- 1The Nurturing Station for the State Key Laboratory of Subtropical Silviculture, Zhejiang A & F University, Zhejiang, China
- 2New Zealand Institute for Plant and Food Research Limited, Ruakura Research Centre, Hamilton, New Zealand
- 3Key Laboratory of Soil Contamination Bioremediation of Zhejiang Province, Zhejiang A & F University, Zhejiang, China
Magnesium (Mg2+) has been shown to reduce the physiological and biochemical stress in plants caused by heavy metals. To date our understanding of how Mg2+ ameliorates the adverse effects of heavy metals in plants is scarce. The potential effect of Mg2+ on lead (Pb2+) toxicity in plants has not yet been studied. This study was designed to clarify the mechanism of Mg2+-induced alleviation of lead (Pb2+) toxicity. Torreya grandis (T. grandis) seedlings were grown in substrate contaminated with 0, 700 and 1400 mg Pb2+ per kg-1 and with or without the addition of 1040 mg kg-1 Mg2+. Growth parameters, concentrations of Pb2+ and Mg2+ in the plants’ shoots and roots, photosynthetic pigment, gas exchange parameters, the maximum quantum efficiency (Fv/Fm), root oxidative activity, ultrastructure of chloroplasts and root growth were determined to analyze the effect of different Pb2+ concentrations on the seedlings as well as the potential ameliorating effect of Mg2+ on the Pb2+ induced toxicity. All measurements were tested by a one-way ANOVA for the effects of treatments. The growth of T. grandis seedlings cultivated in soils treated with 1400 mg kg-1 Pb2+ was significantly reduced compared with that of plants cultivated in soils treated with 0 or 700 mg kg-1 Pb2+. The addition of 1040 mg kg-1 Mg2+ improved the growth of the Pb2+-stressed seedlings, which was accompanied by increased chlorophyll content, the net photosynthetic rate and Fv/Fm, and enhanced chloroplasts development. In addition, the application of Mg2+ induced plants to accumulate five times higher concentrations of Pb2+ in the roots and to absorb and translocate four times higher concentrations of Mg2+ to the shoots than those without Mg2+ application. Furthermore, Mg2+ addition increased root growth and oxidative activity, and protected the root ultrastructure. To the best of our knowledge, our study is the first report on the mechanism of Mg2+-induced alleviation of Pb2+ toxicity. The generated results may have important implications for understanding the physiological interactions between heavy metals and plants, and for successful management of T. grandis plantations grown on soils contaminated with Pb2+.
Introduction
Heavy metal pollution has become a global environmental threat (Krabbenhoft and Sunderland, 2013; Chen et al., 2015). Among metal contaminants, lead (Pb2+) is a major concern because of its extensive distribution in the environment and the substantial environmental and human health problems it can cause. Major sources of Pb2+ pollution include mining and smelting activities as well as Pb2+-containing paints, gasoline, explosives, sewage sludge and fertilizers (Sharma and Dubey, 2005; Buekers et al., 2009). When plants are exposed to Pb2+, even at micromolar levels, adverse effects can occur on plant growth (Hadi et al., 2010), root elongation (Liu et al., 2000), seed germination (Lamhamdi et al., 2011), seedling development (Kaur et al., 2015), chlorophyll production (Rashid and Popovic, 1990), chloroplast lamellar organization (Hu et al., 2007), and antioxidant enzymes system (Gupta et al., 2009, 2010). However, the toxicological response to Pb2+ varies depending on the plant species and tissues analyzed (Pourrut et al., 2011). Huang and Cunningham (1996) showed significant differences in the uptake and translocation of Pb2+ among Triticum aestivum, Thlaspi rotundifolium, and Thlaspi caerulescens. Mimosa caesalpiniaefolia was more tolerant to high Pb2+ concentrations in soil than Erythrina speciosa and Schizolobium parahyba (de Souza et al., 2012). Huang and Cunningham (1996) found that some dicot species can accumulate significantly higher concentrations of Pb2+ in the roots than some monocot species.
Heavy metals could be taken up by cation transporters such as members of the ZIP (Zn-regulated transporter/Fe-regulated transporter-like protein) and natural resistance-associated macrophage protein families (Eide et al., 1996; Korshunova et al., 1999; Guerinot, 2000; Thomine et al., 2000). These bivalent cation transporters are also important uptake systems for essential elements. Therefore, nutrients such as magnesium (Mg2+) are considered to contribute to plants’ tolerance to heavy metal exposure owing to their chemical similarity as well as sharing common transporters with heavy metals (Guerinot, 2000; Pittman, 2005). Over the last decade, studies have revealed the ability of Mg2+ to mitigate heavy metal toxicity caused by aluminum (Al3+) and cadmium (Cd2+) (Kashem and Kawai, 2007; Bose et al., 2011). Kashem and Kawai (2007) reported that adding Mg2+ to nutrient solutions reduced Cd concentrations in plants and enhanced the growth of plants suffering from Cd toxicity. Hermans et al. (2011) indicated that the protective effect of Mg2+ against Cd toxicity may be at least partly attributed to the protection of the photosynthetic apparatus. However, only few studies have investigated the effect of Mg2+ on Pb2+ toxicity. Therefore, we explored the effect of Mg2+ on Pb2+ toxicity and the possible mechanism of Mg2+-induced alleviation of Pb2+ toxicity using a local species Torreya grandis (T. grandis).
Torreya grandis is a gymnosperm tree species belonging to the Taxaceae family, mainly grown in eastern China with significant economic value because of its valuable drupe-like fruits with medicinal effects from its anthelmintic, antitussive, carminative, laxative, antifungal, antibacterial, and antitumor properties (Huang et al., 2001). As the demand for the fruit increased, the acreage of T. grandis has rapidly expanded, and the management intensity has increased with higher inputs of fertilizers and pesticides, such as lead arsenate. In addition, soils near highways, which are usually polluted by exhaust emissions, have also been used for growing T. grandis. Therefore, T. grandis is likely to face with Pb2+ stress. It remains unclear whether T. grandis can be tolerant to high level of Pb2+ stress. Thus, in this study, we performed a pot experiment to test the following hypotheses: (1) High level Pb2+ stress inhibits the growth of T. grandis seedlings; (2) Mg2+ can effectively ameliorate the negative effects of lead stress on the growth of T. grandis seedlings. The information obtained in this study is valuable for the propagation and cultivation of T. grandis under Pb2+ stress conditions.
Materials and Methods
Plant Material and Growth Conditions During All Experiments
All experiments were conducted on the Zhejiang A & F University campus, Lin’an City, Zhejiang province (330°23′N, 119°72′E), China. Two-year-old uniform and healthy T. grandis seedlings (mean ground diameter 5 ± 0.5 mm and seedling height 35 ± 2 cm) were transplanted into plastic pots (16.5 cm inner diameter, 18 cm height, with holes in the bottom, one seedling per pot) filled with 2.5 kg of sterilized substrate mixture of perlite and quartz sand (1:1, v/v). All pots were irrigated with 200 ml of Hoagland’s nutrient solution (3.0 mM KNO3, 2.5 mM Ca(NO3)2, 1.0 mM MgSO4. 7H2O, 1.2 μM FeEDTA, 4.0 μM MnCl2, 22.0 μM H3BO3, 0.4 μM ZnSO4, 0.05 μM Na2MoO4, 1.6 μM CuSO4, and 1.0 μM KH2PO4) every three days and maintained at 75% field capacity of the growth substrate to keep the plants well watered. Day and night temperature was kept between 18.0 and 32.0°C and the relative humidity ranged between 50 and 80%. The light intensity in the greenhouse was monitored daily with an external quantum sensor attached to LI-6400 (Li-COR, Lincoln, NE, USA) and kept within the range of 500–800 μmol m-2 s-1 photosynthetically active radiation (PAR) above the plants.
First Experiment: Exposure of Seedlings to Pb2+
The first experiment to determine the lead concentration that adversely affected T. grandis seedlings was carried out between 1 May and 31 June 2014. One month after transplantation of the seedlings, the height and ground diameter of each seedling were measured as reference values. A completely randomized design with three replications per treatment and five plants per replication was chosen. Total of 45 seedlings were exposed to Pb2+ supplied as Pb (NO3)2 at concentrations of 0 (control), 700 and 1400 mg Pb2+ kg-1 growth substrate. These concentrations were selected based on a report by Huang et al. (2006), who found that Pb2+-concentrations in soil exceeding 1000 mg kg-1 affected the growth of Pinus rigida. After 60 days, the height and ground diameters of the seedlings were recorded.
