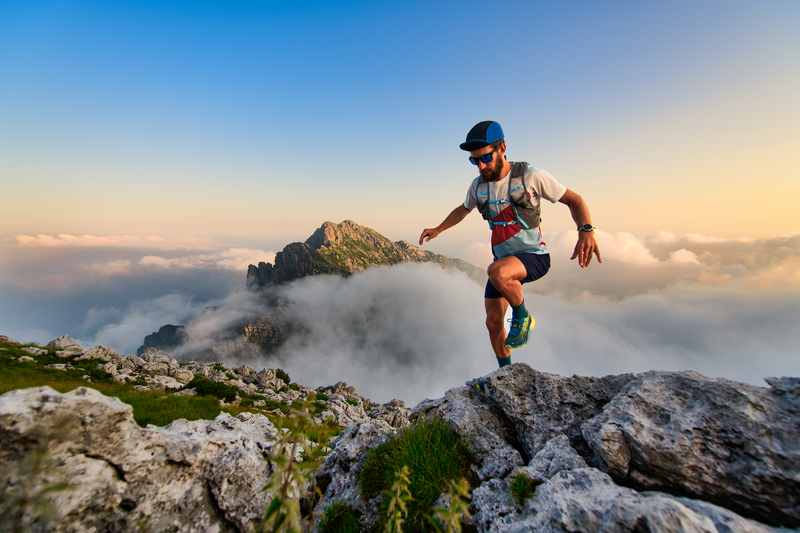
95% of researchers rate our articles as excellent or good
Learn more about the work of our research integrity team to safeguard the quality of each article we publish.
Find out more
REVIEW article
Front. Plant Sci. , 29 August 2016
Sec. Plant Physiology
Volume 7 - 2016 | https://doi.org/10.3389/fpls.2016.01296
This article is part of the Research Topic Roots – the hidden provider View all 17 articles
Roots are subjected to a range of abiotic stresses as they forage for water and nutrients. Cytosolic free calcium is a common second messenger in the signaling of abiotic stress. In addition, roots take up calcium both as a nutrient and to stimulate exocytosis in growth. For calcium to fulfill its multiple roles must require strict spatio-temporal regulation of its uptake and efflux across the plasma membrane, its buffering in the cytosol and its sequestration or release from internal stores. This prompts the question of how specificity of signaling output can be achieved against the background of calcium’s other uses. Threats to agriculture such as salinity, water availability and hypoxia are signaled through calcium. Nutrient deficiency is also emerging as a stress that is signaled through cytosolic free calcium, with progress in potassium, nitrate and boron deficiency signaling now being made. Heavy metals have the capacity to trigger or modulate root calcium signaling depending on their dose and their capacity to catalyze production of hydroxyl radicals. Mechanical stress and cold stress can both trigger an increase in root cytosolic free calcium, with the possibility of membrane deformation playing a part in initiating the calcium signal. This review addresses progress in identifying the calcium transporting proteins (particularly channels such as annexins and cyclic nucleotide-gated channels) that effect stress-induced calcium increases in roots and explores links to reactive oxygen species, lipid signaling, and the unfolded protein response.
