998) and Lewandowski and Schmidt (2006), where NUE is the ratio of yield (yield at Nx-yield at N0) to N supply.
Weather data including precipitation and temperature was obtained from the Illinois State Climatologist and the Illinois state water survey 2015 (Illinois State Water Survey1). Precipitation and temperature records are shown for the location for the duration of the study (Table 1).
Data analysis including ANOVA, mean separation, and normality of the residuals and homogeneity of variances were performed in SAS software (SAS Institute, Cary, NC, USA). Biomass and yield components data were analyzed using Proc Mixed in SAS with N-rate (N), year (Y), and the interaction of N-rate and year (YN) were considered fixed effects and block as random. Tukey’s studentized range test was used to compare biomass yield and phenotypic traits at α = 0.05.
Monthly precipitation and temperature data for 2011–2014 are presented in Tables 1, 2, respectively. June 2012 precipitation was 45% below the 30-year average at 58 mm and July 2012 precipitation was 87% below the 30-year average at 15 mm, whereas August and September 2013 were 9.1 and 9.7 mm, which are 90% less than 30-year average (Table 1).
TABLE 2. Probability values from analysis of variance for biomass yield and yield components† of Miscanthus × giganteus affected by N rate during 2011–2014 at Urbana, IL, USA.
During 2011–2014, the main effects of N rate and year and their interaction effects were significant for biomass yield (Table 2). Biomass yield increased with increased N fertilization up to 60 kg N ha-1, and biomass yields between the two N fertilization rates (60 and 120 kg N ha-1) were not different. As interaction effects indicate, biomass yield generally appeared to decrease from 2011 to 2014 without N fertilization. However, biomass production was consistent throughout the years with N rate of 60 kg N ha-1 (Table 3). From 2011 to 2014, M. × giganteus plots fertilized at 60 kg and 120 kg N ha-1 produced average annual yields of 25.5 and 24.9 Mg ha-1, respectively, compared to 13.0 Mg biomass ha-1 from the unfertilized plots (Table 3).
TABLE 3. Miscanthus × giganteus biomass yield¶ and yield components† as affected by N fertilization rate during 2011–2014 at Urbana, IL, USA.
The main effects of N rate and year were significant for all biomass yield component traits except for the vegetative tiller number and vegetative tiller phytomer number, respectively, and N × year interactions were significant for total tiller number tiller weight, reproductive tiller height, reproductive tiller phytomer number, and vegetative tiller height (Table 2). In general, the values of all yield component traits increased with N fertilization except for the vegetative tiller number and vegetative tiller phytomer number, and differences between fertilized plots and unfertilized plots increased as the stands aged. However, no difference was observed between the two N rates (Table 3). In 2014, the reproductive tiller number was 24 and 42 tillers m-2 for 0 and 60 kg N ha-1, respectively, and tiller weight was 28 and 44 g tiller-1 for 0 and 60 kg N ha-1, respectively (Table 4). While the vegetative tiller number was not affected by N fertilization, total tiller number increased with N fertilization (Table 3). There was no difference in total tiller number among years, but total tiller number was lower in 2012, especially without N application (Table 3).
TABLE 4. Correlation coefficients between biomass yield and yield components† of M. × giganteus during 2011–2014 at Urbana, IL, USA.
The correlations between yield components and biomass yield in 2012, 2013, and 2014 were highly significant, exclusive of vegetative tiller number and vegetative tiller phytomer number in 2014 (Table 4). In 2011, there were weak, or no, observed correlations between yield components and biomass yield. Among biomass yield components, total tiller number, reproductive tiller number, and tiller weight were positively correlated with biomass yield and were the strongest indicators for biomass yield (Table 4). When correlation analysis between yield components and biomass yield were performed across years, the highest correlations were observed between reproductive tiller number and biomass yield (R2 = 0.6831) and tiller weight and biomass yield (R2 = 0.7517) (Figures 1A,B, respectively).
FIGURE 1. Linear regression of reproductive tiller number and biomass yield (A) and tiller weight and biomass yield (B) of Miscanthus × giganteus fertilized by N rates, 0, 60, and 120 kg N ha–1 during 2011–2014 at Urbana, IL, USA.