Second Experiment: Exposure of Seedlings to Pb2+ and Mg2+
The above experiment showed that 1400 mg kg-1 Pb2+ caused Pb2+ toxicity in T. grandis seedlings. Hence, further studies on the effect of Mg2+ on Pb2+ toxicity were restricted to the control and the 1400 mg kg-1 Pb2+ treatments. This second experiment was conducted during 1 May to 31 June 2015. Magnesium (with the irrigation water) was supplied as MgCl2 at 1040 mg kg-1. In total, the following four treatments (total of 60 seedlings) were established: T1 (control without Mg2+ addition); T2 (control with 1040 mg kg-1 Mg2+); T3 (1400 mg kg-1 Pb2+ without 1040 mg kg-1 Mg2+) and T4 (1400 mg kg-1 Pb2+ with 1040 mg kg-1 Mg2+). A completely randomized design with three replications per treatment and five plants per replication was set up.
Plant Harvest
After 60 days of the second experiment, the third and fourth leaves from the plant top, which had been completely developed when Pb2+ treatment started, were collected from all plants, cleaned with tissue paper to remove any surface contamination, immediately frozen in liquid nitrogen and stored at -70°C. Plant growth, concentrations of Pb2+ and Mg2+ in shoots and roots, chlorophyll concentration, root oxidative activity, photosynthesis and ultrastructure of chloroplasts and roots were determined for all samples.
Growth and Morphology Analysis
After 60 days of the two experiments, all seedlings were harvested and separated into shoots and roots for growth and morphology analyses. Shoot biomass and total biomass were measured after drying of the shoots and roots at 80°C for 4 days. Seedling height was defined as the height of the plant from the top of the growth medium to the tip of the uppermost shoot.
Determination of Pb2+ and Mg2+ Concentrations in Plant Shoots and Roots
To determine the concentrations of Pb2+ and Mg2+ in the shoots and roots, the dried plant materials were grounded with a stainless steel mill and passed through a 0.25 mm sieve for analysis of Pb2+ and Mg2+. An aliquot of 0.1 g of the dried plant materials of each treatment was digested with HNO3–HClO4 (4:1, v/v), and the digest was diluted with deionized water (DW) to a final volume of 50 mL. Concentrations of Pb2+ and Mg2+ in the filtrates were analyzed by flame atomic absorption spectroscopy (Perkin Elmer Analyser 300, England). The Pb2+ and Mg2+ concentrations in the entire plant were calculated following Zhang et al. (2011) and expressed in mg kg-1 DW and mg g-1 DW, respectively.
Pigment Concentration in Leaves
Approximately 0.1 g of finely cut and well-mixed fresh plant sample, which was taken from healthy and fully developed leaves at the same position in each treatment, was repeatedly extracted with 8 mL of 95% ethanol (100%, Sinopharm Chemical Reagent Company, Shanghai, China). Pigment was extracted at 4°C for 24 h in darkness and shaken three or four times until the leaf samples blanched (no green color in the leaf tissue). The absorbance was measured with a Shimadzu UV-2550 spectrophotometer (Kyoto, Japan) at 664, 649, and 470 nm after centrifugation of the mixture. The chlorophyll a (Chla), chlorophyll b (Chlb), total chlorophyll (Chl(a+b)), and carotenoid (Car) contents were calculated using the following formulas (Lichtenthaler, 1987). Results are expressed in mg g-1 fresh weight (FW).
Where, Ca, Cb, Ca+b, and Cx+c were the concentrations of Chla, Chlb, Chl (a+b), and Car, respectively. A664, A649, and A470 were the absorbances of pigment extract solution at 664, 649, and 470 nm wavelengths, respectively.
Photosynthetic Parameters and the Maximum Quantum Efficiency of Psii Photochemistry (Fv/Fm)
The youngest healthy and fully developed leaves randomly selected from the first branch were chosen for gas exchange measurements. Field gas exchange measurements were conducted with a LI-6400 portable photosynthesis system (Li-COR, Inc. Lincoln, NE, USA) with a standard leaf chamber equipped with a 6400-02B LED light source (LI-6400, Li-COR, Lincoln, NE, USA). Measurements were conducted at an air concentration of 21% O2, 400 μmol mol-1 carbon dioxide (CO2), 800 μmol m-2 s-1 PAR, 50% relative humidity and a temperature of 20 ± 2°C. The gas exchange measurements were performed on sunny days from 8:30 to 11:30 am.
Chlorophyll fluorescence (Fv/Fm) was determined in the morning (08:00 am–11:00 am) on the healthy and fully developed leaves with a pulse modulation fluorometer (PAM-2500, Walz, Effeltrich, Germany). After 30 min of adaptation to the dark (Tang et al., 2015), the minimum fluorescence (Fo) was determined in a measuring light of approximately 0.5 μmol photon m-2 s-1, and the maximum fluorescence (Fm) was determined under a 0.8-s saturating flash of 10,000 μmol photon m-2 s-1. The Fv/Fm value was calculated as (Fm-Fo)/Fm (Maxwell and Johnson, 2000).
Determination of Root Morphological Traits
After gently washing the roots with deionized water, the total length, volume, and surface area of the root samples were determined by image analysis. The roots were photographed and then the images were analyzed with the root image analysis system software WinRHIZO1.
Root Oxidative Activity
The root oxidative activity was measured according to the method of Mishra (2012) with a slight modification. About 3 g fresh root were immersed in 300 ml of 20 ppm á-naphthylamine solution for 10 minutes to exclude any initial rapid absorption of á-naphthylamine by roots. The intact roots were then transferred to another vial with 300 ml of 20 ppm of á-naphthylamine solution and incubated for four hours at 25 ± 1°C. Then, 2 ml of the incubated solution were mixed with 10 ml of 0.1% sulfanilic acid (in 3% acetic acid) and 2 ml of 50 ppm NaNO2, and diluted to 25 ml using distilled water. The absorbance of the colored solution was determined at 530 nm using spectrophotometry. Root oxidative activity was expressed as μg á-naphthylamine h-1 g-1 FW.
Ultrastructure of Chloroplast and Root
To examine the chloroplast ultrastructure of mesophyll cells, fresh leaves were immediately fixed in 2.5% (v/v) glutaraldehyde (0.1 mol L-1 phosphate buffer, pH 7.2) for at least 48 h after detachment from the plants. The samples were immersed in 1% (v/v) osmium acid for post-fixation, embedded in resin, and ultrathin sectioned for transmission electron microscopy (H7650, Hitachi, Tokyo, Japan).
Data Analysis
Because the Pb2+ and Mg2+ treatments were not applied independently to each seedling, the plants in each treatment combination were not true replicates (Hurlbert, 1984; Maherali and DeLucia, 2000). Therefore, averages of subsamples (five seedlings per replicate) were used for the analysis of variance. All measurements were tested by a one-way ANOVA for the effects of treatments (combinations of Pb2+ and Mg2+ concentration). The effects were considered significant at P < 0.05. Before ANOVA, data were checked for normality and homogeneity of variances, and log-transformed to correct deviations from these assumptions when needed. Significant differences among treatment means were analyzed using Tukey’s multiple comparison post hoc tests. The used statistical software package was SPSS 16 for Windows (SPSS Inc., Chicago, IL, USA).
Results
Effect of Lead on Plant Growth and Development
Plants grown for 60 days at 0, 700, and 1400 mg Pb2+ per kg-1 soil could be visually differentiated. Plants grown at 700 mg kg-1 were larger than those of other treatments (Figure 1). Compared with the control, soil contamination of 700 mg Pb2+ kg-1 significantly increased the growth of T. grandis seedlings (P = 0.0001, Figure 2). However, the 1400 mg kg-1 Pb2+ treatment inhibited plant growth and ground diameter by 60.5% (P = 0.0001) and 83.0% (P = 0.0001), respectively (Figure 2).
