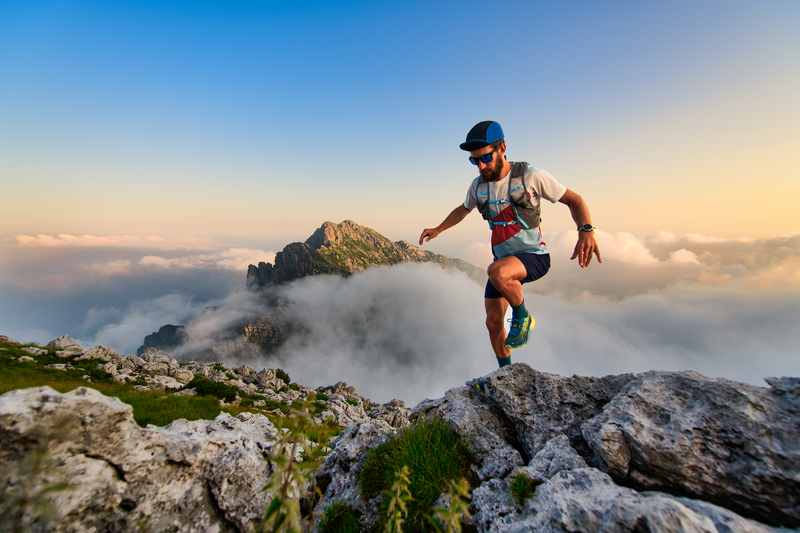
95% of researchers rate our articles as excellent or good
Learn more about the work of our research integrity team to safeguard the quality of each article we publish.
Find out more
ORIGINAL RESEARCH article
Front. Plant Sci. , 17 January 2017
Sec. Plant Physiology
Volume 7 - 2016 | https://doi.org/10.3389/fpls.2016.01992
The search for novel stress tolerance determinants has led to increasing interest in plants native to extreme environments – so called “extremophytes.” One successful strategy has been comparative studies between Arabidopsis thaliana and extremophyte Brassicaceae relatives such as the halophyte Eutrema salsugineum located in areas including cold, salty coastal regions of China. Here, we investigate stress tolerance in the desert species, Anastatica hierochuntica (True Rose of Jericho), a member of the poorly investigated lineage III Brassicaceae. We show that A. hierochuntica has a genome approximately 4.5-fold larger than Arabidopsis, divided into 22 diploid chromosomes, and demonstrate that A. hierochuntica exhibits tolerance to heat, low N and salt stresses that are characteristic of its habitat. Taking salt tolerance as a case study, we show that A. hierochuntica shares common salt tolerance mechanisms with E. salsugineum such as tight control of shoot Na+ accumulation and resilient photochemistry features. Furthermore, metabolic profiling of E. salsugineum and A. hierochuntica shoots demonstrates that the extremophytes exhibit both species-specific and common metabolic strategies to cope with salt stress including constitutive up-regulation (under control and salt stress conditions) of ascorbate and dehydroascorbate, two metabolites involved in ROS scavenging. Accordingly, A. hierochuntica displays tolerance to methyl viologen-induced oxidative stress suggesting that a highly active antioxidant system is essential to cope with multiple abiotic stresses. We suggest that A. hierochuntica presents an excellent extremophyte Arabidopsis relative model system for understanding plant survival in harsh desert conditions.
Abiotic stresses dramatically reduce crop yields leading to huge economic losses and a threat to food security (Boyer, 1982; Bray et al., 2000; Cramer et al., 2011), a problem likely to be exacerbated by climate change (Mittler and Blumwald, 2010; Lobell et al., 2011; Lesk et al., 2016). At the same time, the worldwide human population is continuously growing; from 7 billion in late 2011, it is expected to surpass 9 billion people by 2050 (United Nations, Department of Economic and Social Affairs, Population Division, 2011). Consequently, the demand for global crop production is expected to double by 2050 (Tilman et al., 2011). Yet, most new land with the potential for agriculture is situated in harsh environments such as drylands and deserts, which cover about 41% of the world land areas (Millennium Ecosystem Assessment, 2005). Therefore, the improvement of crop yields under multiple stresses is vital to prevent losses where crops are currently grown and to cultivate them on more marginal land.
The model plant, Arabidopsis thaliana has provided much of our understanding of the molecular responses of plants to stress. However, Arabidopsis is a stress-sensitive plant and thus the search for novel stress tolerance mechanisms and genes has led to increasing interest in naturally stress-tolerant plants native to extreme environments – so called “extremophytes” (John and Spangenberg, 2005; Amtmann, 2009; Dassanayake et al., 2009; Smaoui et al., 2011; Gechev et al., 2012; Oh et al., 2012; Bressan et al., 2013; Cheeseman, 2014; Flowers et al., 2015; Zhu et al., 2015). One successful strategy has been to study extremophyte Arabidopsis relatives (Brassicaceae) such as the metal hyperaccumulator Arabidopsis halleri (Hanikenne et al., 2008), and the halophytes, Schrenkiella parvula (formerly Thellungiella parvula) and Eutrema salsugineum (formerly Thellungiella salsuginea or Thellungiella halophila) (Volkov et al., 2003; Inan et al., 2004; Orsini et al., 2010; Koch and German, 2013). In addition to being salt-tolerant (e.g., Inan et al., 2004; Taji et al., 2004; Kant et al., 2006), E. salsugineum is also tolerant to low soil nitrogen (Kant et al., 2008), high boron levels (Lamdan et al., 2012), low phosphate levels (Velasco et al., 2016), heat stress (Higashi et al., 2013), and shows similar tolerance to cold and freezing stress as Arabidopsis (Griffith et al., 2007; Lee et al., 2012). A number of factors contribute to E. salsugineum stress tolerance including constitutive up- and down-regulation of stress tolerance genes and metabolites suggesting that E. salsugineum is “primed” for stress, gene copy number expansion of ion transport-related genes, sub-functionalization and neo-functionalization of duplicated genes, biased codon usage facilitating more efficient translation of proteins related to ion transportation, and the possible involvement of lineage-specific genes (Taji et al., 2004; Gong et al., 2005; Kant et al., 2006, 2008; Lugan et al., 2010; Oh et al., 2010, 2012, 2014; Sun et al., 2010; Dassanayake et al., 2011; Wu et al., 2012; Champigny et al., 2013; Kazachkova et al., 2013; Yang et al., 2013).
The various stresses to which an extremophyte exhibits tolerance reflect the combination of stresses characteristic of each specific habitat. Thus, to obtain a comprehensive understanding of how plants cope with multiple stresses, it is vital to investigate extremophytes from a range of different habitats. The Negev desert in Southern Israel, part of the Sahara and Arabian deserts belt, is characterized by a combination of temperature extremes, low water and nutrient availability, and high salinity and radiation levels, and is considered an “arid” zone with an annual rainfall of between 25 and 200 mm that is unpredictable with high spatial and temporal variation (Gutterman, 2002; Ward, 2009). Temperatures can vary both daily and seasonally between an absolute daily maximum and minimum of 46.8 and -3.6°C, respectively1. These extreme conditions, together with “islands of soil salinity,” have led to special morphological, physiological and genetic adaptations that allow plants to thrive. Thus, the Negev desert is a likely biogenetic treasure box of genes that are related to abiotic stress adaptations.
We have screened several of the 25 extremophyte Brassicaceae species that inhabit the Negev for tolerance to multiple abiotic stresses. One of these is Anastatica hierochuntica (True Rose of Jericho), a winter annual, Saharo-Arabian desert plant species first described by Linnaei (1753) that colonizes the uppermost, driest zones of wadies or runnels (Friedman and Stein, 1980; Friedman et al., 1981). A. hierochuntica is well-known due to its hygrochasy seed dispersal mechanism whereby the plant dies, dries out and reduction in turgor pressure causes the branches to curl thereby protecting the seeds and preventing their dispersal (Friedman et al., 1978; Hegazy et al., 2006). Once a serious rain event occurs causing wetting of the dead plant, the branches unfold and the seed capsules (siliculae) open. Seeds are then dispersed by raindrops hitting the open siliculae (Gutterman, 2002; Hegazy et al., 2006). Thus, although dead, A. hierochuntica has often been mistaken for a resurrection plant.
Here, we examine whether A. hierochuntica exhibits tolerance to heat, low nitrate and salt stresses associated with its desert habitat. Taking salt stress as a case study, we determine whether A. hierochuntica possesses physiological salt tolerance mechanisms that are similar to its halophytic relative, E. salsugineum, and whether these two extremophytes from very different environments have evolved common or/and species-specific metabolic strategies for coping with salt stress.
Anastatica hierochuntica seeds were originally collected from the Negev Desert (455881′ N and 200751′ E), Israel. The original seed was bulked at the Ben-Gurion University experimental fields in Midreshet Ben-Gurion, and seeds from this collection were further propagated under growth room conditions (16 h light (150 μmol m-2 s-1)/8 h dark; 22°C). Seeds from the F4 generation were used for experiments presented in the current report. A. thaliana (Col-0), A. hierochuntica and E. salsugineum (Shandong ecotype) seeds were surface-sterilized with 50% commercial bleach for 5 min, and then rinsed four times with sterile water. A. hierochuntica seeds were sown onto nutrient agar plates containing MS (Murashige and Skoog, 1962) medium, pH 5.7, 0.5 g L-1 MES, 2% (w/v) sucrose and 0.8% (w/v) agar (Duchefa Biochemie), while Arabidopsis and E. salsugineum seeds were suspended in 0.12% agarose prior to being sown on plates. Seeds were stratified at 4°C (Arabidopsis, 4 days; E. salsugineum, 7 days; A. hierochuntica, 1 day) and plates incubated in the growth room [16 h light (150 μmol m-2 s-1)/8 h dark; 22°C]. Sowing of seeds from the three species was staggered so that germination occurred at approximately the same time. For soil experiments, seedlings with fully expanded cotyledons were transferred from plates to 7 cm × 7 cm × 8 cm pots containing Arabidopsis soil (Weizmann Institute of Science; 70% fine peat [1–10 mm], 30% perlite 4) and irrigated to field capacity with 1 g L-1 of 20-20-20 NPK + micronutrients solution (Haifa chemicals). Flats containing pots were covered with plastic domes and placed in the growth room. After 2 days, domes were gradually removed to allow seedling hardening. Each day, plates or pots were shuffled to remove shelf position effects. Although for the purposes of comparative studies with Arabidopsis and E. salsugineum, light conditions in the growth room were below those usually encountered by A. hierochuntica, Supplementary Figure S1 shows that A. hierochuntica growth rates always remain lower than Arabidopsis even at higher light intensities. This suggests that the slower growth of A. hierochuntica relative to Arabidopsis and E. salsugineum was not an effect of the growth room light conditions.