In this experiment, nitrogen fertilization increased M. × giganteus productivity during 2011–2014, but there were no yield differences between plots fertilized with 60 and 120 kg N ha-1. The positive responses to N fertilization are in agreement with Arundale et al. (2014b). Furthermore, N management is particularly essential for a biomass feedstock because N is associated with productivity and the cost of production (Vogel et al., 2002). The NUE was 0.3 Mg (kg N)-1 for the 60 kg N ha-1 treatments and 0.1 Mg (kg N)-1 for the 120 kg N ha-1 treatments. Increased N application rate led to a reduction in NUE, which is similar to the finding of Lewandowski and Schmidt (2006). However, many other studies reported that N fertilization is not required to achieve high M. × giganteus biomass yields (Himken et al., 1997; Lewandowski et al., 2000; Heaton et al., 2004; Christian et al., 2008). The different responses to N applications can be explained by the following: (1) Much of the M. × giganteus productivity research was conducted in Europe, and despite of the spatial variations, generally atmospheric N deposition rates are higher in Western Europe than in the USA (Holland et al., 2005). The topographical difference might affect soil N, which is thus related to N fertilization biomass yield response. (2) The reported absence of N fertilization effect could also be attributed to relatively short-termed experiments or to M. × giganteus growth during establishment years (Miguez et al., 2008). To produce 15 Mg ha-1 of M. × giganteus biomass, the N, P, and K requirements would be 92 kg N ha-1, 13 kg P ha-1, and 204 kg K ha-1 based on yearly crop off-take (Beale and Long, 1997). In addition, in Ercoli et al. (1999), it was implied that if N fertilizer was not supplied to the cropping system, there would be a reduction of biomass yield over long-termed growth. Conversely, if M. × giganteus is continuously harvested, there is N removal from the soil that should be compensated for by an external source of N. (3) Soil type, especially soil texture, can be an important factor for soil N availability. Even though the soil in our plots was classified as a silt loam soil by the USDA Soil Survey, based on our soil analysis (Maughan et al., 2012), this soil was a sandy loam soil with low CEC and N content. Biomass yield response to N fertilization in our study could be associated with low soil N retention as we observed no yield differences among N-fertilized plots during 2009–2010 (data not shown). Our results suggested that site-specific N management is necessary for sustainable biomass production of M. × giganteus.
Precipitation is the most important factor that directly and indirectly impacts aboveground biomass production in terrestrial ecosystems (Kardol et al., 2010), and roots are the primary connection between soil and soil water to plants (Clothier and Green, 1997; Xi et al., 2013). Plant biomass production positively responds to annual precipitation (Paruelo et al., 1999), and the seasonal precipitation pattern is a key factor in determining perennial grass establishment and biomass yield (Lee and Boe, 2005; Anderson et al., 2015). In addition, Richter et al. (2008) showed that growing season (April–September) precipitation and soil moisture capability are critical factors for perennial grass biomass production. Even though M. × giganteus is a warm-season, C4 grass with high water-use efficiency, biomass productivity can be affected by precipitation during the April–September growing season (Heaton et al., 2004). Anderson et al. (2011), found that M. × giganteus has little drought tolerance or the ability to cope with environments that receive limited precipitation. M. × giganteus roots have grown to an approximate depth of 1.8 m (Carroll and Somerville, 2009), and Neukirchen et al. (1999) reported that M. × giganteus produced 28% of total root biomass in the top 0.30 m soil depth with nearly 50% of the total roots growing in soil layers deeper than 0.90 m. Moreover, Chimento and Amaducci (2015) reported that roots of herbaceous crops, including giant reed, switchgrass and M. × giganteus, had more than 50% of the whole root biomass in the 30 cm of soil, and specifically, a substantial portion of M. × giganteus roots, including fine root biomass and root length density, was distributed in the upper soils. Conversely, Monti and Zatta (2009) wrote that compared to switchgrass where 35% were found in the upper 0.35 m soil, nearly 90% of total M. × giganteus roots were found in that soil layer.