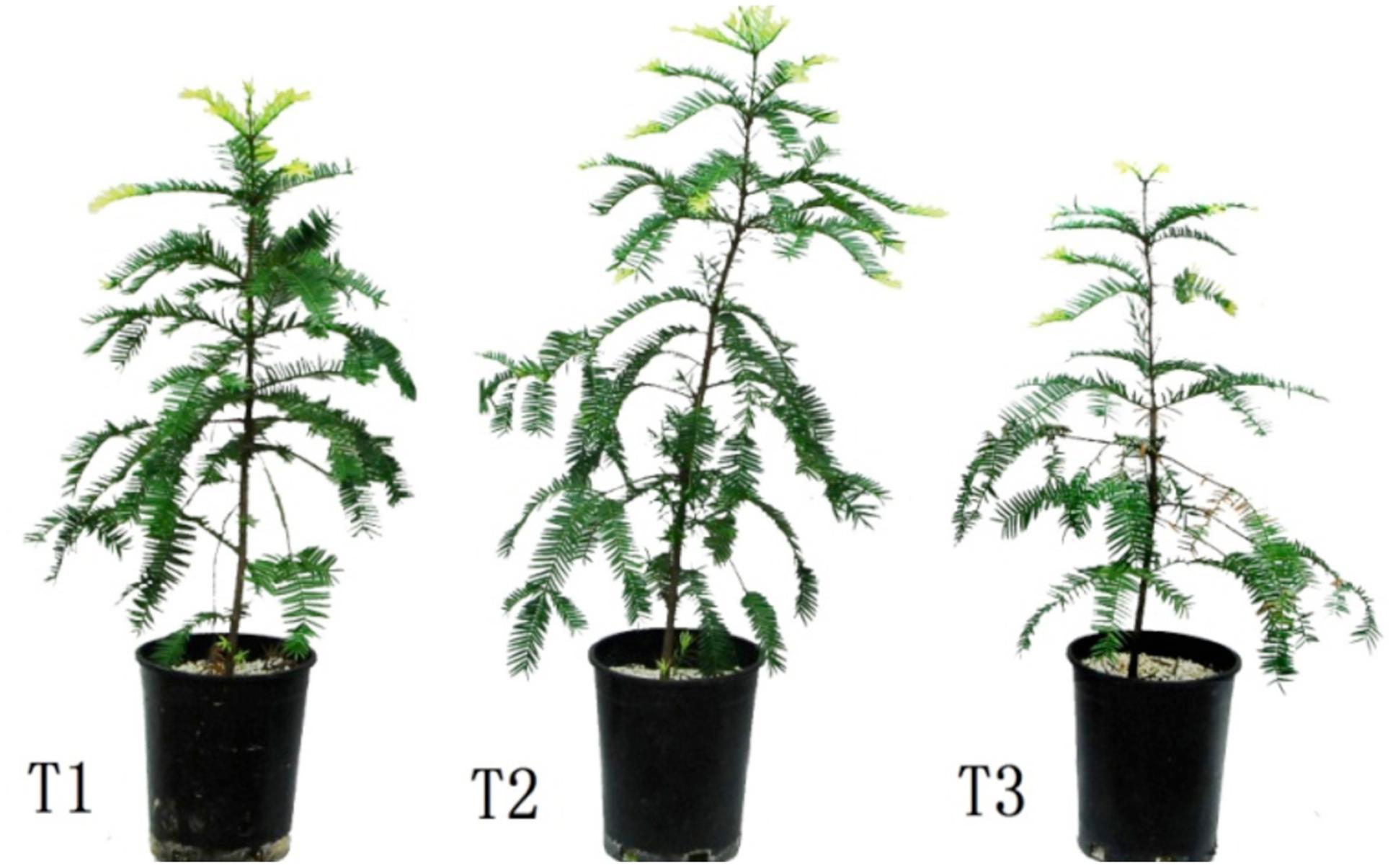
FIGURE 1. Effect of Pb2+ addition to the growth medium on T. grandis seedlings. T1, control, T2, 700 mg kg-1 Pb2+, T3, 1400 mg kg-1 Pb2+.
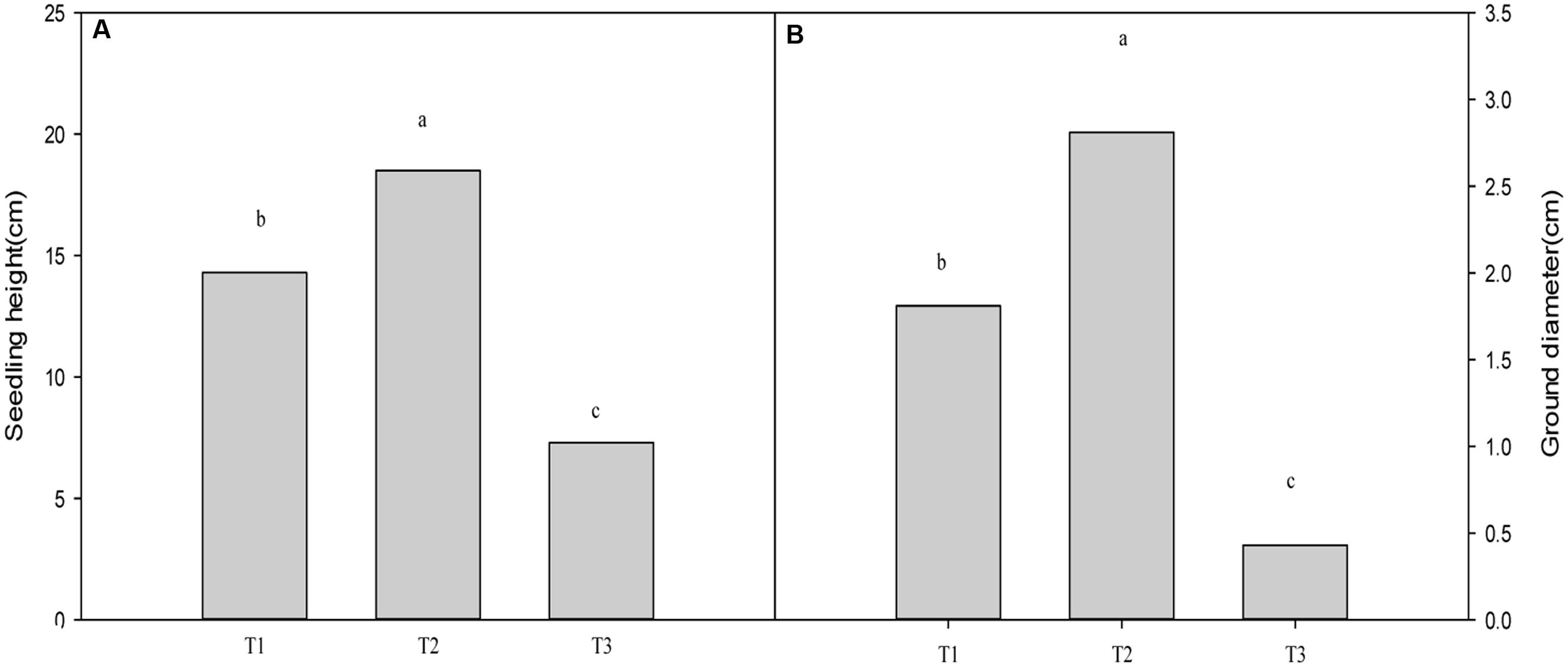
FIGURE 2. Effect of Pb2+ addition to growth medium on the seedling height (A) and ground diameter (B) of T. grandis seedlings. T1, control, T2, 700 mg kg-1 Pb2+, T3, 1400 mg kg-1 Pb2+. Data points and error bars represent mean ± standard deviation (n = 3). Different lower case letters above the columns indicate significant (P < 0.05) difference between treatments.
Effect of Mg2+ on Dry Biomass, Plant Growth, and Morphological Traits of Roots Under Lead Toxicity
Compared with the control seedlings, exposure of plants to 1400 mg kg-1 Pb2+ in a growth medium for 60 days significantly decreased the dry mass of shoots and roots by 19.6% (P = 0.0002) and 24.1% (P = 0.0038), respectively (Table 1). The Mg2+-treated plants had significantly higher shoot (P = 0.0172) and root (P = 0.0118) dry mass than plants under Pb2+ toxicity without Mg2+ application (Table 1). However, Mg2+ had no significant effect on the dry mass of shoots (P = 0.5937) and roots (P = 0.9235) of the non-Pb2+-stressed plants. The leaf area (P = 0.0001) and seedling height (P = 0.0001) of plants under Pb2+ toxicity were significantly lower than those of the control plants. Application of Mg2+ increased the leaf area (P = 0.0004) and seedling height (P = 0.0001) of plants under Pb2+ toxicity (P < 0.05, Table 1). However, there were no significant differences in leaf area (P = 0.4141) and seedling height (P = 0.1411) in the non-lead-stressed plants treated with or without Mg2+.
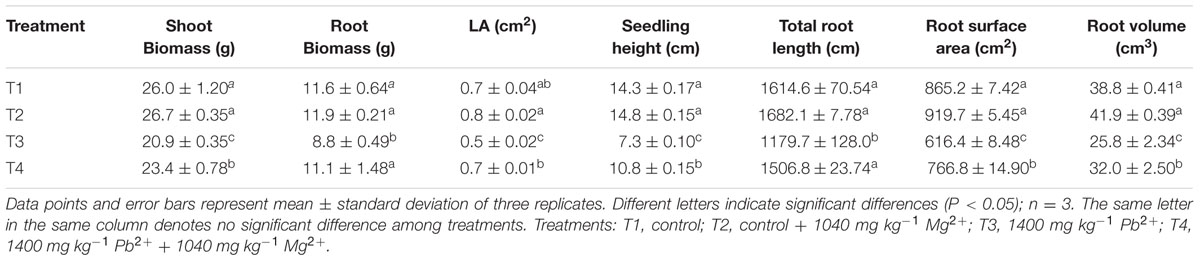
TABLE 1. Effects of Mg2+ on the dry biomass of shoots and roots, leaf area (LA), seedling height, and root morphological traits of T. grandis seedlings grown under Pb2+ toxicity.