For plate-based in vitro analyses, heat stress (45°C) was applied for various periods and plates transferred back to the growth room for an additional 48 h. For salt stress, low NO3- and oxidative stresses, seedlings were first sown on control MS plates. Due to differing root growth rates, A. hierochuntica and Arabidopsis seedlings were transferred to stress treatment plates, 1 and 5 days after stratification, respectively. For the root elongation assay, 10 uniform seedlings were transferred to MS vertical square plates containing various NaCl concentrations. To prevent any osmotic effects due to uptake of sucrose in the aerial tissues (MacGregor et al., 2008), the top of the agar was cut away before transfer of seedlings to ensure that shoots were not in contact with the medium. For the low NO3- and oxidative stress assays, uniform Arabidopsis or A. hierochuntica seedlings were transferred for 5 days to vertical square plates containing MS macroelements (minus ammonium nitrate for low N experiments) and microelements, pH 5.7 (adjusted with KOH), 0.5 g L-1 MES, 2% (w/v) sucrose, 0.8% (w/v) agar (Duchefa Biochemie), and various concentrations of either KNO3- or methyl viologen (MV; Sigma, M-2254).
For soil experiments, salt treatments were applied to plants with four true leaves (Arabidopsis and E. salsugineum) or two true leaves (A. hierochuntica). Pots were irrigated with 1 g L-1 of 20-20-20 NPK + micronutrients solution supplemented with various NaCl concentrations. Salt treatments commenced with 50% of the final salt concentration, and one week later, 100% percent of each salt level was applied. Plants were harvested after an additional week.
Shoot dry weights were obtained by drying samples at 75°C for 3 days. For root elongation assays, root length was measured every 24 h for 4 days, and root relative growth rate was calculated for each consecutive day according to Hunt (1990). For oxidative stress experiments, root elongation was calculated using the formula (L4-L0)/L4 where L0 and L4 are root lengths at days 1 or 4, respectively, after transfer to MV plates. For evaluating total leaf area, leaves were detached, placed between two transparency films (Graphic Vision Media), and scanned. Leaf area was calculated with ImageJ 1.43 software2. Anthocyanin content was analyzed according to Kant et al. (2006). Na+ and K+ ions were extracted as described by Kant et al. (2006) and ion contents determined by flame photometry (Model 410 Flame photometer, Sherwood Scientific, Ltd, UK). Chlorophyll and carotenoids were extracted in 100% methanol at a 1:10 ratio of tissue to solvent, kept overnight at 4°C in the dark, and measured according to Lichtenthaler (1987). Chlorophyll fluorescence was measured with a Mini-PAM device (Walz, Heinz Walz GmbH, Effeltrich, Germany) applying a rapid light curve (White and Critchley, 1999). Non-photochemical quenching (NPQ) and PSII ETR were calculated according to Bilger and Björkman (1990) and Krall and Edwards (1992), respectively.
Chromosome number analysis was performed on young shoot tip meristem protoplasts using a drop-spread technique followed by DAPI staining and bright-field microscopy according to Anamthawat-Jonsson (2003). For flow cytometry analysis of 2C DNA content, nuclear suspensions were prepared by chopping 60–100 mg of young shoot tip tissue in 1 ml of ice-cold nuclei isolation buffer (NIB; Dolezel et al., 1989) with the addition of 5% polyvinylpirrolidone PVP-40. After addition of a further 2 ml NIB, samples were shaken for 1 h followed by filtration through 50 μm nylon mesh and centrifugation at 1,900 rpm for 8 min. The pellet containing the nuclei was resuspended in 1 ml ice-cold NIB supplemented with 50 μg m-1 of DNase-free RNase A and 2 μg ml-1 propidium iodide (Sigma-Aldrich Chemical, Co., USA), vortexed gently for a few seconds and then incubated on ice for 10 min. Samples were filtered through 35 μm mesh and run on a Becton Dickinson FACSVantage SE equipped with a 640-nm dichroic long-pass filter, a 585/42-nm band-pass filter, and an air-cooled argon-ion laser tuned to 15 mW and operating at 488 nm. For each sample, 10,000 nuclei were recorded to calculate the mean position of the G1 peak in the histogram. The ratio of the relative fluorescence from the G0/G1 peak positions for each sample was compared against the internal standard Raphanus sativus cv. Saxa as a genome size reference (2C = 1.11 pg of DNA). Cytometer gain was adjusted so that the G0/G1 peak of R. sativus was positioned on channel 1500.
2C DNA content was calculated as follows:
Sample 2C = (mean position of the G1 sample peak/mean position of the G1 reference peak) × reference 2C DNA.
Plants were grown in soil and subjected to salt stress as described above. The fifth and sixth leaves of Arabidopsis and E. salsugineum plants, and the third and fourth leaves of A. hierochuntica were harvested, pooled, snap-frozen in liquid nitrogen and stored at -80°C. Four biological replicates were analyzed for each treatment and two independent experiments were carried out. Metabolite extraction was performed according to Lisec et al. (2006) with minor modifications (Kazachkova et al., 2013).
Derivatization was performed according to Lisec et al. (2006) while separation, chromatogram evaluation and metabolite identification/annotation were performed according to Kazachkova et al. (2013). Metabolite relative abundance was determined by normalizing the intensity of the peak of each metabolite to the ribitol standard, yielding ‘response’ values. These values were normalized using log10 transformation and analyzed statistically using MultiExperiment Viewer Version 3.1 software (Saeed et al., 2003). Two-way ANOVA tests were performed between all species and paired species. To pinpoint metabolites that were significantly affected by the salt treatment within each species (Figure 8), a one-way ANOVA (Supplementary Table S3) followed by a Dunnett test was performed. For principal component analysis (PCA), log10 transformed response data were used while hierarchical clustering was performed upon linear response values divided by the median abundance of each metabolite.
In the wild, A. hierochuntica plants exhibit great variation in developmental speed and body size ranging from between a few mm to 15–20 cm in height, depending upon the availability of soil moisture. Figure 1 shows various stages of development of A. hierochuntica under our laboratory conditions. In their early stages of growth, plants are very uniform producing two cotyledons followed by two opposite pairs of obovate, densely hirsute true leaves (Figures 1A–C). Bifurcation of the shoot begins with the third and fourth leaves separating into individual branches. An axillary inflorescence is produced at the branch point and forms a dense cluster of sub-sessile, white flowers with four petals (Figures 1D,G,H). Repeated bifurcation proceeds between subsequent pairs of more lanceolate, toothed, hirsute leaves, resulting in a multi-branched, sympodial shoot structure that can vary greatly in overall three-dimensional architecture (Figures 1E,F). Fruits are hairy, two-winged siliculae each containing four seeds (Figures 1I–K).
FIGURE 1. Growth and development of Anastatica hierochuntica. A. hierochuntica plants were raised in the growth room (see Materials and Methods) and photographed after transplantation to soil at the following times: (A) 4 days; (B) 10 days; (C) 19 days; (D) 26 days; (E) 31 days; (F) 4 months; (G) axillary inflorescence at branch point after 26 days; (H) axillary inflorescence with developing siliculae after 31 days; (I) siliculae on 4 month-old plant; (J) dry silicula; (K) A. hierochuntica seeds; (L) Arabidopsis seeds for comparison. Scale bar is 5 mm.
It has been reported using acid carmine staining that the A. hierochuntica genome is organized into 11 (haploid) chromosomes (Lifante et al., 1992). To confirm this finding, we visualized A. hierochuntica chromosomes using a protoplast-dropping protocol (Anamthawat-Jonsson, 2003). Analysis of 4′, 6-diamidino-2-phenylindole (DAPI) staining clearly showed the presence of 22 chromosomes (diploid) (Figure 2A). Flow cytometry revealed that the A. hierochuntica genome is approximately 4.5-fold larger than the Arabidopsis genome (Figures 2B,C). To validate our results, we also performed flow cytometry with E. salsugineum and showed that the genome of this species is approximately twice the size of the Arabidopsis genome as previously reported by Inan et al. (2004). Thus, A. hierochuntica has a genome size of roughly 607 Mbp.
FIGURE 2. Anastatica hierochuntica chromosome number (diploid) and DNA content. (A) DAPI-stained spread of A. hierochuntica chromosomes (2n = 22) in young shoot tip meristems. (B) Flow cytometry analysis of 2C DNA content in Arabidopsis thaliana, A. hierochuntica and Eutrema salsugineum. (C) DNA content of the three Brassicaceae species derived from (B). Data are mean (n = 4) ± SD. The ratio of the relative fluorescence from the G0/G1 peak positions for each sample was compared against the internal standard Raphanus sativus cv. Saxa as a genome size reference (2C = 1.11 pg of DNA). Cytometer gain was adjusted so that the G0/G1 peak of R. sativus was positioned on channel 1500.
Anastatica hierochuntica plants can experience daily temperatures above 40°C. Furthermore, the Negev desert is characterized by patchy soil nitrogen pools (Zaady, 2005). For instance, we measured soil NO3- levels ranging from 0.4 to 4 mM (data not shown). We therefore tested whether A. hierochuntica displays greater tolerance to heat stress and low NO3- levels in the growth medium, than Arabidopsis. In vitro-grown seedlings (two-cotyledon stage) of both species survived 1 h of heat stress (45°C) with an approximate 25% reduction in fresh weight (FW) for both species but no effect on chlorophyll or carotenoid content (Figures 3A–D; Supplementary Figure S2). Two hours of heat stress severely affected Arabidopsis seedlings leaving them pale or bleached (Figure 3A). This effect was reflected in a 67% reduction in FW, and a 45 and 54% reduction in chlorophyll and carotenoid content, respectively, compared to control seedlings. On the other hand, there was no further reduction in the FW of A. hierochuntica seedlings compared to 1 h heat stress and no effect on chlorophyll and carotenoid levels compared to the control. Heat stress of 3 and 4 h caused severe stress to Arabidopsis seedlings as manifest by no further growth of seedlings and almost complete bleaching of cotyledons. In contrast, A. hierochuntica displayed considerably greater heat tolerance than Arabidopsis after 3 and 4 h of heat stress. For instance, 4 h heat stress, caused a 50% drop in A. hierochuntica FW whereas Arabidopsis exhibited a 73% FW reduction. Furthermore, A. hierochuntica seedlings did not become bleached but maintained chlorophyll levels at approximately 65% of control levels with no effect of heat stress on carotenoid content.
FIGURE 3. Effect of heat shock (A–D) and low KNO3- concentrations (E–G) on growth parameters and pigment contents of Arabidopsis (At) and A. hierochuntica (Ah). (A) Picture of seedlings grown on MS medium, 48 h after exposure to 0, 1, 2, 3 or 4 h heat shock (45°C). (B) Shoot FW. (C) Total chlorophyll. (D) Total carotenoids. (E) Picture of seedlings grown for 6 days on MS medium supplemented with the indicated concentrations of KNO3-. (F) Plant FW. (G) Total anthocyanin content. Data are mean of three and four independent experiments for low NO3- and heat, respectively ± SD. Each independent experiment comprised four replicates containing ca. 10–15 seedlings. Bars with different letters indicate a significant difference at P < 0.05 (Tukey HSD test). FW, fresh weight.