With regard to N fertilization and water availability, Chimento and Amaducci (2015) reported that switchgrass root biomass was greater than that of giant reed, and Amaducci et al. (2017) found that switchgrass biomass production was impacted by water availability in fertilized plots, but not in unfertilized plots. Water availability affected the biomass yield of giant reed (Arundo donax L.) in both unfertilized and fertilized plots (Amaducci et al., 2017). Therefore, switchgrass had higher root biomass production than giant reed (Chimento and Amaducci, 2015), which resulted in less sensitivity to water availability than giant reed (Amaducci et al., 2017). On the other hand, Mann et al. (2013) wrote that switchgrass roots are likely to stretch deeply into areas of available soil moisture to overcome increasing moisture deficits that take place near the surface. In this experiment, the precipitation was variable during the 4-year time study period with much less precipitation than the 30-year average during June and July 2012 (32% of the 30-year average) and August and September 2013 (10% of 30-year average). We observed that M. × giganteus biomass yields declined in the unfertilized plots in 2012, while there were no yield reductions in the fertilized plots. It is possible that M. × giganteus tends to adopt a tolerance strategy (Lambers et al., 2008; Farooq et al., 2009) by relying on shallow rhizome production rather than mining deep wet soils. Limited rooting and root production in unfertilized M. × giganteus may have limited biomass production during dry growing seasons. Even though M. × giganteus is likely to exploit shallow rhizome production to overcome water-deficient conditions (Mann et al., 2013), applying N fertilization may help M. × giganteus to develop root structures, which may increase potential water uptake from the subsoil, and thereby overcome periods of low water availability in topsoil (Smika et al., 1961; Viets, 1962; Neukirchen et al., 1999). In addition, it has been reported that drought tolerance in plants could be enhanced by increased N fertilization (Halvorson and Reule, 1994; Fife and Nambiar, 1997; Van Schaik et al., 1997). For instance, N fertilization may alleviate drought stress by preventing cell membrane damage and improving osmoregulation (Saneoka et al., 2004).
Nitrogen fertilization is important for tiller, tiller density, and panicle development as well as for seed production in perennial grasses (Canode and Law, 1978; Haferkamp and Copeland, 1984; Thompson and Clark, 1989, 1993). In this study, N fertilization increased the total number of tillers and the ratio of reproductive tillers and vegetative tillers which resulted in increased tiller weight and biomass yield. This finding agrees with the results reported for switchgrass by Sanderson and Reed (2000) and Muir et al. (2001). Nitrogen fertilization increased tiller survival and N deficiency during early stages of tiller development seemed particularly unfavorable to tiller survival (Power and Alessi, 1978). For example, on average, M. × giganteus expanded vegetatively 0.15 m year-1 and tiller density within the center of a clone decreased as stands age, while tiller density increased toward the clone exterior (Matlaga et al., 2012). Therefore, enhanced N uptake, resulting from N-fertilization, may supply adequate amounts of various nutrients to individual tillers to ensure development and activation of the essential enzyme systems necessary for tiller survival and growth (Power and Alessi, 1978).
Pearson correlation coefficients revealed strong relationships between yield components and biomass yields (Table 4) and strong linear relationships occurred between biomass yields and total number of tillers, reproductive tillers, and tiller weights. Boe and Beck (2008) described that strong linear relationships have been observed between biomass yields and tiller density (tiller m-2) and tiller weight (mass tiller-1) in switchgrass. Das et al. (2004) suggested that tiller density per plant can be used as an indirect selection trait for increasing biomass yield, which can be applicable for M. × giganteus. Moreover, no relationship was found between biomass yields and the number of vegetative tillers, while the number of reproductive tillers was highly correlated with biomass yields, implying that as reproductive tiller increased, biomass yield also increased. With regard to reproductive tillers, Boe and Casler (2005) reported that biomass produced by high-yielding switchgrass cultivars contained predominately reproductive tillers with the maximum number of phytomers tiller-1, and low-yielding types mostly made up of a large number of vegetative tillers having fewer phytomers and lower weight phytomer-1 than reproductive tillers. Boe and Casler (2005) also wrote that switchgrass biomass yields at Madison, WI, USA, were much higher than at Brookings, SD, USA, with the differences resulting from the number of reproductive tillers; the reproductive tillers were approximately three times heavier than the vegetative tillers for cultivars of switchgrass across several environments. In this experiment, the total number of tillers between fertilized and unfertilized plots was significantly different, whereas the number of vegetative tillers was not affected by N fertilization. In addition, adding N fertilization led to an increased number of reproductive tillers, and a correlation between reproductive tiller numbers and biomass yield increased over years. Wilkins (1995) reported that the application of N resulted in the portion of reproductive tiller in perennial ryegrass. This indicates that the increased total tiller number resulted from an increase in reproductive tiller number over consecutive N applications, which ultimately increased the biomass yields.