Total length, surface area and volume of plant roots under Pb2+ toxicity decreased significantly by 26.9% (P = 0.0004), 28.8% (P = 0.0001), and 33.5% (P = 0.0001), respectively, compared with the control plants (Table 1). Treatment of Pb2+-stressed plants with Mg2+ significantly increased total length, surface area and volume of roots by 27.7% (P = 0.0029), 24.3% (P = 0.0113), and 24.0% (P = 0.0001), respectively, compared with plants treated only with Pb2+ for 60 days.
Effect of Mg2+ on Photosynthetic Pigments and Gas Exchange Parameters of Plants Under Lead Toxicity
Variations in the levels of photosynthetic pigments, including chlorophyll a (Chla), chlorophyll b (Chlb), and carotenoids (Car), were evaluated in T. grandis seedlings under lead toxicity (Table 2). The Chla (P = 0.0001) concentrations, Chlb (P = 0.0001) concentrations, Car (P = 0.0004) concentrations and Chla/Chlb (P = 0.0003) ratios were lower but the Car/Chl(a+b) (P = 0.0003) ratios were higher in the Pb2+-treated plants than in the control plants. Application of Mg2+ resulted in higher Chla (P = 0.0001), Chlb (P = 0.0001), and Car (P = 0.0019) concentrations, and also increased the Chla/b (P = 0.0012) ratios but lowered the Car/Chl(a+b) (P = 0.0005) ratios in the leaves of plants exposed to 1400 mg kg-1 Pb2+ (Table 2). However, significant difference in chlorophyll and carotenoid concentrations between seedlings treated with and without Mg2+ under no Pb2+ toxicity was not found.

TABLE 2. Effects of Mg2+ on chlorophyll a (Chla), chlorophyll b (Chlb), carotenoids (Car), Chl(a+b), chlorophyll a:b ratio (Chla/Chlb), and Car/ Chl(a+b) of T. grandis seedling leaves under Pb2+ toxicity.
Compared with the control, Pb2+ toxicity significantly decreased the photosynthetic rate (Pn), stomatal conductance (Gs) and transpiration (Tr) by 54.6% (P = 0.0001), 39.8% (P = 0.0001), and 58.4% (P = 0.0001), respectively, while it increased intercellular CO2 (Ci) by 49.1% (P = 0.0001) (Figure 3; P < 0.05). In leaves of plants under Pb2+ toxicity, Mg2+ treatment significantly increased the levels of Pn, Gs, and Tr by 91.8% (P = 0.0001), 21.0% (P = 0.0001), and 86.4% (P = 0.0001), respectively, whereas it decreased Ci levels by 21.4% (P = 0.0001) compared with non-Mg2+-treated plants under Pb2+ toxicity (Figure 3).
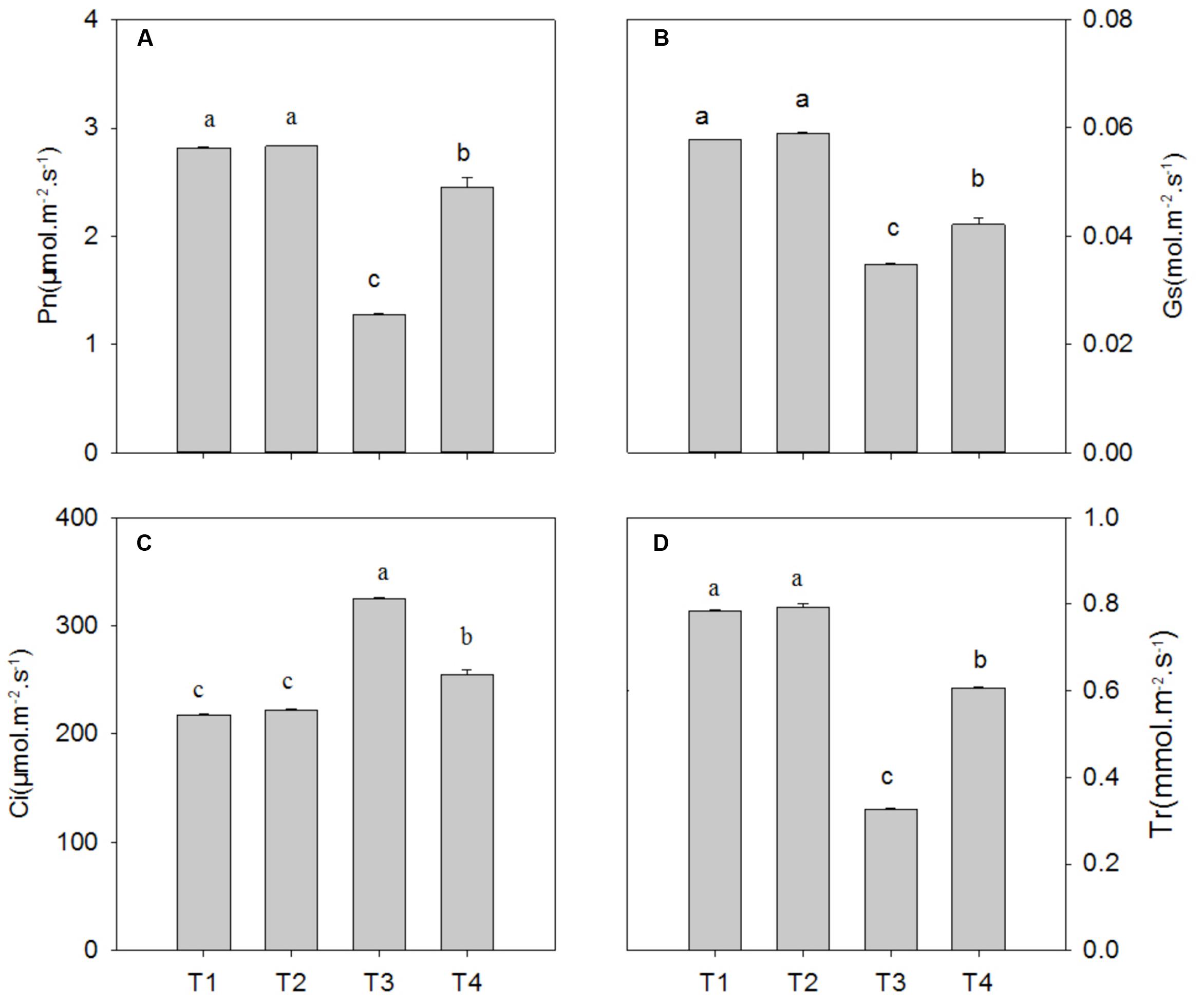
FIGURE 3. The net photosynthetic rate (Pn) (A), stomatal conductance (Gs) (B), internal carbon dioxide concentration (Ci) (C), and transpiration rate (Tr) (D) of T. grandis seedlings grown in media amended with various amounts of Pb2+ and Mg2+. Treatments: T1, control; T2, control + 1040 mg kg-1 Mg2+; T3, 1400 mg kg-1 Pb2+; T4, 1400 mg kg-1 Pb2+ + 1040 mg kg-1 Mg2+. Error bars are standard deviation (n = 3). Different lower case letters above the columns indicate significant (P < 0.05) difference between treatments.
Effect of Mg2+ on Chlorophyll Fluorescence and Oxidative Activity of Roots in Plants Under Lead Toxicity
The Fv/Fm value was significantly decreased by 21.6% (P = 0.0001) in plants under Pb2+ toxicity compared with the control (Figure 4B). Application of Mg2+ significantly increased the Fv/Fm value by 23.7% (P = 0.0001)in leaves of seedlings exposed to 1400 mg kg-1 Pb2+ (Figure 4A).