Anastatica hierochuntica also displayed tolerance to low N stress compared to Arabidopsis (Figure 3E). Arabidopsis showed a progressive, sharp reduction in FW as NO3- levels were reduced in nutrient agar, exhibiting a 75% reduction in FW at 0.05 mM NO3- (Figure 3F; Supplementary Figure S3). In contrast, A. hierochuntica showed a much more moderate drop in FW as NO3- levels were reduced, and FW only fell by 25% at 0.05 mM NO3-. The highly stressed condition of the Arabidopsis seedlings at low NO3- levels was manifest by the production of progressively higher amounts of anthocyanins as NO3- levels dropped (Figure 3G). On the other hand, no significant differences in A. hierochuntica anthocyanin content compared to control could be discerned at any NO3- level.
Because the Negev desert possesses islands of high salinity and A. hierochuntica is also found growing in the Dead Sea valley, we tested whether A. hierochuntica exhibits greater tolerance to salt stress than Arabidopsis. A much greater reduction in Arabidopsis growth was discerned as salt concentration increased than was observed in A. hierochuntica (Figures 4A–C; Supplementary Figure S4). For instance, at 100 and 200 mM NaCl, Arabidopsis displayed a 55 and 77% reduction in FW, respectively, while A. hierochuntica FW was only reduced by 30 and 48% at the respective iso-saline concentration (Figure 4A). Similarly, dry weight was affected to a lesser extent in A. hierochuntica than Arabidopsis at 100 and 200 mM NaCl (Figure 4B). Arabidopsis leaf area was also affected to a greater extent by salt stress than A. hierochuntica. For example, Arabidopsis exhibited a 74% reduction in leaf area at 200 mM NaCl whereas A. hierochuntica leaf area only dropped by 49% (Figure 4C). Furthermore, in an in vitro plate root elongation assay, Arabidopsis seedlings showed a clear and drastic salt-mediated dose-response reduction in root relative growth rate such that at 200 mM NaCl, root elongation had virtually ceased (Figure 4D). On the other hand, 100 mM NaCl only had a mild effect on A. hierochuntica root relative growth rate while root elongation continued even at 200 mM NaCl (Figure 4E).
FIGURE 4. The effect of salt stress on growth parameters of A. hierochuntica and Arabidopsis. (A–C) Plants grown on soil were exposed to incremental increases of NaCl concentration, and were harvested 1 week after the final NaCl concentration was reached. (A) Shoot FW. (B) Shoot DW. (C) Rosette leaf area. (D,E) Seedlings were grown on MS medium in vertical plates without NaCl and then transferred to fresh vertical MS plates supplemented with the indicated NaCl concentrations. (D) Arabidopsis root relative growth rate (RGR). (E) A. hierochuntica root RGR. Data are expressed as a percentage of control (0 mM) RGR on day 1 after transfer to salt stress. Data are mean of three to four experiments ± SD. Each independent experiment comprised four replicate plates with 10 seedlings per plate. For both soil and plate experiments, bars or time points with different letters indicate a significant difference at P < 0.05 (Tukey HSD test). FW, fresh weight; DW, dry weight.
One feature of natural salt tolerance is the ability of plants to tightly control Na+ accumulation (e.g., Kant et al., 2006). Under control conditions, A. hierochuntica possessed about 4.5 times as much Na+ in the shoots as Arabidopsis, similar to the threefold higher amount of E. salsugineum shoot Na+ compared to Arabidopsis (Figure 5A; Kant et al., 2006). Both species accumulated increasing amounts of Na+ as salt concentrations increased, but Arabidopsis accumulated Na+ to a much greater extent than A. hierochuntica (Figure 5B). Arabidopsis and A. hierochuntica exhibited comparable shoot K+ levels under control conditions and a similar reduction in K+ content in response to salt stress (Figure 5C). Thus, under control conditions, A. hierochuntica had a higher Na+/K+ ratio than Arabidopsis (Figure 5D) but as soil salt concentrations increased, Na+/K+ ratios rose to a much lower extent in A. hierochuntica (Figures 5D,E). Both species exhibited similar FW/DW ratios at all salt concentrations (Figure 5F) suggesting that differences in ion contents was not due to variations in tissue water content. Taken together, our ion analysis data suggest that A. hierochuntica is able to tightly control Na+ accumulation.
FIGURE 5. Effect of NaCl on Na+ and K+ contents (A–D) and photochemistry parameters (E,F) of Arabidopsis and A. hierochuntica shoots. Plants grown on soil were exposed to incremental increases of NaCl concentration. The plants were harvested 1 week after the final NaCl concentration was reached. (A) Absolute Na+ content. (B) Relative changes in Na+ content compared to control treatment. (C) K+ content. (D) Na+/K+ ratio. (E) Relative changes in Na+/K+ ratio compared to control treatment. (F) Shoot FW/DW ratio. (G) Non-photochemical quenching (NPQ). (H) PSII electron transport rates (ETR). Values of NPQ and PSII ETR are presented from plants exposed to a light intensity of 1500 Photosynthetic Photon Flux Density (PPFD). Data represent mean (n = 4) ± SD. Each replicate comprised pooled data from six individual plants. The data are representative of similar results from two independent experiments. Bars with different letters indicate a significant difference at P < 0.05 (Tukey HSD test). FW, fresh weight; DW, dry weight.
We recently reported that A. hierochuntica under control conditions exhibits lower NPQ, a mechanism for dissipating excess light energy, than Arabidopsis, and a relatively higher photosystem II electron transport rate (PSII ETR) (Eppel et al., 2014). In the present study, A. hierochuntica exhibited an approximately 50% lower NPQ than Arabidopsis under control conditions (Figure 5G), and while salt stress had no effect on Arabidopsis NPQ, it caused a further 50% fall in A. hierochuntica NPQ. Under control conditions, PSII ETR was about 55% higher in A. hierochuntica compared to Arabidopsis (Figure 5H). Salt stress caused a fall in PSII ETR in both species but A. hierochuntica still maintained a 45% higher PSII ETR in comparison to Arabidopsis. Thus, the NPQ and PSII ETR data suggest that A. hierochuntica photochemistry displays considerable resilience to salt stress.
Eutrema salsugineum exhibits extreme salt tolerance (Kant et al., 2006; Kazachkova et al., 2013), which presented us with the opportunity of comparing three related species originating from very different environments, and differing in their salt tolerance: the salt-sensitive glycophyte Arabidopsis (temperate Columbia, MT, USA), and the two extremophytes – moderately salt-tolerant A. hierochuntica (hot, dry Negev desert), and highly tolerant E. salsugineum (cold, salty coastal areas of the Shandong province, China).
Arabidopsis, E. salsugineum, and A. hierochuntica plants grown in soil were exposed to increasing concentrations of NaCl (E. salsugineum was not exposed to 50 mM NaCl due to the mild effect of this concentration; Kazachkova et al., 2013), and the relative abundance of primary carbon and nitrogen metabolites was determined for leaf tissue. To gain a global view of the differences between the plant species and across treatments, PCA was employed. Inspection of the first two principle components, which account for 68% of the total variance within the data set, allowed classification of samples by species and treatments (Figure 6). The first principal component accounting for 48.2% of total variance clearly separated the metabolism of Arabidopsis from its two extremophyte relatives (Figure 6A). Among the metabolites most affecting the separation between species (in decreasing order of absolute eigenvector value and confirmed by two-way ANOVA) were raffinose, malate, galactinol, fumarate, phosphoric acid, and glycerate (Supplementary Tables S1 and S2). Overall, the two-way ANOVA test for all three species revealed that 25 out of 33 metabolites were significantly different between the species (Supplementary Table S2). Among these 25 metabolites, 22 metabolites also displayed a significant species × salt-treatment interaction.
FIGURE 6. Principal component analyses (PCA) and hierarchical clustering of metabolic profiles of Arabidopsis (At), A. hierochuntica (Ah) and E. salsugineum (Es) under control and salt stress conditions. For PCA, metabolite relative abundance was first normalized using log10 transformation and then analyzed statistically using MultiExperiment Viewer Version 3.1 software (Saeed et al., 2003). The variance explained by each component is indicated in brackets. (A) PCA comparing all three species. (B) PCA comparing E. salsugineum and A. hierochuntica. (C) Hierarchical clustering (HCA) of all three species. For HCA, averages of absolute values (n = 4) for each metabolite normalized by the ribitol standard were divided by the median normalized metabolite abundance and are presented as a heat map. For both analyses, data are representative of similar results from two independent experiments.
The second principal component accounting for 19.8% of total variance discriminated samples according to salt treatment. Among the metabolites most affecting separation between samples (in descending order of absolute eigenvector value and confirmed by two-way ANOVA) were proline, malate, raffinose, serine, fumarate, and myo-inositol. Overall, 20 metabolites were found to be significantly different between salt treatments (Supplementary Table S2). Control and salt-treated Arabidopsis sample groups were substantially spread out across the second component, whereas E. salsuginem and A. hierochuntica sample groups were more condensed.
The complete separation of the metabolic profiles of Arabidopsis and the two extremophytes, and the partially overlapping metabolic response of E. salsugineum and A. hierochuntica suggested both common and species-specific responses of the extremophytes to salt stress (Figure 6A). This notion was supported by hierarchical clustering analysis (HCA) that placed the two extremophytes on a separate clade from Arabidopsis but separated E. salsugineum and A. hierochuntica on two sub-clades (Figure 6C). PCA of the two extremophytes alone clearly illustrated the species-specific metabolic response to salt stress (Figure 6B). The metabolites that primarily affected the separation of the extremophytes (in decreasing order of absolute eigenvector value and confirmed by two-way ANOVA test) were: proline, citrate, aspartate, raffinose, threonic acid, and pyroglutamic acid (Supplementary Tables S1 and S2).
Examination of individual metabolites under control conditions revealed an overall comparable metabolic phenotype between the two extremophytes with most of the metabolites exhibiting a significantly higher abundance in A. hierochuntica and E. salsugineum compared to Arabidopsis (Figure 7). Among the metabolites exhibiting differences between Arabidopsis and the two extremophytes under control conditions were: (i) the TCA cycle intermediates, citrate and malate that were significantly higher in the extremophytes, and fumarate which was significantly higher in Arabidopsis; (ii) the sugars, glucose and fructose; (iii) the hexose phosphates, fructose-6-phosphate and glucose-6-phosphate; (iv) phosphoric acid; (v) the antioxidants, ascorbate and dehydroascorbate (Foyer and Noctor, 2011). The levels of the metabolites indicated in (ii) to (v) were all higher in the extremophytes. In addition, the osmoprotectant proline (Smirnoff and Cumbes, 1989) was higher in the extremophyte plants under control conditions while the osmoprotectants myo-inositol (also a precursor of the osmoprotectant galactinol; Bohnert et al., 2006) and galactinol (also a precursor of the osmoprotectant raffinose; Taji et al., 2002; Nishizawa et al., 2008) were higher in Arabidopsis. Interestingly, the majority of metabolites displayed significantly lower levels in A. hierochuntica compared to E. salsugineum.