There has been substantial interest in M. × giganteus as a bioenergy feedstock due to its high yield potential. Results from our 4-year field evaluation suggest that N fertilization might be necessary for sustainable biomass production with 60 kg N ha-1 being potentially adequate for maximum biomass yield. Nitrogen fertilization is necessary to maintain the tiller density and reproductive development, which are critical yield components for M. × giganteus biomass production. These findings indicate that determining optimal agronomic management could be a useful tool for improving M. × giganteus biomass yields.
M-SL, JG, and DL: Contributing substantial data analysis and interpretation for the work; revising the work for important intellectual content; approving final version to be published; and agreeing to be accountable. AW: Contributing substantial data acquisition, analysis, and interpretation for the work; drafting the work and revising the work critically for important intellectual content; approving final version to be published; and agreeing to be accountable. TV: Contributing substantial conception and design of the work; data analysis and interpretation for the work; revising the work for important intellectual content; approving final version to be published; and agreeing to be accountable.
This work was supported by the North Central Sun Grant Center with funding originating from U.S. Department of Energy and by the Energy Bioscience Institute.
The authors declare that the research was conducted in the absence of any commercial or financial relationships that could be construed as a potential conflict of interest.
We thank the North Central Sun Grant Feedstock Partnership, U.S. Department of Energy, Energy Biosciences Institute, University of Illinois Energy Farm, and the Illinois Experimental Station for supporting this research. We also thank Callan Beeson, Christopher Kunz, Timothy Mies, Rich Pyter, Drew Schlumpf, and Emily Thomas, for assisting with this study.
Amaducci, S., Facciotto, G., Beirgante, S., Perego, A., Serra, P., Ferrarini, A., et al. (2017). Biomass production and energy balance of herbaceous and woody crops on marginal soils in the Po valley. GCB Bioenergy 9, 31–45. doi: 10.1111/gcbb.12341
Anderson, E., Arundale, R., Maughan, M., Oladeinde, A., Wycislo, A., and Voigt, T. (2011). Growth and agronomy of Miscanthus × giganteus for biomass production. Biofuels 2, 167–183. doi: 10.4155/bfs.10.80
Anderson, E. K., Lee, D., Allen, D. J., and Voigt, T. B. (2015). Agronomic factors in the establishment of tetraploid seeded Miscanthus × giganteus. GCB Bioenergy 7, 1075–1083. doi: 10.1111/gcbb.12192
Arundale, R. A., Dohleman, F. G., Heaton, E. A., McGrath, J. M., Voigt, T. B., and Long, S. P. (2014a). Yields of Miscanthus × giganteus and Panicum virgatum decline with stand age in the Midwestern USA. GCB Bioenergy 6, 1–13. doi: 10.1111/gcbb.12077
Arundale, R. A., Dohleman, F. G., Voigt, T. B., and Long, S. P. (2014b). Nitrogen fertilization does significantly increase yields of stands of Miscanthus × giganteus and Panicum virgatum in multiyear trials in Illinois. Bioenergy Res. 7, 408–416. doi: 10.1007/s12155-013-9385-5
Beale, C. V., and Long, S. P. (1997). Seasonal dynamics of nutrient accumulation and partitioning in the perennial C4-grasses Miscanthus × giganteus and Spartina cynosuroides. Biomass Bioenergy 12, 419–428. doi: 10.1016/S0961-9534(97)00016-0
Beard, J. B., and Beard, H. J. (2005). Beard’s Turfgrass Encyclopedia. East Lansing, MI: Michigan State University Press.