Plant roots are exposed to a variety of abiotic stresses as they navigate the soil, foraging for nutrients and water. Cytosolic free calcium ([Ca2+]cyt) is central to the response to these stresses, acting as a second messenger but also driving exocytosis (Carroll et al., 1998). Specificity of [Ca2+]cyt signaling is determined by the amplitude and duration (and possible oscillation) of the [Ca2+]cyt increase, often referred to as the “signature”’ (McAinsh and Pittman, 2009), that is elicited by the stimulus. This signature would be driven by the opening of plasma membrane (PM) and endomembrane Ca2+-permeable channels and terminated by the activity of Ca2+ efflux transporters in those membranes, plus Ca2+-binding proteins, to restore the resting [Ca2+]cyt of 100–200 nM. Use of organelle-targeted Ca2+ reporting proteins has shown that the Ca2+ content of the endoplasmic reticulum (ER) and Golgi increases after stress-induced transient increases in [Ca2+]cyt, strongly suggesting that Ca2+ is sequestered there to terminate the [Ca2+]cyt signal (Ordenes et al., 2012; Bonza et al., 2013). Transport of Ca2+ into organelles is catalyzed by Ca2+-ATPases. There are two distinct families: The Auto-inhibited Ca2+-ATPases, ACA (that also operate at the PM) and the ER Ca2+-ATPases, ECA; reviewed by Bonza et al., 2016). The lower affinity CAX (Cation/H+ Exchangers) appear to be restricted to endomembranes but also facilitate Ca2+ sequestration (Connorton et al., 2012). Changes in organelle free Ca2+ in roots could also play a part in signaling, most notably in the formation of symbioses and cell death (Stael et al., 2012; Zhao et al., 2013; Wagner et al., 2015). Decoding the [Ca2+]cyt signature will be effected by specific Ca2+-binding proteins. Calmodulins (CaMs) and Calmodulin-like proteins (CMLs) are encoded by multi-gene families in plants. They lack kinase domains, suggesting these proteins must target others with enzymatic activity. CaMs modulate transcription by binding to Calmodulin-binding Transcription Activators (CAMTAs) (Virdi et al., 2015). Other multi-gene families are also evident for Ca2+-Dependent Protein Kinases (CPKs) and Calcineurin-B Like proteins (CBLs). The latter target CBL-Interacting Protein Kinases (CIPKs) to effect cellular responses (Thoday-Kennedy et al., 2015). Changes in [Ca2+]cyt also have the potential to activate lipid signaling pathways. A somewhat forgotten aspect of Ca2+ signaling is the Ca2+ activation of members of the Phospholipase C and Phospholipase D families (Qin et al., 1997; Hunt et al., 2004; Dressler et al., 2014; Ruelland et al., 2015; Hou et al., 2016). Phospholipase C catalyses production of diacylglycerol and inositol trisphosphate (InsP3) while Phospholipase D catalyses production of phosphatidic acid, thus [Ca2+]cyt would have the capacity to trigger distinct lipid signals depending on the location and Ca2+-sensitivity of the phospholipases. Targets of lipid signals have been reviewed recently by Hou et al. (2016).
The vast majority of [Ca2+]cyt measurements are from Arabidopsis thaliana seedlings and guard cells, achieved using the luminescent Ca2+-interacting aequorin protein. Far fewer studies have focused specifically on roots or utilized the greater sensitivity and spatial resolution of ratiometric fluorescent dyes. The genetically encoded YC3.6 Ca2+ reporter is now being used for both Arabidopsis and rice roots (Behera et al., 2015), holding much promise for the future. It is now clear that an identical stimulus can elicit markedly different root [Ca2+]cyt signatures depending on genus. So far, rice root [Ca2+]cyt signals have been found to be lower in amplitude but of longer duration than those of Arabidopsis (Behera et al., 2015).
Electrophysiological studies of root cell plasma membrane (PM) have advanced our understanding of the Ca2+ influx routes that could generate [Ca2+]cyt signatures. There is a central role for PM voltage in [Ca2+]cyt signaling, as individual stresses can hyperpolarize (render it more negative) or depolarize (render it less negative). Manipulating PM voltage elicits distinct [Ca2+]cyt signatures and resultant transcriptional responses (Whalley et al., 2011; Whalley and Knight, 2013). Studies on root epidermal and root hair PM have shown that this membrane harbors channels that are activated by hyperpolarized voltage (Hyperpolarization-Activated Ca2+ Channels (HACCs); Véry and Davies, 2000; Demidchik et al., 2002, 2009; Ma et al., 2012), Depolarization-Activated Ca2+ Channels (DACCS); Demidchik et al., 2002; Miedema et al., 2008) and Voltage-Independent Ca2+ Channels (VICCs) (Demidchik et al., 2002). Thus changes in voltage would activate specific suites of channels to generate a signature. An additional tier of regulation of the PM Ca2+ influx routes is afforded by reactive oxygen species (ROS) that are produced during development and stress responses (Figure 1). This regulation depends on the specific ROS, its position, the cell type and the cell’s developmental state. In Arabidopsis roots, sensitivity of PM Ca2+ channel activation by extracellular H2O2 decreases as epidermal cells mature but is still greater than that of the cortex (Demidchik et al., 2007). A similar picture emerges for extracellular hydroxyl radicals, which elicit greater PM Ca2+ influx currents in the epidermis and root hairs than the pericycle (Demidchik et al., 2003; Foreman et al., 2003). In epidermal PM of the elongation zone, extracellular hydroxyl radicals elicit different Ca2+ channel activity to extracellular H2O2 (Demidchik et al., 2003, 2007). Thus, ROS will play a significant part of generating cell-specific [Ca2+]cyt signatures in response to stress.