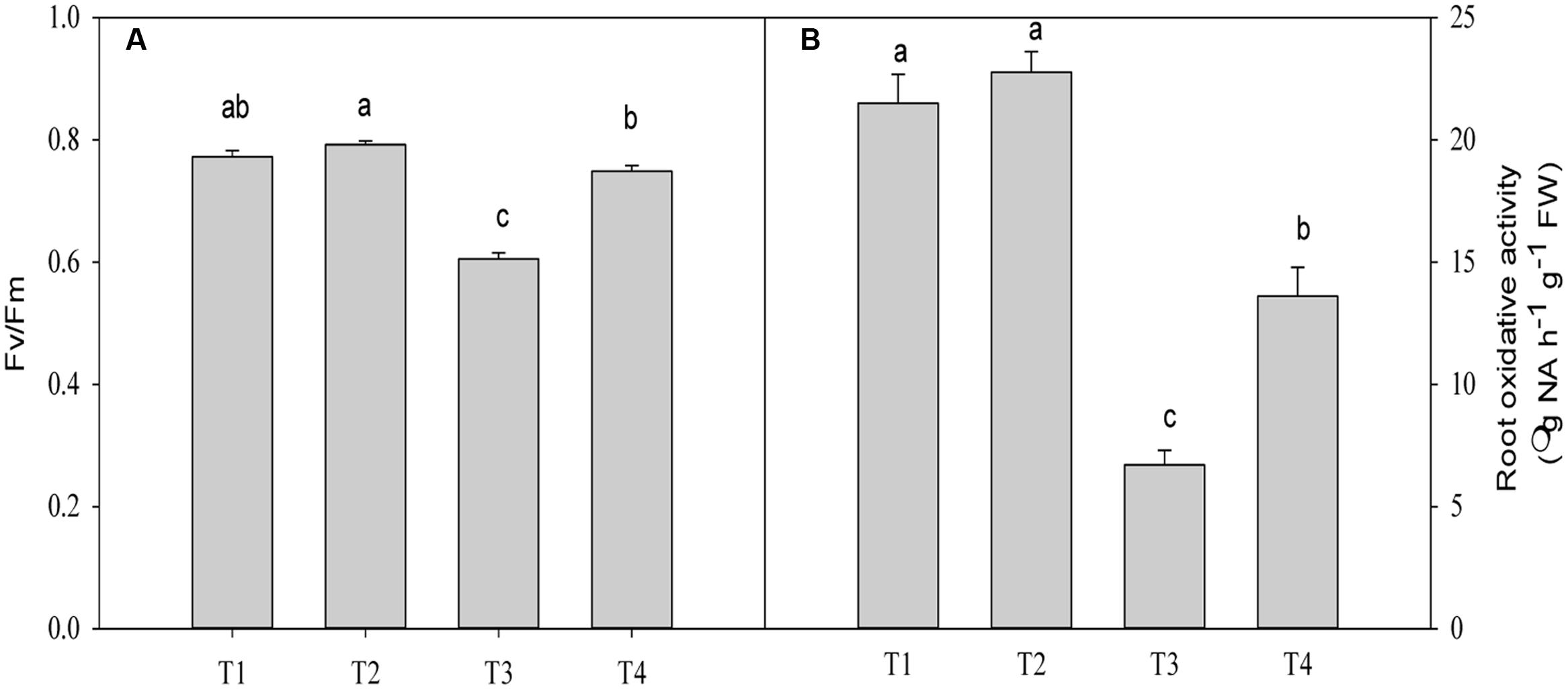
FIGURE 4. The maximum quantum efficiency (Fv/Fm) (A) and root oxidative activity (B) of T. grandis seedlings grown in media amended with various amounts of Pb2+ and Mg2+. Treatments: T1, control; T2, control + 1040 mg kg-1 Mg2+; T3, 1400 mg kg-1 Pb2+; T4, 1400 mg kg-1 Pb2+ + 1040 mg kg-1 Mg2+. Error bars are standard deviation (n = 3). Different lower case letters above the columns indicate significant (P < 0.05) difference between treatments.
The root oxidative activity in plants under Pb2+ toxicity decreased compared with the control (P = 0.0001, Figure 4B). Application of Mg2+ to Pb2+-stressed plants significantly increased root oxidative activity by 102.9% (P = 0.0001) compared with plants only treated with Pb2+ (Figure 4A). However, significant difference in oxidative activity between plants treated with and without Mg under no Pb2+ toxicity was not found (P = 0.4361, Figure 4B).
Effect of Mg2+ on Pb2+ and Mg2+ Accumulation in Plant Tissues Under Lead Toxicity
Under Pb2+ toxicity, Pb2+ contents in the roots were three times higher than in the shoots, indicating that the roots accumulated the majority of the absorbed Pb2+. After application of Mg2+, the Pb2+ concentrations in the roots were higher than in roots of the Pb2+-stressed plants, whereas the Pb2+ uptake in the above-ground parts was lower compared to the plants without Mg2+ application. Interestingly, the distribution of Mg2+ in roots and shoots of the T. grandis seedlings differed significantly. The Mg2+ concentration in the shoots of the control plants was higher than in the roots. However, Pb2+ toxicity had no significant effect on the distribution of Mg2+ between roots (P = 0.6412) and shoots (P = 0.8785) compared with the control. Application of Mg2+ significantly increased Mg2+ accumulation in the shoots (P = 0.0001) and roots (P = 0.0002), and Mg2+ concentration was four times higher in the shoots than in the roots (Table 3).
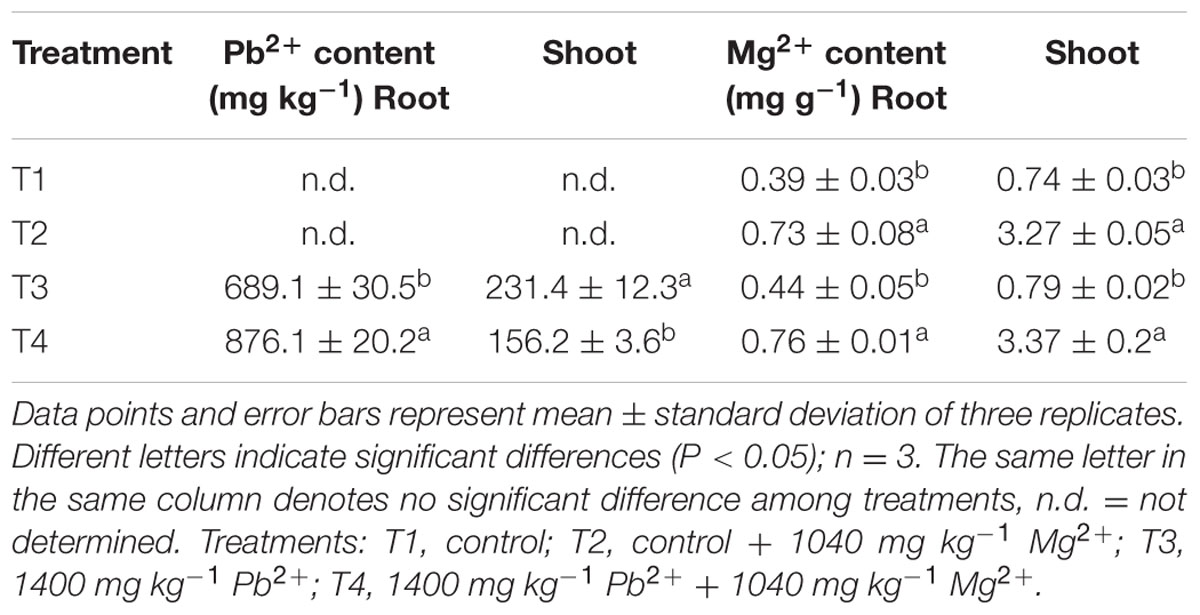
TABLE 3. Effects of Mg2+ on concentrations of Pb2+ and Mg2+ in shoots and roots of T. grandis seedlings grown under Pb2+ toxicity.
Effect of Mg2+ on Ultrastructural Modifications of Leaves and Roots in Plants Under Lead Toxicity
Application of Mg2+ caused significant differences in the ultrastructure of the chloroplasts of the T. grandis seedlings grown under Pb2+ toxicity (Figure 5). Elliptical-shaped chloroplasts with thylakoids were found in the control plants. However, the integrity of the ultrastructure was severely affected by Pb2+ toxicity. Chloroplasts were swollen and had irregularly shaped grana, decreased lamellae, and increased osmiophilic granule numbers, and the thylakoid membrane system in plants was in disorder. Interestingly, application of Mg2+ promoted the development of chloroplasts, grana and stroma lamellae as well as reduced the osmiophilic granule numbers.