Boe, A. (2007). Variation between two switchgrass cultivars for components of vegetative and seed biomass. Crop Sci. 47, 636–642. doi: 10.2135/cropsci2006.04.0260
Boe, A., and Beck, D. L. (2008). Yield components of biomass in switchgrass. Crop Sci. 48, 1306–1311. doi: 10.2135/cropsci2007.08.0482
Boe, A., and Casler, M. D. (2005). Hierarchical analysis of switchgrass morphology. Crop Sci. 45, 2465–2472. doi: 10.1007/s00122-010-1488-1
Canode, C. L., and Law, A. G. (1978). Influence of fertilizer and residue management on grass seed production. Agron. J. 70, 543–546. doi: 10.2134/agronj1978.00021962007000040006x
Carroll, A., and Somerville, C. (2009). Cellulosic biofuels. Annu. Rev. Plant Biol. 60, 165–182. doi: 10.1146/annurev.arplant.043008.092125
Chimento, C., and Amaducci, S. (2015). Characterization of fine root system and potential contribution to soil organic carbon of six perennial bioenergy crops. Biomass Bioenergy 83, 116–122. doi: 10.1016/j.biombioe.2015.09.008
Christian, D. G., Riche, A. B., and Yates, N. E. (2008). Growth, yield and mineral content of Miscanthus × giganteus grown as a biofuel for 14 successive harvests. Ind. Crops Prod. 28, 320–327. doi: 10.1016/j.indcrop.2008.02.009
Clifton-Brown, J. C., Breuer, J., and Jones, M. B. (2007). Carbon mitigation by the energy crop, Miscanthus. Glob. Change Biol. 13, 2296–2307. doi: 10.1111/j.1365-2486.2007.01438.x
Clothier, B. E., and Green, S. R. (1997). Roots: the big movers of water and chemicals in soil. Soil Sci. 162, 534–543. doi: 10.1097/00010694-199708000-00002
Das, M. K., Fuentes, R. G., and Taliaferro, C. M. (2004). Genetic variability and trait relationships in switchgrass. Crop Sci. 44, 443–448. doi: 10.2135/cropsci2004.4430
Delogu, G., Cattivelli, L., Pecchioni, N., De Falcis, D., Maggiore, T., and Stanca, A. M. (1998). Uptake and agronomic efficiency of nitrogen in winter barley and winter wheat. Eur. J. Agron. 9, 11–20. doi: 10.1016/S1161-0301(98)00019-7
Ercoli, L., Mariotti, M., Masoni, A., and Bonari, E. (1999). Effect of irrigation and nitrogen fertilization on biomass yield and efficiency of energy use in crop production of Miscanthus. Field Crops Res. 63, 3–11. doi: 10.1016/S0378-4290(99)00022-2
Farooq, M., Wahid, A., Kobayashi, N., Fujita, D., and Basra, S. M. A. (2009). Plant drought stress: effects, mechanisms and management. Agron. Sustain. Dev. 29, 185–212. doi: 10.1051/agro:2008021
Fife, D. N., and Nambiar, E. K. S. (1997). Changes in the canopy and growth of Pinus radiata in response to nitrogen supply. For. Ecol. Manag. 93, 137–152. doi: 10.1016/S0378-1127(96)03917-5
Guo, J., Thapa, S., Voigt, T., Rayburn, A. L., Boe, A., and Lee, D. K. (2015). Phenotypic and biomass yield variations in natural populations of prairie cordgrass (Spartina pectinata Link) in the USA. Bioenergy Res. 8, 1371–1383. doi: 10.1007/s12155-015-9604-3
Haferkamp, M. R., and Copeland, T. D. (1984). Shoot growth and development of Alamo switchgrass as influenced by mowing and fertilization. J. Range Manag. 37, 406–412. doi: 10.2307/3899625
Halvorson, A. D., and Reule, C. A. (1994). Nitrogen fertilizer requirements in an annual dryland cropping system. Agron. J. 86, 315–318. doi: 10.2134/agronj1994.00021962008600020020x
Heaton, E. A., Dohleman, F. G., and Long, S. P. (2008). Meeting US biofuel goals with less land: the potential of Miscanthus. Glob. Change Biol. 14, 2000–2014. doi: 10.1111/j.1365-2486.2008.01662.x
Heaton, E. A., Voigt, T., and Long, S. P. (2004). A quantitative review comparing the yields of two candidate C4 perennial biomass crops in relation to nitrogen, temperature and water. Biomass Bioenergy 27, 21–30. doi: 10.1016/j.biombioe.2003.10.005
Himken, M., Lammel, J., Neukirchen, D., Czypionka-Krause, U., and Olfs, H.-W. (1997). Cultivation of Miscanthus under West European conditions: seasonal changes in dry matter production, nutrient uptake and remobilization. Plant Soil 189, 117–126. doi: 10.1023/A:1004244614537
Hodkinson, T. R., Chase, M. W., Takahashi, C., Leitch, I. J., Bennett, M. D., and Renvoize, S. A. (2002). The use of DNA sequencing (ITS and TRNL-F), AFLP, and fluorescent in situ hybridization to study allopolyploid Miscanthus (Poaceae). Am. J. Bot. 89, 279–286. doi: 10.3732/ajb.89.2.279
Holland, E. A., Braswell, B. H., Sulzman, J., and Lamarque, J. F. (2005). Nitrogen deposition onto the United States and Western Europe: synthesis of observations and models. Ecol. Appl. 15, 38–57. doi: 10.1890/03-5162
Kardol, P., Campany, C. E., Souza, L., Norby, R. J., Weltzin, J. F., and Classen, A. T. (2010). Climate change effects on plant biomass alter dominance patterns and community evenness in an experimental old-field ecosystem. Glob. Change Biol. 16, 2676–2687. doi: 10.1111/j.1365-2486.2010.02162.x
Lambers, H., Chapin III, F. S., and Pons, T. L. (2008). Plant Physiological Ecology. 2nd Edn. New York, NY: Springer-Verlag.