FIGURE 7. Comparison of metabolites between Arabidopsis, E. salsugineum, and A. hierochuntica under control conditions. Data are representative of similar results from two independent experiments. Metabolites that significantly differ between species are marked with asterisks (Student’s t-test, P < 0.05, n = 4). Black and gray bars indicate metabolites that are more abundant in the numerators or denominators, respectively. THZ, 4-methyl-5-hydroxyethyl-thiazole.
Inspection of the levels of metabolites in response to salt stress revealed only a few common metabolite responses. All three species exhibited increased levels of the osmoprotectants myo-inositol and proline in response to salt stress (Figure 8). Notably, E. salsugineum exhibited greater proline content than Arabidopsis and A. hierochuntica at control and 100 mM NaCl. High proline levels are a well-characterized feature of soil-grown, well-fertilized E. salsugineum (Kant et al., 2006; Guevara et al., 2012; Kazachkova et al., 2013). In addition, glycine levels were reduced in response to stress in all three species although the actual level of glycine was highest in Arabidopsis at all treatment levels compared to the extremophytes.
FIGURE 8. Comparison of Arabidopsis (white bars), E. salsugineum (black bars), and A. hierochuntica (gray bars) metabolic responses to salt stress. Data are mean (n = 4) ± SD, and are representative of two independent experiments. Asterisks represent significant difference (P < 0.05, Dunnett test) between Arabidopsis, E. salsugineum or A. hierochuntica and their respective controls. GPGro, glycerophosphoglycerol.
In contrast to the few common metabolic responses between the three species, a considerable number of metabolites exhibited an overall common response in both extremophytes that was different to that observed in Arabidopsis. For instance, among amino acids, the level of alanine in Arabidopsis gradually declined in response to salt, while remaining constitutively high in the extremophytes and even rising slightly in A. hierochuntica at 200 mM NaCl. Aspartate was barely detectable in Arabidopsis, yet increased substantially with salt concentration in both extremophyte species, but particularly in E. salsugineum. In contrast, the levels of threonine increased dramatically in Arabidopsis, while remaining relatively lower in the extremophytes, with a small but significant rise in A. hierochuntica at higher salt levels. Serine and glycerate, which are intermediates of the photorespiratory cycle, also displayed differences in accumulation between the extremophytes and Arabidopsis. Serine content increased in response to salt in both Arabidopsis and A. hierochuntica but accumulated to considerably higher levels in Arabidopsis at the highest salt concentration. On the other hand, serine only displayed a slight salt-mediated decrease in E. salsugineum. Overall glycerate levels were much lower in Arabidopsis than in the extremophytes. Furthermore, while glycerate exhibited no response to salt in Arabidopsis, it was maintained at a constantly higher level in A. hierochuntica and displayed a salt-mediated increase in E. salsugineum.
The levels of the TCA cycle intermediates, citrate and malate, were substantially higher in the extremophytes compared to Arabidopsis. However, whereas in E. salsugineum both citrate and malate levels increased significantly in response to salt, citrate levels in A. hierochuntica increased significantly while malate remained at a constantly higher level than in Arabidopsis. Fumarate content, on the other hand, was substantially higher in Arabidopsis, decreasing in response to salt stress, but remaining constantly low in the extremophytes. The levels of other carboxylic acids, such as ascorbate and dehydroascorbate, were constitutively higher in the extremophytes than in Arabidopsis.
Differences in sugar contents between Arabidopsis and the extremophytes were also observed. The most striking differences were in the levels of the osmoprotectants galactinol and raffinose (Taji et al., 2002; Nishizawa et al., 2008). Both increased dramatically in Arabidopsis, but were induced to a much lower extent in the extremophytes. In addition, fructose-6-phosphate and glucose-6-phosphate accumulated to a greater extent in the extremophytes, being maintained at overall constant levels in A. hierochuntica, while significantly reduced in E. salsugineum in response to salt.
We also discerned species-specific responses that differentiated between the extremophytes. For example, the level of serine in E. salsugineum was significantly reduced by salt stress whereas in A. hierochuntica serine content increased significantly. Pyroglutamate (possibly part of the glutathione recycling pathway, an osmoprotectant and a Glu reservoir (Ohkama-Ohtsu et al., 2008; Kumar and Bachhawat, 2012; Schreiber et al., 2012), fructose, and glycerophosphoglycerol all exhibited constitutively higher levels in E. salsugineum compared to A. hierochuntica.
The constitutively higher levels of the antioxidant compounds ascorbate and dehydroascorbate in A. hierochuntica (and E. salsugineum) compared to Arabidopsis (Figures 7 and 8) suggested that A. hierochuntica possesses a constitutively active antioxidant system. Comparison of the response of Arabidopsis and A. hierochuntica seedlings to ROS-forming methyl viologen (MV) (Babbs et al., 1989; Fujii et al., 1990), demonstrated that A. hierochuntica seedlings exhibited remarkable tolerance to increasing concentrations of MV compared to Arabidopsis (Figure 9A). A. hierochuntica shoot FW and root elongation decreased to a far lesser extent than Arabidopsis as MV concentration rose. Furthermore, A. hierochuntica maintained total chlorophyll levels in response to extreme MV concentrations (12 μM) whereas chlorophyll levels were reduced in Arabidopsis, which exhibited signs of bleaching (Figures 9A–D; Supplementary Figure S5). The severe stress symptoms observed in Arabidopsis were also reflected in the high accumulation of anthocyanins in response to MV whereas only low levels of anthocyanins were observed in A. hierochuntica (Figure 9E). These data suggest that A. hierochuntica has a highly active antioxidant system.
FIGURE 9. The effect of oxidative stress on growth parameters and pigment contents of Arabidopsis and A. hierochuntica. Seedlings grown on vertical MS plates were transferred to 0, 0.5, 1, 4, 8, or 12 μM Methyl viologen for 5 days. (A) Phenotypes of seedlings after 5 days on 0 and 12 μM Methyl viologen. (B) Shoot FW. (C) Root elongation. (D) Total chlorophyll content. (E) Total anthocyanin content. Data are mean (n = 4) ± SD. Each replicate plate contained ca. 10 (Arabidopsis) or ca. 6 (A. hierochuntica) seedlings. Data are representative of two independent experiments. Bars with different letters indicate a significant difference at P < 0.05 (Tukey HSD test). FW, fresh weight.
The Brassicaceae family comprises over 3,600 species including many important crops as well as the premier model plant system A. thaliana (Franzke et al., 2011; Al-Shehbaz, 2012). It contains 301 genera classified into 49 monophyletic tribes. The family can be split into two major groups: the Aethionema group and the core group (Franzke et al., 2011), the latter of which can be divided into three major lineages (lineages I, II, and III).
In recent years, a number of genomic resources have been generated for the Brassicaceae thereby providing an impressive platform for evolutionary and comparative biology studies. At least 36 genomes have been sequenced or are being sequenced (Koenig and Weigel, 2015) including 13 species from lineage I (e.g., A. thaliana, Arabidopsis lyrata, Capsella rubella), 17 species from lineage II or extended lineage II (e.g., Arabis alpina, Brassica napus, E. salsugineum, S. parvula) but only three species from lineage III (Diptychocarpus strictus, Euclidium syriacum, Malcolmia maritima) (Franzke et al., 2011; Kiefer et al., 2014; Huang et al., 2015). Moreover, of the completed genomes, none are from lineage III species. This rich collection of genomes affords a means of understanding characteristics such as developmental and life history traits that may be missing in A. thaliana. In particular, extremophytes of the Brassicaceae family that thrive in some of the most extreme environments offer excellent systems for comprehending the molecular mechanisms underlying adaptation to harsh environments.
The extremophyte A. hierochuntica from the tribe Anastaticeae belongs to the underrepresented lineage III species (Couvreur et al., 2010; Kiefer et al., 2014; Huang et al., 2015), for which there is a dearth of physiological and molecular knowledge. Moreover, A. hierochuntica presents an opportunity for comparison of a desert annual with Brassicaceae extremophytes such as E. salsugineum that will facilitate our understanding of how plants have adapted to very different extreme habitats.
In the current study, we demonstrated that A. hierochuntica is tolerant to several stresses associated with its desert habitat, namely high temperatures, low soil N, and salinity (Figures 3 and 4). Both heat tolerance and low N tolerance are important traits for which A. hierochuntica might be a source of novel tolerance determinants. Heat stress, especially when combined with drought, can lead to huge losses in crop production (Mittler, 2006; Mittler and Blumwald, 2010; Lesk et al., 2016) particularly because cereals are most sensitive to these stresses during the grain-filling phase (Barnabas et al., 2008). Indeed, a recent report identified heat tolerance in wheat at the reproductive stage as being a key trait for increasing yields under projected climate change (Stratonovitch and Semenov, 2015). Although detailed reports on extremophytes able to withstand extremely high temperatures are sparse, some work has begun (Emad El-Deen, 2005; Lawson et al., 2014; Yates et al., 2014; Gallas and Waters, 2015). Rhazya stricta in particular, can maintain fully functional photosynthesis in the field at leaf temperatures as high as 43°C along with light intensities >1000 μmol photons m-1 s-1 (Lawson et al., 2014).
Nitrogen use efficiency (NUE) is another trait which has been the focus of much research and breeding efforts (McAllister et al., 2012; Han et al., 2015). Crop plants only exploit about 30 to 40% of applied N (Raun and Johnson, 1999), and the remaining N is lost by leaching, denitrification, volatilization, soil erosion, and microbial consumption thereby increasing both environmental N pollution and production costs (Good et al., 2004). Because traditional breeding for improved NUE has hit a plateau, the use of low N-tolerant extremophytes has been suggested as one approach to identify new alleles and genes (Kant et al., 2011). Indeed, E. salsugineum is more tolerant to N-limiting conditions than Arabidopsis, and possesses higher N content, total amino acids and total soluble protein at low soil N levels (Kant et al., 2008). The higher NUE of E. salsugineum can be partly attributed to constitutively higher NO3- uptake under low N conditions, as well as differential expression of genes encoding NO3- assimilation and transporter genes.