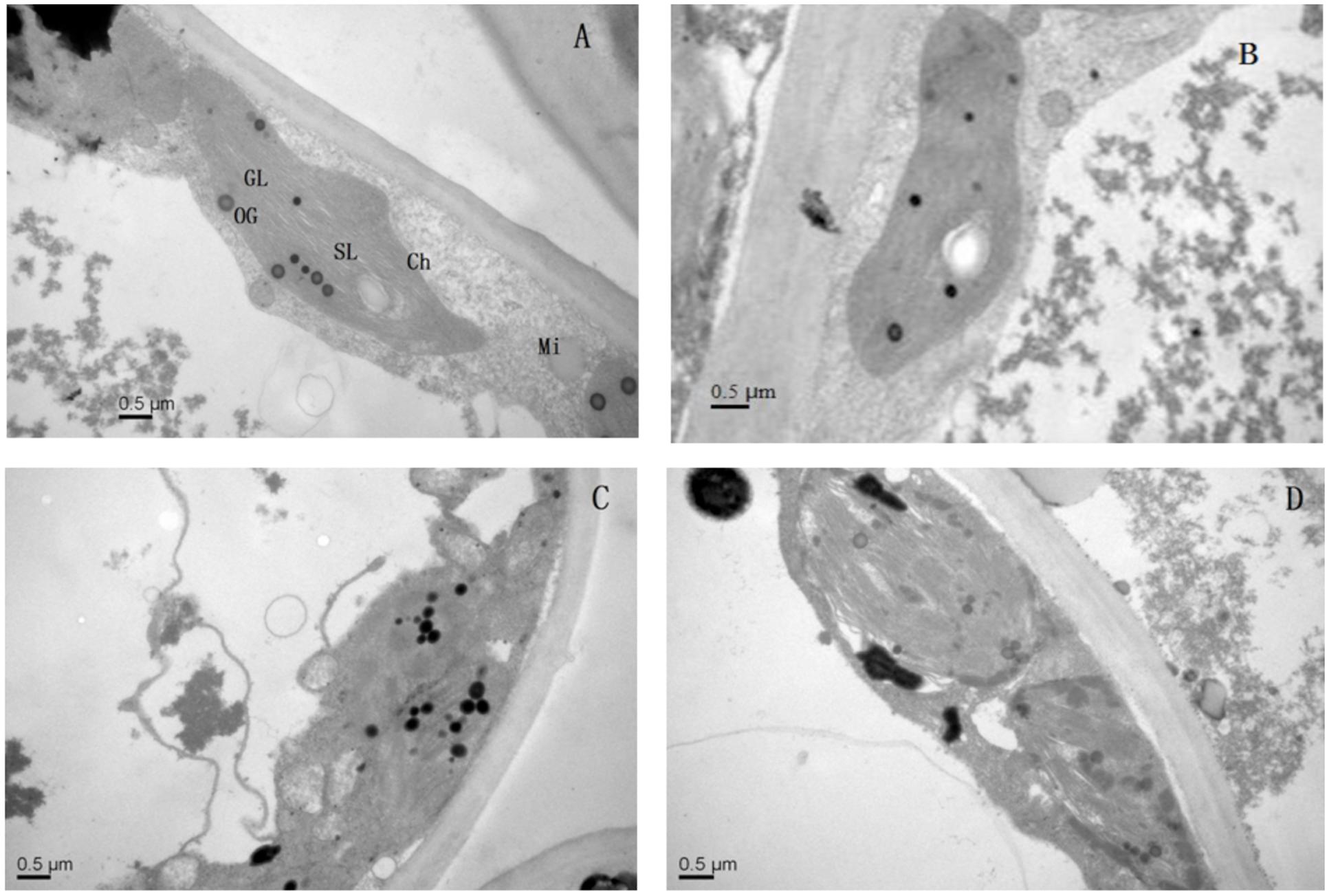
FIGURE 5. The transmission electron micrographs of chloroplasts in T. grandis seedlings grown in media amended with various amounts of Pb2+ and Mg2+. (A) Control; (B) control + 1040 mg kg-1 Mg2+; (C) 1400 mg kg-1 Pb2+; (D) 1400 mg kg-1 Pb2+ + 1040 mg kg-1 Mg2+.
Lead toxicity had a marked influence on the ultrastructure of the seedlings’ roots (Figure 6). Compared with the control, the root cell structure under lead toxicity was completely destroyed. The nucleus was almost invisible and the mitochondria appeared as hollow bubbles. Application of Mg2+ protected the integrity of the root cells as evidenced by visible nuclei, slightly condensed chromatin and irregularly swollen mitochondria with fractured and fuzzy cristae.
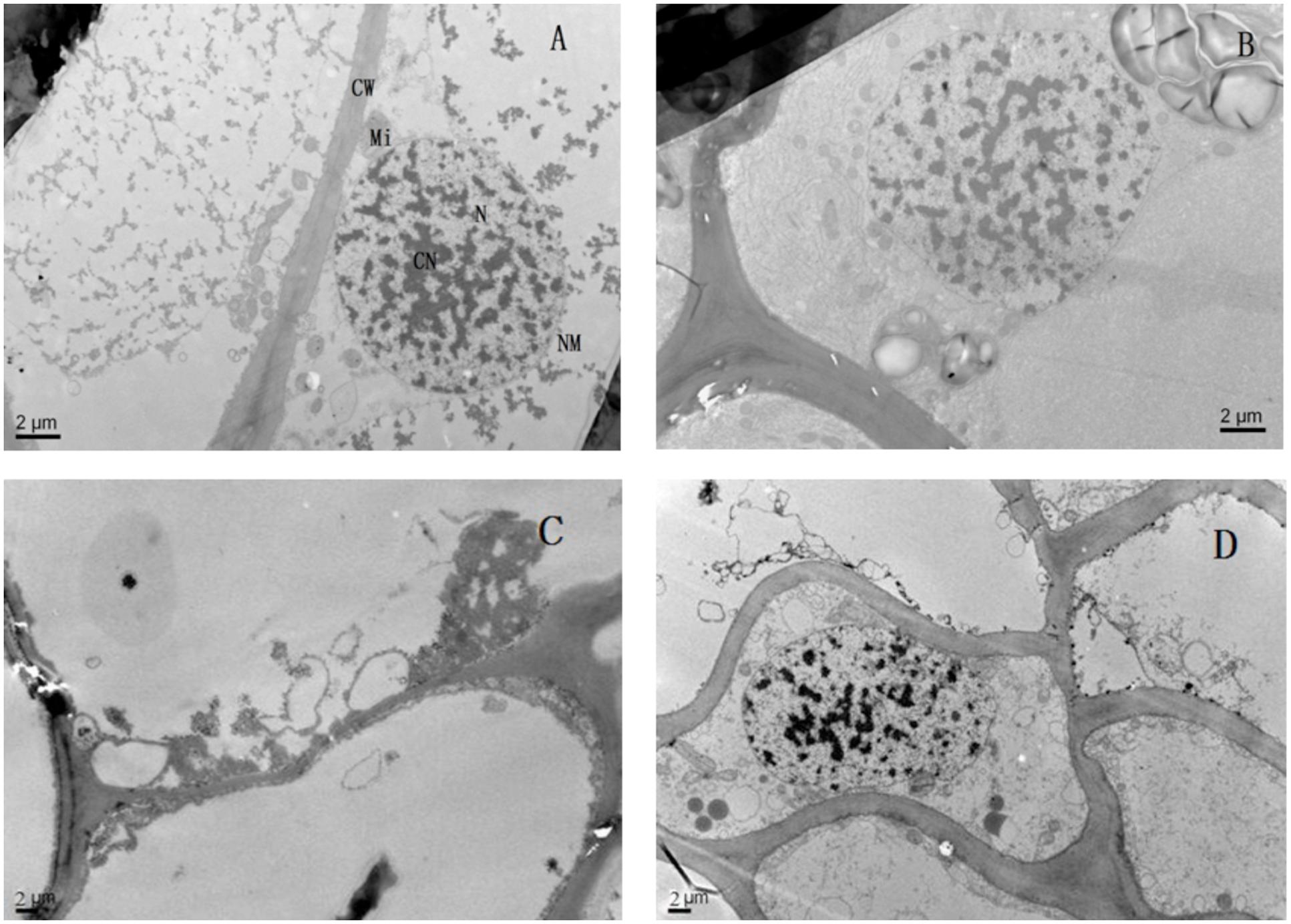
FIGURE 6. The transmission electron micrographs of root of T. grandis seedlings grown in media amended with various amounts of Pb2+ and Mg2+. (A) Control; (B) control + 1040 mg kg-1 Mg2+; (C) 1400 mg kg-1 Pb2+; (D) 1400 mg kg-1 Pb2+ + 1040 mg kg-1 Mg2+.
Discussion
The growth of T. grandis seedlings was highest at 700 mg kg-1 Pb2+ in soil, whereas the lowest growth of plants was found at 1400 mg kg-1 Pb2+ (Figures 1 and 2), indicating that lead stress toxicity in T. grandis seedlings did not occur at 700 mg kg-1 Pb2+ in soil. Meanwhile, visible toxic symptoms, such as old leaves yellowing and chlorosis, were observed in plants exposed to 1400 mg kg-1 Pb2+ (Figure 1). The treatment with 1400 mg kg-1 Pb2+ significantly decreased plant growth and the development of the T. grandis seedlings, as indicated by the decreased shoot dry mass, root dry mass, seedling height and leaf area (Table 1). Similarly, Hadi et al. (2010) found that 500 mg kg-1 Pb2+ in soil did not affect the germination rate of maize (Zea mays) seeds and that the young maize seedlings did not exhibit any visible toxic symptoms.