Lee, D. K., and Boe, A. (2005). Biomass production of switchgrass in central South Dakota. Crop Sci. 45, 2583–2590. doi: 10.2135/cropsci2005.04-0003
Lewandowski, I. (1998). Propagation method as an important factor in the growth and development of Miscanthus × giganteus. Ind. Crops Prod. 8, 229–245. doi: 10.1016/S0926-6690(98)00007-7
Lewandowski, I., Clifton-Brown, J. C., Scurlock, J. M. O., and Huisman, W. (2000). Miscanthus: European experience with a novel energy crop. Biomass Bioenergy 19, 209–227. doi: 10.1016/S0961-9534(00)00032-5
Lewandowski, I., and Schmidt, U. (2006). Nitrogen, energy and land use efficiencies of miscanthus, reed canary grass and triticale as determined by the boundary line approach. Agric. Ecosyst. Environ. 112, 335–346. doi: 10.1016/j.agee.2005.08.003
Mann, J. J., Barney, J. N., Kyser, G. B., and DiTomaso, J. M. (2013). Root system dynamics of Miscanthus × giganteus and Panicum virgatum in response to rainfed and irrigated conditions in California. BioEnergy Res. 6, 678–687. doi: 10.1007/s12155-012-9287-y
Matlaga, D. P., Schutte, B. J., and Davis, A. S. (2012). Age-dependent demographic rates of the bioenergy crop Miscanthus × giganteus in Illinois. Invasive Plant Sci. Manag. 5, 238–248. doi: 10.1614/IPSM-D-11-00083.1
Maughan, M., Bollero, G., Lee, D. K., Darmody, R., Bonos, S., Cortese, L., et al. (2012). Miscanthus × giganteus productivity: the effects of management in different environments. GCB Bioenergy 4, 253–265. doi: 10.1111/j.1757-1707.2011.01144.x
Miguez, F. E., Villamil, M. B., Long, S. P., and Bollero, G. A. (2008). Meta-analysis of the effects of management factors on Miscanthus × giganteus growth and biomass production. Agric. For. Meteorol. 148, 1280–1292. doi: 10.1016/j.agrformet.2008.03.010
Monti, A., and Zatta, A. (2009). Root distribution and soil moisture retrieval in perennial and annual energy crops in Northern Italy. Agric. Ecosyst. Environ. 132, 252–259. doi: 10.1016/j.agee.2009.04.007
Muir, J. P., Sanderson, M. A., Ocumpaugh, W. R., Jones, R. M., and Reed, R. L. (2001). Biomass production of ‘Alamo’switchgrass in response to nitrogen, phosphorus, and row spacing. Agron. J. 93, 896–901. doi: 10.2134/agronj2001.934896x
Neukirchen, D., Himken, M., Lammel, J., Czypionka-Krause, U., and Olfs, H. W. (1999). Spatial and temporal distribution of the root system and root nutrient content of an established Miscanthus crop. Eur. J. Agron. 11, 301–309. doi: 10.1016/S1161-0301(99)00031-3
Paruelo, J. M., Lauenroth, W. K., Burke, I. C., and Sala, O. E. (1999). Grassland precipitation-use efficiency varies across a resource gradient. Ecosystems 2, 64–68. doi: 10.1007/s100219900058
Power, J. F., and Alessi, J. (1978). Tiller development and yield of standard and semidwarf spring wheat varieties as affected by nitrogen fertilizer. J. Agric. Sci. 90, 97–108. doi: 10.1017/S0021859600048632
Richter, G. M., Riche, A. B., Dailey, A. G., Gezan, S. A., and Powlson, D. S. (2008). Is UK biofuel supply from Miscanthus water-limited? Soil Use Manag. 24, 235–245. doi: 10.1111/j.1475-2743.2008.00156.x
Sanderson, M. A., and Reed, R. L. (2000). Switchgrass growth and development: water, nitrogen, and plant density effects. J. Range Manag. 53, 221–227. doi: 10.2307/4003287