Anastatica hierochuntica exhibited several physiological features of salt tolerance that are similar to those observed in E. salsugineum (Inan et al., 2004; Kant et al., 2006). Firstly, under control conditions, A. hierochuntica possesses higher shoot Na+ levels than Arabidopsis (Figure 5). In E. salsugineum, higher shoot Na+ content probably aids in maintaining a constitutively more negative leaf osmotic potential than Arabidopsis (Inan et al., 2004). Secondly, under saline conditions A. hierochuntica exhibits tight control of Na+ accumulation compared to Arabidopsis (Figures 5A–D) again similar to E. salsugineum (Kant et al., 2006). A significantly reduced Na+ inward current and smaller Na+ influx into E. salsugineum roots compared to Arabidopsis (Volkov and Amtmann, 2006; Wang et al., 2006), as well as differential expression of a gene encoding the Na+/H+ antiporter, SOS1 (Kant et al., 2006), likely contribute to stringent control of Na+ uptake in the extremophyte.
Thirdly, A. hierochuntica displays a higher PSII electron transport and a lower NPQ than Arabidopsis under control and saline conditions suggesting that a greater amount of light energy is used for productive purposes in A. hierochuntica than in Arabidopsis (Figures 5G,H). E. salsugineum is also able to maintain a lower NPQ than Arabidopsis under saline conditions while PSII electron transport actually increases in response to salt (Stepien and Johnson, 2009).
Anastatica hierochuntica displays constitutively high levels of antioxidant compounds (Figures 7 and 8) and striking tolerance to ROS-generating MV (Figure 9) suggesting that A. hierochuntica possesses a highly active antioxidant system, which is a common feature of extremophytes. In E. salsugineum and S. parvula for example, several enzymes involved in ROS scavenging are highly active, and antioxidant metabolites such as thioredoxin, ascorbate, and dehydroascorbate exhibit constitutively higher levels than in Arabidopsis (M’rah et al., 2007; Kazachkova et al., 2013; Uzilday et al., 2014). Higher expression of E. salsugineum genes encoding components of the antioxidant machinery under both control and salt stress conditions might underlie the higher enzyme activities and antioxidant levels (Taji et al., 2004; Gong et al., 2005).
Active antioxidant mechanisms are not only important for desert extremophytes or halophytes but are also crucial for other extremophytes. For instance, arctic plants such as Deschampsia antarctica, have to sustain photochemistry under a combination of low temperatures and high light. D. antarctica possesses constitutively higher superoxide dismutase (SOD) activity compared to other Poaceae, expresses a hydrogen peroxide-resistant MnSOD isozyme during cold acclimation, and produces a number of inducible UV-B protective compounds (Xiong and Day, 2001; Perez-Torres et al., 2004; Ruhland et al., 2005).
In addition to antioxidant mechanisms, energy dissipating processes can also prevent generation of damaging ROS. For instance, in extremophytes such as A. hierochuntica and the alpine plant, Ranunculus glacialis, photorespiration plays a role in energy dissipation (Streb et al., 2005; Eppel et al., 2014). However, this does not appear to be the case in E. salsugineum. Rather, there is a stress-mediated rise in the protein levels of plastid terminal oxidase that could function as an alternative electron sink and prevent generation of ROS (Stepien and Johnson, 2009). Interestingly, the amount of PTOX in R. glacialis leaves exceeds the amount found in all other plants species examined, and even when electron flow to assimilation, photorespiration and the Mehler reaction are blocked, R. glacialis leaves under high light intensity can maintain electron flow (Streb et al., 2005; Laureau et al., 2013).
Thus, for extremophytes encountering a combination of severe stresses such as A. hierochuntica (high light intensity, high temperatures, low soil nutrient status, saline soils), highly active antioxidant systems often coupled with excess energy dissipation mechanisms appear crucial for survival in extreme habitats.
Our metabolic profiling data suggested that the extremophytes possess both common and species-specific stress-mediated changes in metabolism that are different from Arabidopsis (Figures 6–8). Furthermore, the PCA analysis suggested that the different levels of salt stress cause much larger changes in Arabidopsis than in the extremophytes (Figure 6) indicating that A. hierochuntica and E. salsugineum may be primed for stress in agreement with other reports on E. salsugineum (e.g., Kant et al., 2006; Kazachkova et al., 2013; Vera-Estrella et al., 2014). Further support for the notion that the extremophytes are primed for stress could be seen in the analysis of metabolite levels under control conditions (Figure 7). Both extremophytes showed accumulation of antioxidants, sugars and TCA cycle intermediates, which have been previously shown to be important for plant responses to various stresses, including salt and drought (Smirnoff, 1996; Urano et al., 2009; Lugan et al., 2010; Obata and Fernie, 2012). Not all extremophytes, however, are primed for stress. For example, the salt tolerant extremophyte legume Lotus creticus exhibits no such phenomenon (Sanchez et al., 2011).
Striking differences were observed between the extremophytes and Arabidopsis in the abundance of specific TCA cycle intermediates. Both extremophytes exhibited high levels of malate and citrate but low levels of fumarate whereas Arabidopsis showed the opposite pattern (Figures 7 and 8). This metabolic signature appears to be an important feature of extremophyte Brassicaceae under control, salt and low-N conditions and irrespective of growth platforms (Kant et al., 2008; Kazachkova et al., 2013). We previously speculated that a large increase in the malate pool and low fumarate levels could reflect the generation of oxaloacetate to provide carbon skeletons for enhanced amino acid synthesis in E salsugineum (Kazachkova et al., 2013). Oxaloacetate can be further metabolized to aspartate (Sweetlove et al., 2010), and indeed we observed higher control and salt-induced levels of aspartate in the extremophytes compared to Arabidopsis. One use of higher aspartate levels might be to supply amino groups to the photorespiratory cycle via glycine (Novitskaya et al., 2002). Alanine can also contribute amino groups to glycine during photorespiration (Liepman and Olsen, 2001; Novitskaya et al., 2002), and the extremophytes were able to maintain alanine levels under control and salt stress conditions whereas alanine content decreased in Arabidopsis in response to salt. Consistent with the idea that the extremophytes can sustain metabolic flux through the photorespiratory cycle, the glycine to serine ratio was always lower in the extremophytes than in Arabidopsis. A high glycine to serine ratio often correlates with the oxygenation of rubisco in the short term stages after induction of photorespiratory conditions (Novitskaya et al., 2002). However, increased flux through the photorespiratory pathway causes a reduction in the glycine to serine ratio (Timm et al., 2012). Further support for a higher photorespiratory flux in the extremophytes can be derived from the higher glycerate levels in A. heirochuntica and E. salsugineum under control and salt stress conditions compared to Arabidopsis. Glycerate is the product of the penultimate reaction in the photorespiratory pathway and is converted to 3-phosphoglycerate, which is fed back into the Calvin cycle (Hagemann and Bauwe, 2016). As mentioned above, photorespiration plays an important role in excess energy dissipation in A. hierochuntica (Eppel et al., 2014).
Another reason for low levels of malate in Arabidopsis compared to the extremophytes might be due to the likely greater salt-mediated inhibition of photosynthesis in Arabidopsis (Stepien and Johnson, 2009). This could lead to reduced carbon flux through the glycolytic pathway and thus a need for the conversion of malate to pyruvate by malic enzyme to maintain the function of the TCA cycle (Casati et al., 1999). On the other hand, both A. hierochuntica and E. salsugineum exhibit a relatively higher photosynthetic rate and lower NPQ than A. thaliana and other plant species, under control and salt conditions, and under various CO2 concentrations and light intensities (Figures 5G,H; Stepien and Johnson, 2009; Eppel et al., 2014). Indeed, the higher overall levels of glucose, fructose, glucose 6-phosphate, and fructose 6-phosphate in the extremophytes may indicate a higher glycolysis flux either from photosynthesis or starch consumption, which may maintain pyruvate entry into the TCA cycle.
Raffinose and its precursor galactinol are thought to be osmoprotectants and antioxidants (Taji et al., 2002; Nishizawa et al., 2008; Farrant et al., 2009). We observed a large rise in galactinol and raffinose content in Arabidopsis in response to salt whereas levels of these metabolites remained low in the extremophytes (Figure 8). It is remarkable that extremophytes from two greatly differing habitats have both evolved to avoid accumulation of high levels of raffinose as a stress tolerance component similar to the desiccation-tolerant spike moss Selaginella lepidophylla (Yobi et al., 2012). Although, it is unclear what advantage is gained by not accumulating raffinose, this sugar can be metabolized into sucrose and thereafter into glucose and fructose for glycolysis.
The increased accumulation of metabolites observed in the extremophytes is costly (Munns, 2002) and could contribute to the slower growth rate of E. salsugineum compared to Arabidopsis (Lugan et al., 2010). However, A. hierochuntica, where the levels of most metabolites and therefore the costs are lower compared to E. salsugineum, exhibits fast germination and seedling establishment. It is possible that the saline habitat of E. salsugineum, demands constantly high levels of compatible solutes and antioxidants. In contrast, A. hierochuntica is exposed to lower salinity levels than E. salsugineum, but experiences intense light radiation, low water and nutrient availability and temperature extremes. By accumulating specific compounds, such as antioxidants, while moderately accumulating other solutes such as sugars and amino acids, A. hierochuntica could have developed a stress-tolerance strategy with lower metabolic costs, suited to its specific habitat, allowing fast seedling establishment and quick completion of its life cycle to avoid the dry season.
GE, RS, and SB conceived and designed the study. GE and RS performed all experiments except for the chromosome count, chlorophyll fluorescence and flow cytometry, which were performed by AK, AE, and AC, respectively, under the supervision of SB, SR, and NT-Z, respectively. GE and YK, under the supervision of AF, performed the metabolic profiling experiments and data analysis. TA participated in the oxidative stress experiments. YG supervised and participated in field collections of A. hierochuntica as well as advising on growth protocols. GE and SB wrote the article and all authors read and approved the manuscript.
This research was supported by the Koshland Foundation for Support of Interdisciplinary Research in Combatting Desertification, the Goldinger Trust Jewish Fund for the Future, and the I-CORE Program of the Planning and Budgeting Committee and The Israel Science Foundation [152/11].
The authors declare that the research was conducted in the absence of any commercial or financial relationships that could be construed as a potential conflict of interest.
The authors thank Noga Sikron for excellent technical help and Albert Batushansky for statistical advice. We appreciate Prof. J. Doležel’s kind donation of R. sativus seeds. Thanks to the Gilat Hasade Services Laboratory for ion content measurements, and to María Fernanda Arroyave Martínez for the picture of Arabidopsis and A. hierochuntica growth at higher light intensities. We are most grateful to Anna Amtmann for critical reading of this manuscript.
The Supplementary Material for this article can be found online at: http://journal.frontiersin.org/article/10.3389/fpls.2016.01992/full#supplementary-material
Al-Shehbaz, I. A. (2012). A generic and tribal synopsis of the Brassicaceae (Cruciferae). Taxon 61, 931–954.