Chlorophyll fluorescence is the focus in studies of photosynthetic regulation and plant responses to the environment due to its sensitivity, convenience and non-destructive characteristics (Dai et al., 2009). Generally, plants subjected to heavy metal stress typically have lower Fv/Fm values than non-stressed plants, which is associated with photoinhibition of PSII (Krause and Weis, 1991; Wu et al., 2014). In the present study, the Fv/Fm ratio was significantly reduced in the plants treated with 1400 mg kg-1 Pb2+ (Figure 4A). Indeed, this result was consistent with the gas exchange results, in which lead toxicity decreased Pn, Tr and Gs compared with the control (Figure 3), indicating photoinhibition of the photosynthetic capacity in T. grandis seedlings under lead stress conditions. A similar result was also described by Rashid and Popovic (1990) for spinach leaves treated with 2 mM Pb2+. Interestingly, in this study the Ci value was higher in Pb2+-treated plants than in the control plants, indicating that the reduction of photosynthesis under lead stress conditions primarily resulted from non-stomatal limitations. Additionally, photoinhibition and reduction of the photosynthetic capacity under lead stress conditions were manifested by changes of leaf chlorophyll contents. This was explained by its important role in photosynthesis and plant growth. In the present study, we found a strong reduction in the levels of Chla, Chlb, total Chl content and carotenoids in plants treated with 1400 mg kg-1 Pb2+ (Table 2), which was consistent with the results of de Souza et al. (2012), who reported that Pb2+ exposure led to a reduction of Chla and Chlb contents in leaves as well as a reduction of Car levels. Application of Mg2+ to Pb2+-stressed plants improved plant growth, which was accompanied with increased chlorophyll contents, Pn levels and Fv/Fm ratios (Tables 1 and 2; Figures 3 and 4A). Thus, the positive and beneficial effects of Mg2+ on the growth of T. grandis seedlings might be associated with improving the photosynthetic capacity and alleviating photoinhibition. A similar result was reported by Hermans et al. (2011), who indicated that the protective effect of Mg2+ against Cd toxicity may be at least partly attributed to the protection of the photosynthetic apparatus. It is well known that photoinhibition primarily results from overproduction of reactive oxygen species (ROS) through the photosynthetic electron transport chain under stress circumstances (Critchley, 1981). However, it needs to be further elucidated if Mg2+ protects the photosynthetic membrane from photo-oxidation by effectively scavenging ROS under lead stress conditions.
The influence of heavy metal on cellular organization is important for understanding physiological alterations under stress conditions (Souza et al., 2005). In the present study, chloroplasts were highly susceptible to stress induced by high lead conditions, as indicated by decreased lamellae, increased numbers of osmiophilic granules and disrupted thylakoid membranes (Figure 5). Damage to chloroplasts and thylakoid membranes in plants treated with heavy metals has been reported by Wu et al. (2014). We found that Mg2+ ameliorated the chloroplast ultrastructural disorders caused by lead (Figure 5D), which might explain the improved photosynthesis of the Mg-treated plants. Meanwhile, as also suggested by our study, the main processes underlying the improvement in plant photosynthesis induced by Mg2+ treatment are the enhanced light-use-efficiency and the protection of the chloroplast structures.
Many scientists have reported that Mg2+ supplementation enhances the tolerance to toxic metals by reducing the uptake and translocation of metals, including Cd2+ and Al3+ (Kashem and Kawai, 2007; Bose et al., 2011). We found that the accumulation of lead was greater in the roots than in the shoots of T. grandis seedlings (Table 3), indicating that the plants translocated lower concentrations of metals into the shoots (Yang et al., 1998). Higher Pb2+ concentrations in roots than shoots were observed in the Mg2+-alleviated plants, whereas the shoot Mg2+ concentrations were four-fold higher than the root Mg2+ concentrations in the Mg2+-alleviated plants (Table 3). These results suggest that Mg2+ application through the watering solution helped decrease the Pb2+ accumulation in the shoots. Similar findings have been reported by Kashem and Kawai (2007), who found that magnesium-alleviated plants showed decreased shoot Cd2+ concentration in Japanese mustard spinach (Brassica rapa L. var. pervirdis).
Furthermore, lead uptake significantly reduced total root length, surface area and volume compared with the control plants (Table 1). However, the application of Mg2+ increased the indices of root morphological traits of T. grandis seedlings under lead toxicity. Root oxidative activity implies the degree of root development and metabolic status (Liu et al., 2008). In the present study, lower root oxidative activity was found in T. grandis seedlings under Pb2+ toxicity than in the non-Pb2+-treated control plants, whereas higher root oxidative activity was observed in the Mg2+-alleviated plants than in the Pb2+-toxic plants (Figure 4B). These findings indicate that additional Mg2+ might increase the absorptive area of roots and, hence, increase the uptake of water and nutrients to improve plant growth (Glinka, 1980). This finding was consistent with the observed ultrastructure of the T. grandis seedlings roots. The Mg2+ application protected the integrity of the root cells, resulting in a visible nucleus, slightly condensed chromatin and irregularly swollen mitochondria with fractured and fuzzy cristae (Figure 6). Thus, Mg2+ application is an effective method to alleviate Pb2+ toxicity in T. grandis seedlings by improving root growth and root oxidative activity and protecting root ultrastructure.
Conclusion
Torreya grandis seedlings exposed to 1400 mg kg-1 Pb2+ exhibited stress toxicity as indicated by reduced shoot growth. Mg2+ addition under Pb2+ stress conditions might have beneficial effects on the growth of T. grandis seedlings, as evidenced by increased shoot dry biomass, root dry biomass, chlorophyll contents, and photosynthesis as well as improved chloroplast ultrastructure. Moreover, additional Mg2+ in the solution containing Pb2+ decreased the Pb2+ concentration in the shoots and increased the Mg2+ concentration in the shoots. Furthermore, we showed that the positive effects of Mg2+ on the growth of T. grandis were triggered by protecting the morphology, activity and ultrastructure of the roots. To our knowledge, this study is the first study to show Mg2+-induced alleviation of lead toxicity in T. grandis seedlings and is of great importance for the cultivation of T. grandis seedlings in China, where soils are often contaminated with lead.
Author Contributions
All authors listed, have made substantial, direct and intellectual contribution to the work, and approved it for publication.
Conflict of Interest Statement
The authors declare that the research was conducted in the absence of any commercial or financial relationships that could be construed as a potential conflict of interest.
Acknowledgment
This work was financially supported by the Special Fund for Forest Scientific Research in the Public Welfare (201504708).
Footnotes
References
Bose, J., Babourina, O., and Rengel, Z. (2011). Role of magnesium in alleviation of aluminium toxicity in plants. J. Exp. Bot. 62, 2251–2264. doi: 10.1093/jxb/erq456
Buekers, J., Redeker, E. S., and Smolders, E. (2009). Lead toxicity to wildlife: derivation of a critical blood concentration for wildlife monitoring based on literature data. Sci. Total Environ. 407, 3431–3438. doi: 10.1016/j.scitotenv.2009.01.044
Chen, G. J., Shi, H. B., Tao, J. S., Chen, L., Liu, Y. Y., Lei, G. L., et al. (2015). Industrial arsenic contamination causes catastrophic changes in freshwater ecosystems. Sci. Rep. 5:17419. doi: 10.1038/srep17419
Critchley, C. (1981). Studies on the mechanism of photoinhibition in higher plants: I. Effects of high light intensity on chloroplast activities in cucumber adapted to low light. Plant Physiol. 67, 1161–1165. doi: 10.1104/pp.67.6.1161
Dai, Y., Shen, Z., Liu, Y., Wang, L., Hannaway, D., and Lu, H. (2009). Effects of shade treatments on the photosynthetic capacity, chlorophyll fluorescence, and chlorophyll content of Tetrastigma hemsleyanum Diels et Gilg. Environ. Exp. Bot. 65, 177–182. doi: 10.1016/j.envexpbot.2008.12.008
de Souza, S. C. R., de Andrade, S. A. L., de Souza, L. A., and Schiavinato, M. A. (2012). Lead tolerance and phytoremediation potential of Brazilian leguminous tree species at the seedling stage. J. Environ. Manage. 110, 299–307. doi: 10.1016/j.jenvman.2012.06.015
Eide, D., Broderius, M., Fett, J., and Guerinot, M. L. (1996). A novel iron-regulated metal transporter from plants identified by functional expression in yeast. Proc. Natl. Acad. Sci. U.S.A. 93, 5624–5628. doi: 10.1073/pnas.93.11.5624
Glinka, Z. (1980). Abscisic acid promotes both volume flow and ion release to the xylem in sunflower roots. Plant Physiol. 65, 537–540. doi: 10.1104/pp.65.3.537
Guerinot, M. L. (2000). The ZIP family of metal transporters. Biochim. Biophys. Acta 1465, 190–198. doi: 10.1016/S0005-2736(00)00138-3
Gupta, D. K., Huang, H. G., Yang, X. E., Razafindrabe, B. H. N., and Inouhe, M. (2010). The detoxification of lead in Sedum alfredii H. is not related to phytochelatins but the glutathione. J. Hazard. Mater. 177, 437–444. doi: 10.1016/j.jhazmat.2009.12.052
Gupta, D. K., Nicoloso, F. T., Schetinger, M. R. C., Rossato, L. V., Pereira, L. B., Castro, G. Y., et al. (2009). Antioxidant defense mechanism in hydroponically grown Zea mays seedlings under moderate lead stress. J. Hazard. Mater. 172, 479–484. doi: 10.1016/j.jhazmat.2009.06.141
Hadi, F., Bano, A., and Fuller, M. P. (2010). The improved phytoextraction of lead (Pb2+) and the growth of maize (Zea mays L.): the role of plant growth regulators (GA3 and IAA) and EDTA alone and in combinations. Chemosphere 80, 457–462. doi: 10.1016/j.chemosphere.2010.04.020
Hermans, C., Chen, J., Coppens, F., Inzé, D., and Verbruggen, N. (2011). Low magnesium status in plants enhances tolerance to cadmium exposure. New Phytol. 192, 428–436. doi: 10.1111/j.1469-8137.2011.03814.x
Hu, J., Shi, G., Xu, Q., Wang, X., Yuan, Q., and Du, K. (2007). Effects of Pb2+ on the active oxygen-scavenging enzymes activities and ultrastructure in Potamogeton crispus leaves. Russ. J. Plant Physiol. 54, 414–419. doi: 10.1134/S1021443707030181
Huang, G. P., Liu, Y. Q., and Cheng, L. (2006). Effect of Pb2+ pollution on physiological characteristics of Pinus rigida. Acta Agric. Univ. Jiangxiensis 28, 833–837.