Saneoka, H., Moghaieb, R. E., Premachandra, G. S., and Fujita, K. (2004). Nitrogen nutrition and water stress effects on cell membrane stability and leaf water relations in Agrostis palustris Huds. Environ. Exp. Bot. 52, 131–138. doi: 10.1016/j.envexpbot.2004.01.011
Smika, D. E., Haas, H. J., Rogler, G. A., and Lorenz, R. J. (1961). Chemical properties and moisture extraction in rangeland soils as influenced by nitrogen fertilization. J. Range Manag. 14, 213–216. doi: 10.2307/3895152
Thompson, D. J., and Clark, K. W. (1989). Influence of nitrogen fertilization and mechanical stubble removal on seed production of Kentucky bluegrass in Manitoba. Can. J. Plant Sci. 69, 939–943. doi: 10.4141/cjps89-114
Thompson, D. J., and Clark, K. W. (1993). Effects of clipping and nitrogen fertilization on tiller development and flowering in Kentucky bluegrass. Can. J. Plant Sci. 73, 569–575. doi: 10.4141/cjps93-077
Van Schaik, A. H., Struik, P. C., and Damian, T. G. (1997). Effects of irrigation and N on the vegetative growth of Aloe barbadensis Mill. in Aruba. Trop. Agric. 74, 104–109.
Viets, F. G. (1962). Fertilizers and the efficient use of water. Adv. Agron. 14, 223–264. doi: 10.1016/S0065-2113(08)60439-3
Vogel, K. P., Brejda, J. J., Walters, D. T., and Buxton, D. R. (2002). Switchgrass biomass production in the Midwest USA. Agron. J. 94, 413–420. doi: 10.2134/agronj2002.0413
Wilkins, P. W. (1995). Independence of dry matter yield and leaf yield among perennial ryegrass varieties differing in seasonal yield distribution. Grass Forage Sci. 50, 155–161. doi: 10.1111/j.1365-2494.1995.tb02308.x
Xi, B., Wang, Y., Jia, L., Bloomberg, M., Li, G., and Di, N. (2013). Characteristics of fine root system and water uptake in a triploid Populus tomentosa plantation in the North China Plain: implications for irrigation water management. Agric. Water Manag. 117, 83–92. doi: 10.1016/j.agwat.2012.11.006
Keywords: Miscanthus × giganteus, bioenergy, biomass productivity, nitrogen fertilization, yield components
Citation: Lee M-S, Wycislo A, Guo J, Lee DK and Voigt T (2017) Nitrogen Fertilization Effects on Biomass Production and Yield Components of Miscanthus × giganteus. Front. Plant Sci. 8:544. doi: 10.3389/fpls.2017.00544
Received: 02 December 2016; Accepted: 27 March 2017;
Published: 18 April 2017.
Edited by:
Luisa M. Trindade, Wageningen University and Research Centre, NetherlandsReviewed by:
Olena Kalinina, University of Hohenheim, GermanyCopyright © 2017 Lee, Wycislo, Guo, Lee and Voigt. This is an open-access article distributed under the terms of the Creative Commons Attribution License (CC BY). The use, distribution or reproduction in other forums is permitted, provided the original author(s) or licensor are credited and that the original publication in this journal is cited, in accordance with accepted academic practice. No use, distribution or reproduction is permitted which does not comply with these terms.
*Correspondence: Thomas Voigt, dHZvaWd0QGlsbGlub2lzLmVkdQ==
Disclaimer: All claims expressed in this article are solely those of the authors and do not necessarily represent those of their affiliated organizations, or those of the publisher, the editors and the reviewers. Any product that may be evaluated in this article or claim that may be made by its manufacturer is not guaranteed or endorsed by the publisher.
Research integrity at Frontiers
Learn more about the work of our research integrity team to safeguard the quality of each article we publish.