FIGURE 1. Reactive oxygen species (ROS) regulation of PM Ca2+ channels in the Arabidopsis root elongation zone epidermis. NADPH oxidases (RBOH) generate extracellular superoxide anion that can undergo conversion to H2O2 and hydroxyl radicals (OH⋅) (Richards et al., 2015). H2O2 could activate HACC (orange) at the extracellular PM face or enter the cytosol through aquaporins (blue) to activate at the cytosolic face (directly or indirectly) (Demidchik et al., 2007). Extracellular hydroxyl radicals activate Annexin 1 (pink) (Demidchik et al., 2003; Foreman et al., 2003; Laohavisit et al., 2012). Ca2+ influx would depolarise the PM and if unopposed this could activate DACCs (yellow) (Demidchik et al., 2002). Increased [Ca2+]cyt could further activate RBOH
Stress-induced [Ca2+]cyt elevation in roots remains poorly understood in terms of the genes encoding the PM or endo membrane Ca2+ channels involved. Plants have multi-gene families of Glutamate Receptor-Like channels (GLR; activated by a range of extracellular nitrogenous ligands) and Cyclic Nucleotide-Gated channels (CNGC; activated by intracellular cyclic nucleotides), with each gene encoding a potential subunit of a potentially tetrameric channel. Some members have been characterized as having Ca2+ channel forming ability (reviewed by Swarbreck et al., 2013 and Weiland et al., 2016). Membrane residency has yet to be determined for all proteins and while the majority tested are in the PM, in Arabidopsis GLR3.5 has been localized to both mitochondria and chloroplast, depending on its splicing variant (Teardo et al., 2015), CNGC19 to the vacuole (Yuen and Christopher, 2013) and CNGC20 potentially to both PM and vacuole (Fischer et al., 2013; Yuen and Christopher, 2013). Mechanosensitive Ca2+ channels of the PM have also been identified (Nakagawa et al., 2007; Hou et al., 2014; Yuan et al., 2014; Kamano et al., 2015), as has a vacuolar Ca2+ efflux channel TPC1 (Two Pore Channel1; Peiter et al., 2005). Root cells will express specific complements of these genes and their transcription can change under abiotic stress (Dinneny et al., 2008; Roy et al., 2008), with the implication that stress resets the [Ca2+]cyt signaling system.
The threat of abiotic stress is global. Drought threatens plant productivity across continents, with water shortage not only imposing an osmotic challenge but also leading to soil hardness that roots must overcome. Changing weather patterns are bringing greater rainfalls to some areas (particularly Northern Europe) thus leading to the threat of hypoxic challenge from waterlogged soil (Shabala et al., 2014). Salinity stress arising from sodic soils is made worse by irrigation and counteracting nutritional deprivation by fertilizer application comes with an increasing economic and environmental cost. In this review, the effects of salinity, water availability (including soil hardness), nutritional deprivation, heavy metals and cold on root [Ca2+]cyt will be addressed. The candidate channels for elevating [Ca2+]cyt in roots will be introduced and the downstream consequences of the signal will be reviewed.