Amtmann, A. (2009). Learning from evolution: Thellungiella generates new knowledge on essential and critical components of abiotic stress tolerance in plants. Mol. Plant 2, 3–12. doi: 10.1093/mp/ssn094
Anamthawat-Jonsson, K. (2003). Preparation of chromosomes from plant leaf meristems for karyotype analysis and in situ hybridization. Methods Cell Sci. 25, 91–95. doi: 10.1007/s11022-004-5620-y
Babbs, C. F., Pham, J. A., and Coolbaugh, R. C. (1989). Lethal hydroxyl radical production in paraquat-treated plants. Plant Physiol. 90, 1267–1270. doi: 10.1104/pp.90.4.1267
Barnabas, B., Jager, K., and Feher, A. (2008). The effect of drought and heat stress on reproductive processes in cereals. Plant Cell Environ. 31, 11–38. doi: 10.1111/j.1365-3040.2007.01727.x
Bilger, W., and Björkman, O. (1990). Role of the xanthophyll cycle in photoprotection elucidated by measurements of light-induced absorbance changes, fluorescence and photosynthesis in leaves of Hedera. . Photosynth. Res. 25, 173–185. doi: 10.1007/BF00033159
Bohnert, H. J., Gong, Q., Li, P., and Ma, S. (2006). Unraveling abiotic stress tolerance mechanisms–getting genomics going. Curr. Opin. Plant Biol. 9, 180–188. doi: 10.1016/j.pbi.2006.01.003
Boyer, J. S. (1982). Plant productivity and environment. Science 218, 443–448. doi: 10.1126/science.218.4571.443
Bray, E. A., Bailey-Serres, J., and Weretilnyk, E. (2000). “Responses to abiotic stresses,” in Biochemistry and Molecular Biology of Plants, eds B. B. Buchanan, W. Gruissem, and R. L. Jones (Rockville, MD: American Society of Plant Physiologists), 1158–1203.
Bressan, R., Park, H., Orsini, F., Oh, D.-H., Dassanayake, M., Inan, G., et al. (2013). Biotechnology for mechanisms that counteract salt stress in extremophile species: a genome-based view. Plant Biotechnol. Rep. 7, 27–37. doi: 10.1007/s11816-012-0249-9
Casati, P., Drincovich, M. F., Edwards, G. E., and Andreo, C. S. (1999). Malate metabolism by NADP-malic enzyme in plant defense. Photosynth. Res. 61, 99–105. doi: 10.1023/A:1006209003096
Champigny, M. J., Sung, W. W. L., Catana, V., Salwan, R., Summers, P. S., Dudley, S. A., et al. (2013). RNA-Seq effectively monitors gene expression in Eutrema salsugineum plants growing in an extreme natural habitat and in controlled growth cabinet conditions. BMC Genomics 14:578. doi: 10.1186/1471-2164-14-578
Cheeseman, J. M. (2014). The evolution of halophytes, glycophytes and crops, and its implications for food security under saline conditions. New Phytol. 206, 557–570. doi: 10.1111/nph.13217
Couvreur, T. L. P., Franzke, A., Al-Shehbaz, I. A., Bakker, F. T., Koch, M. A., and Mummenhoff, K. (2010). Molecular phylogenetics, temporal diversification, and principles of evolution in the mustard family (Brassicaceae). Mol. Biol. Evol. 27, 55–71. doi: 10.1093/molbev/msp202
Cramer, G. R., Urano, K., Delrot, S., Pezzotti, M., and Shinozaki, K. (2011). Effects of abiotic stress on plants: a systems biology perspective. BMC Plant Biol. 11:163. doi: 10.1186/1471-2229-11-163
Dassanayake, M., Haas, J. S., Bohnert, H. J., and Cheeseman, J. M. (2009). Shedding light on an extremophile lifestyle through transcriptomics. New Phytol. 183, 764–775. doi: 10.1111/j.1469-8137.2009.02913.x
Dassanayake, M., Oh, D.-H., Hong, H., Bohnert, H. J., and Cheeseman, J. M. (2011). Transcription strength and halophytic lifestyle. Trends Plant Sci. 16, 1–3. doi: 10.1111/j.1469-8137.2009.02913.x
Dolezel, J., Binarova, P., and Lucretti, S. (1989). Analysis of nuclear DNA content in plant cells by flow cytometry. Biol. Plant. 31, 113–120. doi: 10.1007/BF02907241
Emad El-Deen, H. M. (2005). Population ecology of Rhazya stricta Decne. in western Saudi Arabia. Int. J. Agric. Biol. 7, 932–938.
Eppel, A., Shaked, R., Eshel, G., Barak, S., and Rachmilevitch, S. (2014). Low induction of non-photochemical quenching and high photochemical efficiency in the annual desert plant Anastatica hierochuntica. Physiol. Plant. 151, 544–558. doi: 10.1111/ppl.12146
Farrant, J. M., Lehner, A., Cooper, K., and Wiswedel, S. (2009). Desiccation tolerance in the vegetative tissues of the fern Mohria caffrorum is seasonally regulated. Plant J. 57, 65–79. doi: 10.1111/j.1365-313X.2008.03673.x
Flowers, T. J., Munns, R., and Colmer, T. D. (2015). Sodium chloride toxicity and the cellular basis of salt tolerance in halophytes. Ann. Bot. 115, 419–431. doi: 10.1093/aob/mcu217
Foyer, C. H., and Noctor, G. (2011). Ascorbate and glutathione: the heart of the redox hub. Plant Physiol. 155, 2–18. doi: 10.1104/pp.110.167569
Franzke, A., Lysak, M. A., Al-Shehbaz, I. A., Koch, M. A., and Mummenhoff, K. (2011). Cabbage family affairs: the evolutionary history of Brassicaceae. Trends Plant Sci. 16, 108–116. doi: 10.1016/j.tplants.2010.11.005
Friedman, J., Gunderman, N., and Ellis, M. (1978). Water response of the hygrochastic skeletons of the True Rose of Jericho (Anastatica hierochuntica L.). Oecologia 32, 289–301. doi: 10.1007/BF00345108
Friedman, J., and Stein, Z. (1980). The influence of seed dispersal mechanisms on the distribution and survival of Anastatica hierochuntica (Cruciferae) in the Negev Desert. J. Ecol. 68, 43–50. doi: 10.2307/2259242
Friedman, J., Stein, Z., and Rushkin, E. (1981). Drought tolerance of germinating seeds and young seedlings of Anastatica hierochuntica L. Oecologia 51, 400–403. doi: 10.1007/BF00540912
Fujii, T., Yokoyama, E., Inoue, K., and Sakurai, H. (1990). The sites of electron donation of photosystem I to methyl viologen. Biochim. Biophys. Acta 1015, 41–48. doi: 10.1016/0005-2728(90)90213-N
Gallas, G., and Waters, E. R. (2015). Boechera species exhibit species-specific responses to combined heat and high light stress. PLoS ONE 10:e0129041. doi: 10.1371/journal.pone.0129041
Gechev, T. S., Dinakar, C., Benina, M., Toneva, V., and Bartels, D. (2012). Molecular mechanisms of desiccation tolerance in resurrection plants. Cell. Mol. Life Sci. 69, 3175–3186. doi: 10.1007/s00018-012-1088-0
Gong, Q., Li, P., Ma, S., Rupassara, S. I., and Bohnert, H. J. (2005). Salinity stress adaptation competence in the extremophile Thellungiella halophila in comparison with its relative Arabidopsis thaliana. Plant J. 44, 826–839. doi: 10.1111/j.1365-313X.2005.02587.x
Good, A. G., Shrawat, A. K., and Muench, D. G. (2004). Can less yield more? Is reducing nutrient input into the environment compatible with maintaining crop production? Trends Plant Sci. 9, 597–605. doi: 10.1016/j.tplants.2004.10.008
Griffith, M., Timonin, M., Wong, A. C. E., Gray, G. R., Akhter, S. R., Saldanha, M., et al. (2007). Thellungiella: an Arabidopsis-related model plant adapted to cold temperatures. Plant Cell Environ. 30, 529–538. doi: 10.1111/j.1365-3040.2007.01653.x
Guevara, D. R., Champigny, M. J., Tattersall, A., Dedrick, J., Wong, C. E., Li, Y., et al. (2012). Transcriptomic and metabolomics analysis of Yukon Thellungiella plants grown in cabinets and their natural habitat show phenotypic plasticity. BMC Plant Biol. 12:175. doi: 10.1186/1471-2229-12-175
Gutterman, Y. (2002). Survival Strategies of Annual Desert Plants (Adaptations of Desert Organisms). Heidelberg: Springer.