Huang, J. W., and Cunningham, S. D. (1996). Lead phytoextraction: species variation in lead uptake and translocation. New Phytol. 134, 75–84. doi: 10.1111/j.1469-8137.1996.tb01147.x
Huang, Y., Wang, J., Li, G., Zheng, Z., and Su, W. (2001). Antitumor and antifungal activities in endophytic fungi isolated from pharmaceutical plants Taxus mairei, Cephalotaxus fortunei and Torreya grandis. FEMS Immunol. Med. Microbiol. 31, 163–167. doi: 10.1111/j.1574-695X.2001.tb00513.x
Hurlbert, S. H. (1984). Pseudoreplication and the design of ecological field experiments. Ecol. Monogr. 54, 187–211. doi: 10.2307/1942661
Kashem, M. D. A., and Kawai, S. (2007). Alleviation of cadmium phytotoxicity by magnesium in Japanese mustard spinach. Soil Sci. Plant Nutr. 53, 246–251. doi: 10.1111/j.1747-0765.2007.00129.x
Kaur, G., Kaur, S., Singh, H. P., Batish, D. R., Kohli, R. K., and Rishi, V. (2015). Biochemical adaptation in Zea mays root to short-term Pb2+ exposure: ROS generation and metabolism. Bull. Environ. Contam. Toxicol. 95, 246–253. doi: 10.1007/s00128-015-1564-y
Korshunova, Y. O., Eide, D., Clark, W. G., Guerinot, M. L., and Pakrasi, H. B. (1999). The IRT1 protein from Arabidopsis thaliana is a metal transporter with a broad substrate range. Plant Mol. Biol. 40, 37–44. doi: 10.1023/A:1026438615520
Krabbenhoft, D. P., and Sunderland, E. M. (2013). Global change and mercury. Science 341, 1457–1458. doi: 10.1126/science.1242838
Krause, G. H., and Weis, E. (1991). Chlorophyll fluorescence and photosynthesis: the basics. Annu. Rev. Plant Biol. 42, 313–349. doi: 10.1146/annurev.pp.42.060191.001525
Lamhamdi, M., Bakrim, A., Aarab, A., Lafont, R., and Sayah, F. (2011). Lead phytotoxicity on wheat (Triticum aestivum L.) seed germination and seedling growth. C. R. Biol. 334, 118–126. doi: 10.1016/j.ecoenv.2010.08.041
Lichtenthaler, H. K. (1987). Chlorophylls and carotenoids – pigments of photosynthetic biomembranes. Methods Enzymol. 148, 350–382. doi: 10.1016/0076-6879(87)48036-1
Liu, D. H., Jiang, W., Liu, C., Xin, C., and Hou, W. (2000). Uptake and accumulation of lead by roots, hypocotyls and shoots of Indian mustard [Brassica juncea (L.)]. Bioresour. Technol. 71, 273–277. doi: 10.1016/S0960-8524(99)00082-6
Liu, R. X., Zhou, Z. G., Guo, W. Q., Chen, B. L., and Oosterhuis, D. M. (2008). Effects of N fertilization on root development and activity of water-stressed cotton (Gossypium hirsutum L.) plants. Agric. Water Manage. 95, 1261–1270. doi: 10.1016/S0960-8524(99)00082-6
Maherali, H., and DeLucia, E. H. (2000). Interactive effects of elevated CO2 and temperature on water transport in ponderosa pine. Am. J. Bot. 87, 243–249. doi: 10.2307/2656912
Maxwell, K., and Johnson, G. N. (2000). Chlorophyll fluorescence – a practical guide. J. Exp. Bot. 51, 659–668. doi: 10.1093/jexbot/51.345.659
Mishra, A. (2012). Intermittent irrigation enhances morphological and physiological efficiency of rice plants. Agriculture 58, 121–130. doi: 10.2478/v10207-012-0013-8
Pittman, J. K. (2005). Managing the manganese: molecular mechanisms of manganese transport and homeostasis. New Phytol. 167, 733–742. doi: 10.1111/j.1469-8137.2005.01453.x
Pourrut, B., Shahid, M., Dumat, C., Winterton, P., and Pinelli, E. (2011). Lead uptake, toxicity, and detoxification in plants. Rev. Environ. Contam. Toxicol. 213, 113–136. doi: 10.1007/978-1-4419-9860-6_4
Rashid, A., and Popovic, R. (1990). Protective role of CaCl2 against Pb2+ inhibition in photosystem II. FEBS Lett. 2711, 181–184. doi: 10.1016/0014-5793(90)80401-4
Sharma, P., and Dubey, R. S. (2005). Lead toxicity in plants. Braz. J. Plant Physiol. 17, 35–52. doi: 10.1590/S1677-04202005000100004
Souza, J. F., Dolder, H., and Cortelazzo, A. L. (2005). Effect of excess cadmium and zinc ions on roots and shoots of maize seedlings. J. Plant Nutr. 28, 1923–1931. doi: 10.1080/01904160500310435
Tang, H., Hu, Y. Y., Yu, W. W., Song, L. L., and Wu, J. S. (2015). Growth, photosynthetic and physiological responses of Torreya grandis seedlings to varies light environments. Trees 29, 1011–1022. doi: 10.1007/s00468-015-1180-9
Thomine, S., Wang, R., Ward, J. M., Crawford, N. M., and Schroeder, J. I. (2000). Cadmium and iron transport by members of a plant metal transporter family in Arabidopsis with homology to Nramp genes. Proc. Natl. Acad. Sci. U.S.A. 97, 4991–4996. doi: 10.1073/pnas.97.9.4991
Wu, M., Wang, P. Y., Sun, L. G., Zhang, J. J., Yu, J., Wang, Y. W., et al. (2014). Alleviation of cadmium toxicity by cerium in rice seedlings is related to improved photosynthesis, elevated antioxidant enzymes and decreased oxidative stress. Plant Growth Regul. 74, 251–260. doi: 10.1007/s10725-014-9916-x
Yang, M. G., Lin, X. Y., and Yang, X. E. (1998). Impact of Cd on growth and nutrient accumulation of different plant species. Chin. J. Appl. Ecol. 19, 89–94.
Keywords: Torreya grandis, lead toxicity, magnesium, heavy metal phytotoxicity, phytoremediation
Citation: Shen J, Song L, Müller K, Hu Y, Song Y, Yu W, Wang H and Wu J (2016) Magnesium Alleviates Adverse Effects of Lead on Growth, Photosynthesis, and Ultrastructural Alterations of Torreya grandis Seedlings. Front. Plant Sci. 7:1819. doi: 10.3389/fpls.2016.01819
Received: 30 September 2016; Accepted: 18 November 2016;
Published: 30 November 2016.
Edited by:
Jian-Guo Huang, University of Chinese Academy of Sciences, ChinaReviewed by:
Anitha Kunhikrishnan, National Institute of Agricultural Science, South KoreaLei Chen, Hokkaido University, Japan
Copyright © 2016 Shen, Song, Müller, Hu, Song, Yu, Wang and Wu. This is an open-access article distributed under the terms of the Creative Commons Attribution License (CC BY). The use, distribution or reproduction in other forums is permitted, provided the original author(s) or licensor are credited and that the original publication in this journal is cited, in accordance with accepted academic practice. No use, distribution or reproduction is permitted which does not comply with these terms.
*Correspondence: Jiasheng Wu, d3Vqc0B6YWZ1LmVkdS5jbg==
†These authors have contributed equally to this work.