The transporters for Na+ influx into the root are not fully known but include the PM cyclic nucleotide-gated channels CNGC3 (Gobert et al., 2006) and CNGC10 (Guo et al., 2008; Jin et al., 2015) in Arabidopsis. Na+ ingress is opposed by the Annexin1 protein and the AGB1 heterotrimeric G protein subunit in Arabidopsis roots (Laohavisit et al., 2013; Yu and Assmann, 2015). The mechanisms for sensing the increase in cytosolic Na+ remain obscure (Maathuis, 2014; Shabala et al., 2015) however what is clear is that Na+ entry depolarizes the root epidermal PM voltage (Maathuis, 2014). This is significant in that it implicates depolarization-activated and voltage-independent PM Ca2+-permeable channels in generating the transient [Ca2+]cyt increases observed in roots in response to NaCl (Figure 2). Both channels types are present in Arabidopsis root epidermal PM (Demidchik et al., 2002). However, involvement of hyperpolarization-activated PM Ca2+ channels should not be dismissed because increasing [Ca2+]cyt shifts their activation voltage to more depolarized values (Véry and Davies, 2000; Demidchik et al., 2002) and they are implicated in the Arabidopsis root response to NaCl (Ma et al., 2012; Laohavisit et al., 2013). Critically, hyperosmotic stress hyperpolarizes root epidermal PM voltage (Maathuis, 2014) and this would potentially be a basis for generating a component of the [Ca2+]cyt signal specific to the hyperosmotic component of NaCl stress.
FIGURE 2. Ca2+ transporters in salinity-stress signaling. Na+ enters root epidermis and depolarises the PM, possibly activating DACCs (yellow) with the [Ca2+]cyt increase also possibly activating HACCs (orange). Na+ sensing results in cGMP/cAMP production that could activate CNGC HACCs (Shabala et al., 2015). InsP3 and cADPR production causes Ca2+ release from stores by unknown channels (DeWald et al., 2001; Tracy et al., 2008; Zhang Y. et al., 2015; Zhang X. et al., 2015). ARP2/3 restricts mitochondrial Ca2+ efflux (Zhao et al., 2013). [Ca2+]cyt activation of RBOH NADPH oxidase activity would promote extracellular hydroxyl radical formation and activation of Annexin1-mediated Ca2+ influx (pink) (Laohavisit et al., 2013). We hypothesize that these are held in a microdomain (red). This leads to SOS1 transcription (Laohavisit et al., 2013). CaM activation would restrict CNGC-mediated Ca2+ influx and promote efflux by ACA Ca2+-ATPase activation (Boursiac and Harper, 2007). [Ca2+]cyt elevation activates CBL4 and CIPK24 (purple) leading to SOS1-mediated Na+ extrusion (Liu et al., 2000). CIPK24 activates vacuolar V-ATPase to provide the driving force for NHX1 and CAX1 to sequester Na+ and Ca2+ respectively, also activated by CIPK24 (Cheng et al., 2004; Batelli et al., 2007; Quintero et al., 2011).
Application of NaCl can cause a heterogeneous increase in Arabidopsis and rice root [Ca2+]cyt that depends on cell type, external [Ca2+], and the bathing medium’s effect on PM voltage (Kiegle et al., 2000; Tracy et al., 2008; Laohavisit et al., 2013; Choi et al., 2014; Zhang Y. et al., 2015). Pericycle cells of Arabidopsis have a lower amplitude of [Ca2+]cyt increase and a more variable recovery phase than the surrounding tissues (Kiegle et al., 2000), suggesting a cell-specific transporter complement. Block of NaCl-induced [Ca2+]cyt increase by lanthanides implicates PM Ca2+ influx channels in the response of both Arabidopsis and rice roots (Tracy et al., 2008; Zhang Y. et al., 2015). The anti-apoptotic protein Bcl-2 mimics lanthanides in that its overexpression impairs the NaCl-induced [Ca2+]cyt increase in rice roots (Kim et al., 2014). Clearly, the [Ca2+]cyt increase could lead to cell death if it were great enough and Bcl-2 may interact directly with PM channels. The genetic identities of the PM Ca2+ influx channels initiating the NaCl-induced [Ca2+]cyt increase remain unknown. It has been suggested that they may be Ca2+-permeable CNGCs (Shabala et al., 2015) as salinity stress increased cytosolic cGMP in Arabidopsis seedlings within seconds (Donaldson et al., 2004). The source of cGMP may prove to be critical as only the [Ca2+]cyt response to low NaCl (50 mM) was sensitive to inhibition of soluble guanylyl cyclase activity and the signal evoked by an equivalent osmotic stress was insensitive (Donaldson et al., 2004). It remains feasible that neither the ionic nor osmotic components of the [Ca2+]cyt increase in response to high [NaCl] require cGMP. The osmotic component of NaCl stress could increase [Ca2+]cyt in Arabidopsis roots through the PM mechanosensitive Ca2+-permeable channel OSCA1 (Reduced Hyperosmolality-Induced [Ca2+]I increase1). This channel mediates the [Ca2+]cyt response to hyperosmotic stress (Yuan et al., 2014). Its discovery through a screen of aequorin-expressing mutants (Yuan et al., 2014) highlights the potential of this approach for identification of channels (and their families) involved in stress-induced [Ca2+]cyt elevation.