Hagemann, M., and Bauwe, H. (2016). Photorespiration and the potential to improve photosynthesis. Curr. Opin. Chem. Biol. 35, 109–116. doi: 10.1016/j.cbpa.2016.09.014
Han, M., Okamoto, M., Beatty, P. H., Rothstein, S. J., and Good, A. G. (2015). The genetics of nitrogen use efficiency in crop plants. Annu. Rev. Genet. 49, 269–289. doi: 10.1146/annurev-genet-112414-055037
Hanikenne, M., Talke, I. N., Haydon, M. J., Lanz, C., Nolte, A., Motte, P., et al. (2008). Evolution of metal hyperaccumulation required cis-regulatory changes and triplication of HMA4. Nature 453, 391–396. doi: 10.1038/nature06877
Hegazy, A. K., Barakat, H. N., and Kabiel, H. F. (2006). Anatomical significance of the hygrochastic movement in Anastatica hierochuntica. Ann. Bot. 97, 47–55. doi: 10.1093/aob/mcj011
Higashi, Y., Ohama, N., Ishikawa, T., Katori, T., Shimura, A., Kusakabe, K., et al. (2013). HsfA1d, a protein identified via FOX hunting using Thellungiella salsuginea cDNAs improves heat tolerance by regulating heat-stress-responsive gene expression. Mol. Plant 6, 411–422. doi: 10.1093/mp/sst024
Huang, C.-H., Sun, R., Hu, Y., Zeng, L., Zhang, N., Cai, L., et al. (2015). Resolution of Brassicaceae phylogeny using nuclear genes uncovers nested radiations and supports convergent morphological evolution. Mol. Biol. Evol. 33, 394–412. doi: 10.1093/molbev/msv226
Inan, G., Zhang, Q., Li, P., Wang, Z., Cao, Z., Zhang, H., et al. (2004). Salt cress. A halophyte and cryophyte Arabidopsis relative model system and its applicability to molecular genetic analyses of growth and development of extremophiles. Plant Physiol. 135, 1718–1737. doi: 10.1104/pp.104.041723
John, U. P., and Spangenberg, G. C. (2005). Xenogenomics: genomic bioprospecting in indigenous and exotic plants through EST discovery, cDNA microarray-based expression profiling and functional genomics. Comp. Funct. Genomics 6, 230–235. doi: 10.1002/cfg.475
Kant, S., Bi, Y.-M., and Rothstein, S. J. (2011). Understanding plant response to nitrogen limitation for the improvement of crop nitrogen use efficiency. J. Exp. Bot. 62, 1499–1509. doi: 10.1093/jxb/erq297
Kant, S., Bi, Y. M., Weretilnyk, E., Barak, S., and Rothstein, S. J. (2008). The Arabidopsis halophytic relative Thellungiella halophila tolerates nitrogen-limiting conditions by maintaining growth, nitrogen uptake, and assimilation. Plant Physiol. 147, 1168–1180. doi: 10.1104/pp.108.118125
Kant, S., Kant, P., Raveh, E., and Barak, S. (2006). Evidence that differential gene expression between the halophyte, Thellungiella halophila, and Arabidopsis thaliana is responsible for higher levels of the compatible osmolyte proline and tight control of Na+ uptake in T. halophila. Plant Cell Environ. 29, 1220–1234. doi: 10.1111/j.1365-3040.2006.01502.x
Kazachkova, Y., Batushansky, A., Cisneros, A., Tel-Zur, N., Fait, A., and Barak, S. (2013). Growth platform-dependent and -independent phenotypic and metabolic responses of Arabidopsis and its halophytic relative, Eutrema salsugineum, to salt stress. Plant Physiol. 162, 1583–1598. doi: 10.1104/pp.113.217844
Kiefer, M., Schmick, R., German, D. A., Mandakova, T., Lysak, M. A., Al-Shehbaz, I. A., et al. (2014). BrassiBase: introduction to a novel knowledge database on Brassicaceae evolution. Plant Cell Physiol 55, e3. doi: 10.1093/pcp/pct158
Koch, M. A., and German, D. (2013). Taxonomy and systematics are key to biological information: Arabidopsis, Eutrema (Thellungiella), Noccaea and Schrenkiella (Brassicaceae) as examples. Front. Plant Sci. 4:267. doi: 10.3389/fpls.2013.00267
Koenig, D., and Weigel, D. (2015). Beyond the thale: comparative genomics and genetics of Arabidopsis relatives. Nat. Rev. Genet. 16, 285–298. doi: 10.1038/nrg3883
Krall, J. P., and Edwards, G. E. (1992). Relationship between photosystem II activity and CO2 fixation in leaves. Physiol. Plant. 86, 180–187. doi: 10.1111/j.1399-3054.1992.tb01328.x
Kumar, A., and Bachhawat, A. K. (2012). Pyroglutamic acid: throwing light on a lightly studied metabolite. Curr. Sci. 102, 288–297.
Lamdan, N. L., Attia, Z. I. V., Moran, N., and Moshelion, M. (2012). The Arabidopsis-related halophyte Thellungiella halophila: boron tolerance via boron complexation with metabolites? Plant Cell Environ. 35, 735–746. doi: 10.1111/j.1365-3040.2011.02447.x
Laureau, C., De Paepe, R., Latouche, G., Moreno-Chacon, M., Finazzi, G., Kuntz, M., et al. (2013). Plastid terminal oxidase (PTOX) has the potential to act as a safety valve for excess excitation energy in the alpine plant species Ranunculus glacialis L. Plant Cell Environ. 36, 1296–1310. doi: 10.1111/pce.12059
Lawson, T., Davey, P. A., Yates, S. A., Bechtold, U., Baeshen, M., Baeshen, N., et al. (2014). C3 photosynthesis in the desert plant Rhazya stricta is fully functional at high temperatures and light intensities. New Phytol. 201, 862–873. doi: 10.1111/nph.12559
Lee, Y. P., Babakov, A., de Boer, B., Zuther, E., and Hincha, D. K. (2012). Comparison of freezing tolerance, compatible solutes and polyamines in geographically diverse collections of Thellungiella sp. and Arabidopsis thaliana accessions. BMC Plant Biol. 12:131. doi: 10.1186/1471-2229-12-131
Lesk, C., Rowhani, P., and Ramankutty, N. (2016). Influence of extreme weather disasters on global crop production. Nature 529, 84–87. doi: 10.1038/nature16467
Lichtenthaler, H. K. (1987). Chlorophylls and carotenoids: pigments of photosynthetic biomembranes. Methods Enzymol. 148, 350–382. doi: 10.1016/0076-6879(87)48036-1
Liepman, A. H., and Olsen, L. J. (2001). Peroxisomal alanine : glyoxylate aminotransferase (AGT1) is a photorespiratory enzyme with multiple substrates in Arabidopsis thaliana. Plant J. 25, 487–498. doi: 10.1046/j.1365-313x.2001.00961.x
Lifante, Z. D., Luque, T., and Barbara, C. S. (1992). Chromosome numbers of plants collected during Iter Mediterraneum II in Israel. Bocconea 26, 151–172. doi: 10.7320/Bocc26.151
Lisec, J., Schauer, N., Kopka, J., Willmitzer, L., and Fernie, A. R. (2006). Gas chromatography mass spectrometry-based metabolite profiling in plants. Nat. Protoc. 1, 387–396. doi: 10.1038/nprot.2006.59
Lobell, D. B., Schlenker, W., and Costa-Roberts, J. (2011). Climate trends and global crop production since 1980. Science 333, 616–620. doi: 10.1126/science.1204531
Lugan, R., Niogret, M.-F., Leport, L., Guégan, J.-P., Larher, F. R., Savouré, A., et al. (2010). Metabolome and water homeostasis analysis of Thellungiella salsuginea suggests that dehydration tolerance is a key response to osmotic stress in this halophyte. Plant J. 64, 215–229. doi: 10.1111/j.1365-313X.2010.04323.x
MacGregor, D. R., Deak, K. I., Ingram, P. A., and Malamy, J. E. (2008). Root system architecture in Arabidopsis grown in culture is regulated by sucrose uptake in the aerial tissues. Plant Cell 20, 2643–2660. doi: 10.1105/tpc.107.055475
McAllister, C. H., Beatty, P. H., and Good, A. G. (2012). Engineering nitrogen use efficient crop plants: the current status. Plant Biotechnol. J. 10, 1011–1025. doi: 10.1111/j.1467-7652.2012.00700.x
Millennium Ecosystem Assessment (2005). Ecosystems and Human Well-Being: Desertification Synthesis. Washington, DC: World Resources Institute.
Mittler, R. (2006). Abiotic stress, the field environment and stress combination. Trends Plant Sci. 11, 15–19. doi: 10.1016/j.tplants.2005.11.002
Mittler, R., and Blumwald, E. (2010). Genetic engineering for modern agriculture: challenges and perspectives. Annu. Rev. Plant Biol. 61, 443–462. doi: 10.1146/annurev-arplant-042809-112116
M’rah, S., Ouergh, Z., Eymery, F., Rey, P., Hajji, M., Grignon, C., et al. (2007). Efficiency of biochemical protection against toxic effects of accumulated salt differentiates Thellungiella halophila from Arabidopsis thaliana. J. Plant Physiol. 164, 375–384. doi: 10.1016/j.jplph.2006.07.013
Munns, R. (2002). Comparative physiology of salt and water stress. Plant Cell Environ. 25, 239–250. doi: 10.1046/j.0016-8025.2001.00808.x
Murashige, T., and Skoog, F. (1962). A revised medium for rapid growth and bioassays with tobacco tissue cultures. Physiol. Plant. 15, 473–497. doi: 10.1111/j.1399-3054.1962.tb08052.x
Nishizawa, A., Yabuta, Y., and Shigeoka, S. (2008). Galactinol and raffinose constitute a novel function to protect plants from oxidative damage. Plant Physiol. 147, 1251–1263. doi: 10.1104/pp.108.122465
Novitskaya, L., Trevanion, S. J., Driscoll, S., Foyer, C. H., and Noctor, G. (2002). How does photorespiration modulate leaf amino acid contents? A dual approach through modelling and metabolite analysis. Plant Cell Environ. 25, 821–835. doi: 10.1046/j.1365-3040.2002.00866.x
Obata, T., and Fernie, A. R. (2012). The use of metabolomics to dissect plant responses to abiotic stresses. Cell. Mol. Life Sci. 69, 3225–3243. doi: 10.1007/s00018-012-1091-5
Oh, D.-H., Dassanayake, M., Bohnert, H. J., and Cheeseman, J. M. (2012). Life at the extreme: lessons from the genome. Genome Biol. 13, 241. doi: 10.1186/gb-2012-13-3-241
Oh, D.-H., Dassanayake, M., Haas, J. S., Kropornika, A., Wright, C., Paino d’Urzo, M., et al. (2010). Genome structures and halophyte-specific gene expression of the extremophile Thellungiella parvula in comparison with Thellungiella salsuginea (Thellungiella halophila) and Arabidopsis. Plant Physiol. 154, 1040–1052. doi: 10.1104/pp.110.163923
Oh, D.-H., Hong, H., Lee, S. Y., Yun, D.-J., Bohnert, H. J., and Dassanayake, M. (2014). Genome structures and transcriptomes signify niche adaptation for the multiple-ion-tolerant extremophyte Schrenkiella parvula. Plant Physiol. 164, 2123–2138. doi: 10.1104/pp.113.233551
Ohkama-Ohtsu, N., Oikawa, A., Zhao, P., Xiang, C., Saito, K., and Oliver, D. J. (2008). A γ-glutamyl transpeptidase-independent pathway of glutathione catabolism to glutamate via 5-oxoproline in Arabidopsis. Plant Physiol. 148, 1603–1613. doi: 10.1104/pp.108.125716
Orsini, F., D’Urzo, M. P., Inan, G., Serra, S., Oh, D. H., Mickelbart, M. V., et al. (2010). A comparative study of salt tolerance parameters in 11 wild relatives of Arabidopsis thaliana. J. Exp. Bot. 61, 3787–3798. doi: 10.1093/jxb/erq188
Perez-Torres, E., García, A., Dinamarca, J., Alberdi, M., Gutierrez, A., Gidekel, M., et al. (2004). The role of photochemical quenching and antioxidants in photoprotection of Deschampsia antarctica. Funct. Plant Biol. 31, 731–741. doi: 10.1071/FP03082
Raun, W. R., and Johnson, G. V. (1999). Improving nitrogen use efficiency for cereal production. Agron. J. 91, 357–363. doi: 10.2134/agronj1999.00021962009100030001x
Ruhland, C. T., Xiong, F. S., Clark, W. D., and Day, T. A. (2005). The influence of ultraviolet-B radiation on growth, hydroxycinnamic acids and flavonoids of Deschampsia antarctica during springtime ozone depletion in Antarctica. Photochem. Photobiol. 81, 1086–1093. doi: 10.1562/2004-09-18-RA-321
Saeed, A. I., Sharov, V., White, J., Li, J., Liang, W., Bhagabati, N., et al. (2003). TM4: a free, open-source system for microarray data management and analysis. Biotechniques 34, 374–378.