The initial increase in [Ca2+]cyt could be amplified by the production of ROS sourced ultimately by PM NADPH oxidases (encoded by Respiratory Burst Oxidase Homolog genes), with [Ca2+]cyt activating these enzymes through their EF hands (Figure 2). In accordance with this, Arabidopsis root cortical cells lacking RBOHD and F have much lower PM hyperpolarization-activated Ca2+ activity in response to NaCl challenge than wild type (Ma et al., 2012). Mutant seedlings have an impaired [Ca2+]cyt response. Arabidopsis RBOHD can be activated by CIPK26/CBL1/9 (Drerup et al., 2013) but a direct link to stress has not been shown. Extracellular hydroxyl radicals are likely to be the ROS involved in [Ca2+]cyt elevation (Chung et al., 2008; Demidchik et al., 2010; Laohavisit et al., 2013; Richards et al., 2015). The extracellular superoxide anions produced by NADPH oxidases are readily converted to H2O2 and wall Fe/Cu act as Fenton catalysts to generate hydroxyl radicals (Richards et al., 2015). Their production by Arabidopsis roots is significantly increased under NaCl stress (Demidchik et al., 2010) and RBOHC is implicated as a driver for production (Chung et al., 2008). Extracellular hydroxyl radicals activate a PM Ca2+ influx in Arabidopsis root epidermis (Demidchik et al., 2003; Foreman et al., 2003) that has now been shown to be mediated by Annexin1 (Laohavisit et al., 2012).
Annexins are Ca2+-binding proteins that can bind to or insert into membranes and are implicated in stress reactions (Laohavisit and Davies, 2011; Davies, 2014). Critically, when root epidermal protoplasts are challenged with NaCl, the resultant PM hyperpolarization-activated Ca2+ influx is lost in the annexin1 loss of function mutant and the [Ca2+]cyt signal is impaired (Laohavisit et al., 2013). Hydroxyl radicals are potent but short-lived so their effects must be close to the site of production (Richards et al., 2015). As NADPH oxidases can be held in lipid rafts it may be that hydroxyl radicals target co-resident channels. Finally, extracellular ATP levels of Arabidopsis roots increase in response to NaCl (Dark et al., 2011). Extracellular ATP activates root epidermal PM hyperpolarization-activated Ca2+ influx channels via RBOHC (Demidchik et al., 2009), suggesting the involvement of extracellular ROS in channel activation. Whether these channels involve Annexin1 remains to be determined but both the annexin1 and rbohc loss of function mutants are impaired in [Ca2+]cyt-dependent transcriptional responses under NaCl stress (Chung et al., 2008; Laohavisit et al., 2013).
Although not demonstrated in roots, the Arabidopsis Actin-Related Protein2/3 (ARP2/3) acts to limit NaCl-induced [Ca2+]cyt increase, partly by limiting Ca2+ release from mitochondria. In the arp2/3 mutant, the [Ca2+]cyt increase is greater than wild type and so is the extent of mitochondrial-driven cell death (Zhao et al., 2013). Release of vacuolar Ca2+ to the cytosol in Arabidopsis roots may be by a Na+/Ca2+ exchanger encoded by AtNCL (Na+/Ca2+ Exchanger-Like; Wang et al., 2012; Li P.H. et al., 2016). This tonoplast protein is thought to sequester Na+ into the vacuole, coupled to the release of vacuolar Ca2+. The concomitant increase in [Ca2+]cyt could provide a negative feedback mechanism to limit further transport as Ca2+ binding to the exchanger’s EF hands has been shown to be inhibitory in vitro (Li P.H. et al., 2016). Pharmacological approaches have also implicated internal stores in the NaCl-induced [Ca2+]cyt increase in roots of both Arabidopsis and rice. Inhibitors of store release of Ca2+ by cADPR (cyclic ADP ribose) and inositol trisphosphate (InsP3) suggested involvement of the InsP3 pathway in Arabidopsis roots (Tracy et al., 2008). Moreover, salt and hyperosmotic stress in Arabidopsis roots caused an InsP3 accumulation that correlated well with [Ca2+]cyt increase (DeWald et al., 2001). The rice [Ca2+]cyt signal was also sensitive to disruption of putative InsP3-gated store release whilst impairment by thapsigargin implicated the ER as a participating store (Zhang Y. et al., 2015). However, using ER-targeted YC3.6, Bonza et al. (2013) detected an increase in Arabidopsis root ER [Ca2+] in response to salt stress. This followed the salt-induced [Ca2+]cyt increase and critically, a drop in ER [Ca2+] prior to the [Ca2+]cyt increase was never observed. Therefore, with the level of resolution available, it appears that in this system the ER does not contribute to the [Ca2+]cyt signal through store release but acts to return [Ca2+]cyt to resting levels. Studies on Populus euphratica cultured cells identified the vacuole as the site of InsP3 and cADPR action (Zhang X. et al., 2015). Certainly, Phospholipase C isoforms (as the source of InsP3) are firmly implicated in salt stress responses (reviewed by Ruelland et al., 2015).
The genetic identities of the endomembrane Ca2+-permeable channels implicated by pharmacological studies remain elusive. GLRs have recently been postulated to be involved in ER Ca2+ release (Weiland et al., 2016). The TPC1 vacuolar channel of Arabidopsis would be capable of releasing Ca2+ to the cytosol and recent analyses of its crystal structure has thrown greater light on its regulation by voltage and EF hands (Guo et al., 2016; Kintzer and Stroud, 2016). At current resolution afforded by YC3.6, its loss does not appear to have a significant impact on the magnitude of the Arabidopsis root [Ca2+]cyt increase to salt, rather it slightly delays the response (Choi et al., 2014). However, TPC1 has a significant part to play in the propagation of a [Ca2+]cyt wave that travels from the root apex through the cortical and endodermal tissue to signal the NaCl challenge to the shoots and elicit a transcriptional response. ROS also relay a salinity stress signal (reliant on RBOHD) in Arabidopsis leaves (Miller et al., 2009) but whether this occurs in roots and is involved in propagating the [Ca2+]cyt wave remains to be tested.
Salinity-induced [Ca2+]cyt elevation in roots drives a transcriptional response (Laohavisit et al., 2013; Zhang Y. et al., 2015) and post-translational modifications. The proteins sensing the NaCl-induced [Ca2+]cyt increase are now being elucidated. For example, the Arabidopsis vacuolar Two Pore K+ channel 1 (TPK1) would bind Ca2+, and open to release K+ to the cytosol to maintain a favorable Na+/K+ ratio (Latz et al., 2013). This could be further enhanced by phosphorylation by CPK3, which requires micromolar [Ca2+] for activity. CPK3 is present at both the PM and vacuole. It does not appear to contribute to a transcriptional response under salt stress but has a discrete set of protein targets to phosphorylate (Mehlmer et al., 2010). On prolonged salt stress, CPK29 expression is induced. This protein can phosphorylate TPK1 at sub-micromolar [Ca2+] and is envisaged to be part of longer-term K+ homeostasis in adapted roots (Latz et al., 2013). Also in Arabidopsis, CPK27 (present at the root PM) acts to promote Na+ efflux (Zhao et al., 2015). Intriguingly, CPK7 acts to limit water transport in Arabidopsis roots through lowering PIP1 aquaporin abundance (Li et al., 2015) but whether this is relevant to salinity or osmotic stress is not yet known. It can be readily envisaged that calmodulins will bind Ca2+ and as these are negative regulators of CNGC channels (Hua et al., 2003), would act to limit further Na+ or Ca2+ influx at the PM. Further, CaM activation of ACAs (Autoinhibited Ca2+ ATPases) would restore [Ca2+]cyt to pre-stimulus levels (Boursiac and Harper, 2007). Expression of ACAs varies with salt stress and, as shown by rice roots, can relate to salt tolerance (Yamada et al., 2014).