Sanchez, D. H., Pieckenstain, F. L., Escaray, F., Erban, A., Kraemer, U., Udvardi, M. K., et al. (2011). Comparative ionomics and metabolomics in extremophile and glycophytic Lotus species under salt stress challenge the metabolic pre-adaptation hypothesis. Plant Cell Environ. 34, 605–617. doi: 10.1111/j.1365-3040.2010.02266.x
Schreiber, K. J., Austin, R. S., Gong, Y., Zhang, J., Fung, P., Wang, P. W., et al. (2012). Forward chemical genetic screens in Arabidopsis identify genes that influence sensitivity to the phytotoxic compound sulfamethoxazole. BMC Plant Biol. 12:226. doi: 10.1186/1471-2229-12-226
Smaoui, A., Jouini, J., Rabhi, M., Bouzaien, G., Albouchi, A., and Abdelly, C. (2011). Physiological and anatomical adaptations induced by flooding in Cotula coronopifolia. Acta Biol. Hung. 62, 182–193. doi: 10.1556/ABiol.62.2011.2.8
Smirnoff, N. (1996). Botanical briefing: the function and metabolism of ascorbic acid in plants. Ann. Bot. 78, 661–669. doi: 10.1006/anbo.1996.0175
Smirnoff, N., and Cumbes, Q. J. (1989). Hydroxyl radical scavenging activity of compatible solutes. Phytochemistry 28, 1057–1060. doi: 10.1016/0031-9422(89)80182-7
Stepien, P., and Johnson, G. N. (2009). Contrasting responses of photosynthesis to salt stress in the glycophyte Arabidopsis and the halophyte Thellungiella: role of the plastid terminal oxidase as an alternative electron sink. Plant Physiol. 149, 1154–1165. doi: 10.1104/pp.108.132407
Stratonovitch, P., and Semenov, M. A. (2015). Heat tolerance around flowering in wheat identified as a key trait for increased yield potential in Europe under climate change. J. Exp. Bot. 66, 3599–3609. doi: 10.1093/jxb/erv070
Streb, P., Josse, E.-M., Gallouet, E., Baptist, F., Kuntz, M., and Cornic, G. (2005). Evidence for alternative electron sinks to photosynthetic carbon assimilation in the high mountain plant species Ranunculus glacialis. Plant Cell Environ. 2, 1123–1135. doi: 10.1111/j.1365-3040.2005.01350.x
Sun, Q., Gao, F., Zhao, L., Li, K., and Zhang, J. (2010). Identification of a new 130 bp cis-acting element in the TsVP1 promoter involved in the salt stress response from Thellungiella halophila. BMC Plant Biol. 10:90. doi: 10.1186/1471-2229-10-90
Sweetlove, L. J., Beard, K. F. M., Nunes-Nesi, A., Fernie, A. R., and Ratcliffe, G. R. (2010). Not just a circle: flux modes in the plant TCA cycle. Trends Plant Sci. 15, 462–470. doi: 10.1016/j.tplants.2010.05.006
Taji, T., Ohsumi, C., Iuchi, S., Seki, M., Kasuga, M. K., and Kobayashi, M. (2002). Important roles of drought- and cold-inducible genes for galactinol synthase in stress tolerance in Arabidopsis thaliana. Plant J. 29, 417–426. doi: 10.1046/j.0960-7412.2001.01227.x
Taji, T., Seki, M., Satou, M., Sakurai, T., Kobayashi, M., Ishiyama, K., et al. (2004). Comparative genomics in salt tolerance between Arabidopsis and Arabidopsis-related halophyte salt cress using Arabidopsis microarray. Plant Physiol. 135, 1697–1709. doi: 10.1186/1471-2229-10-261
Tilman, D., Balzer, C., Hill, J., and Belfort, B. L. (2011). Global food demand and the sustainable intensification of agriculture. Proc. Natl. Acad. Sci. U.S.A. 108, 20260–20264. doi: 10.1073/pnas.1116437108
Timm, S., Florian, A., Arrivault, S., Stitt, M., Fernie, A. R., and Bauwe, H. (2012). Glycine decarboxylase controls photosynthesis and plant growth. FEBS Lett. 586, 3692–3697. doi: 10.1016/j.febslet.2012.08.027
United Nations, Department of Economic and Social Affairs, Population Division (2011). World Population Prospects: The 2010 Revision, Vol. I. Comprehensive Tables. ST/ESA/SER.A/313. New York, NY: United Nations, Department of Economic and Social Affairs, Population Division.
Urano, K., Maruyama, K., Ogata, Y., Morishita, Y., Takeda, M., Sakurai, N., et al. (2009). Characterization of the ABA-regulated global responses to dehydration in Arabidopsis by metabolomics. Plant J. 57, 1065–1078. doi: 10.1111/j.1365-313X.2008.03748.x
Uzilday, B., Ozgur, R., Sekmen, A. H., Yildiztugay, E., and Turkan, I. (2014). Changes in the alternative electron sinks and antioxidant defence in chloroplasts of the extreme halophyte Eutrema parvulum (Thellungiella parvula) under salinity. Ann. Bot. 115, 449–463. doi: 10.1093/aob/mcu184
Velasco, V. M. E., Mansbridge, J., Bremner, S., Carruthers, K., Summers, P. S., Sung, W. W. L., et al. (2016). Acclimation of the crucifer Eutrema salsugineum to phosphate limitation is associated with constitutively high expression of phosphate-starvation genes. Plant Cell Environ 39, 1818–1834. doi: 10.1111/pce.12750
Vera-Estrella, R., Barkla, B. J., and Pantoja, O. (2014). Comparative 2D-DIGE analysis of salinity responsive microsomal proteins from leaves of salt-sensitive Arabidopsis thaliana and salt-tolerant Thellungiella salsuginea. J. Proteomics 111, 113–127. doi: 10.1016/j.jprot.2014.05.018
Volkov, V., and Amtmann, A. (2006). Thellungiella halophila, a salt-tolerant relative of Arabidopsis thaliana, has specific root ion channel features supporting K+/Na+ homeostasis under salinity stress. Plant J. 48, 342–353. doi: 10.1111/j.1365-313X.2006.02876.x
Volkov, V., Wang, B., Dominy, P. J., Fricke, W., and Amtmann, A. (2003). Thellungiella halophila, a salt-tolerant relative of Arabidopsis thaliana, possesses effective mechanisms to discriminate between potassium and sodium. Plant Cell Environ. 27, 1–14. doi: 10.1046/j.0016-8025.2003.01116.x
Wang, B., Davenport, R. J., Volkov, V., and Amtmann, A. (2006). Low unidirectional sodium influx into root cells restricts net sodium accumulation in Thellungiella halophila, a salt-tolerant relative of Arabidopsis thaliana. J. Exp. Bot. 57, 1161–1170. doi: 10.1093/jxb/erj116
White, A. J., and Critchley, C. (1999). Rapid light curves?: a new fluorescence method to assess the state of the photosynthetic apparatus. Photosyn. Res. 59, 63–72. doi: 10.1023/A:1006188004189
Wu, H.-J., Zhang, Z., Wang, J.-Y., Oh, D.-H., Dassanayake, M., Liu, B., et al. (2012). Insights into salt tolerance from the genome of Thellungiella salsuginea. Proc. Natl. Acad. Sci. U.S.A. 109, 12219–12224. doi: 10.1073/pnas.1209954109
Xiong, F. S., and Day, T. A. (2001). Effect of solar ultraviolet-B radiation during springtime ozone depletion on photosynthesis and biomass production of Antarctic vascular plants. Plant Physiol. 125, 738–775. doi: 10.1104/pp.125.2.738
Yang, R., Jarvis, D. E., Chen, H., Beilstein, M. A., Grimwood, J., Jenkins, J., et al. (2013). The reference genome of the halophytic plant Eutrema salsugineum. Front. Plant. Sci. 4:46. doi: 10.3389/fpls.2013.00046
Yates, S. A., Chernukhin, I., Alvarez-Fernandez, R., Bechtold, U., Baeshen, M., Baeshen, N., et al. (2014). The temporal foliar transcriptome of the perennial C3 desert plant Rhazya stricta in its natural environment. BMC Plant Biol. 14:2. doi: 10.1186/1471-2229-14-2
Yobi, A., Wone, B. W. M., Xu, W., Alexander, D. C., Guo, L., Ryals, J. A., et al. (2012). Comparative metabolic profiling between desiccation-sensitive and desiccation-tolerant species of Selaginella reveals insights into the resurrection trait. Plant J. 72, 983–999. doi: 10.1111/tpj.12008
Zaady, E. (2005). Seasonal change and nitrogen cycling in a patchy negev desert: a review. Arid Land Res. and Manag. 19, 111–124. doi: 10.1080/15324980590916512
Keywords: abiotic stress, Arabidopsis relatives, Brassicaceae, desert plants, Eutrema salsugineum, extremophile plants, halophytes, extremophytes
Citation: Eshel G, Shaked R, Kazachkova Y, Khan A, Eppel A, Cisneros A, Acuna T, Gutterman Y, Tel-Zur N, Rachmilevitch S, Fait A and Barak S (2017) Anastatica hierochuntica, an Arabidopsis Desert Relative, Is Tolerant to Multiple Abiotic Stresses and Exhibits Species-Specific and Common Stress Tolerance Strategies with Its Halophytic Relative, Eutrema (Thellungiella) salsugineum. Front. Plant Sci. 7:1992. doi: 10.3389/fpls.2016.01992
Received: 07 September 2016; Accepted: 15 December 2016;
Published: 17 January 2017.
Edited by:
Vasileios Fotopoulos, Cyprus University of Technology, CyprusReviewed by:
Lars Matthias Voll, University of Erlangen-Nuremberg, GermanyCopyright © 2017 Eshel, Shaked, Kazachkova, Khan, Eppel, Cisneros, Acuna, Gutterman, Tel-Zur, Rachmilevitch, Fait and Barak. This is an open-access article distributed under the terms of the Creative Commons Attribution License (CC BY). The use, distribution or reproduction in other forums is permitted, provided the original author(s) or licensor are credited and that the original publication in this journal is cited, in accordance with accepted academic practice. No use, distribution or reproduction is permitted which does not comply with these terms.
*Correspondence: Simon Barak, c2ltb25AYmd1LmFjLmls
Disclaimer: All claims expressed in this article are solely those of the authors and do not necessarily represent those of their affiliated organizations, or those of the publisher, the editors and the reviewers. Any product that may be evaluated in this article or claim that may be made by its manufacturer is not guaranteed or endorsed by the publisher.
Research integrity at Frontiers
Learn more about the work of our research integrity team to safeguard the quality of each article we publish.