The Salt Overly Sensitive (SOS) pathway lies downstream of the root [Ca2+]cyt increase. Delineated in Arabidopsis and now acknowledged as operating in crops and trees (Thoday-Kennedy et al., 2015), the SOS pathway leads to Na+ efflux from the cytosol. Efflux across the PM is mediated by the SOS1 Na/H+ antiporter. Salt stress induction of SOS1 transcription lies downstream of Annexin1 in Arabidopsis roots and as SOS1 is required for adaptive adventitious root formation, the annexin1 loss of function mutant accordingly produces fewer of these than wild type (Laohavisit et al., 2013). Additionally, the stability of salt stress-induced SOS1 transcript requires RBOHC (Chung et al., 2008), further suggesting that this NADPH oxidase and Annexin1 may be in the same pathway. In Arabidopsis roots, increased [Ca2+]cyt is sensed at the PM by CBL4 (SOS3) which can then react with the serine/threonine protein kinase CIPK24 (SOS2) (Liu et al., 2000). The resultant CBL/CIPK complex phosphorylates the PM Na/H+ antiporter SOS1 at its auto-inhibitory C-terminus to achieve activation of Na+ efflux (Quintero et al., 2011). Activation of SOS1 can also be achieved in Arabidopsis by Mitogen Activated Protein Kinase6 (MPK6) and loss of MPK6 function impairs root growth under salt stress (Yu et al., 2010). MPK6 is activated by a phosphatidic acid produced under salt stress by Phospholipase Dα1 (PLDα1) (Yu et al., 2010). As this PLD isoform contains a Ca2+-binding C2 domain for its activation it is feasible that salt-induced [Ca2+]cyt increase could activate SOS1 through this lipid-mediated pathway via MPK6 (Yu et al., 2015). This may help explain the importance of activation of PLDs in salt-stressed crop roots such as barley (Meringer et al., 2016). SOS2 may also promote activity of the vacuolar V-type H+-ATPase to provide the driving force for Na+ sequestration (Batelli et al., 2007). The NHX1 Na+-H+ exchanger may also be regulated by SOS2 to aid vacuolar Na+ sequestration (Qiu et al., 2004) and SOS2 activation of the vacuolar CAX1 Ca2+/H+ antiporter would help terminate a [Ca2+]cyt signature through Ca2+ sequestration (Cheng et al., 2004). Other CAX are involved in root tolerance of salt stress and the pathways to their induction and regulation now need to be identified (Yamada et al., 2014).
Salt exposure puts the plant’s ER under stress, leading to an accumulation of unfolded or misfolded proteins that could lead to cell death (Liu et al., 2007, 2011). Such ER stress triggers upregulation of a suite of responses termed the “Unfolded Protein Response” (UPR), in which folding capacity is upregulated (including by Ca2+-regulated chaperones), translation is curtailed and the ER-associated degradation pathway acts to lower the aberrant protein load (Deng et al., 2013; Ruberti et al., 2015; Hossain et al., 2016; Wan and Jiang, 2016). Expression of the ER Ca2+-binding chaperones Calnexin and Calreticulin has been shown to upregulated in the Arabidopsis UPR (Christensen et al., 2008; Liu et al., 2011) and it would now be interesting to test whether these are involved in regulating levels of Ca2+ in the ER under stress. However, expression of rice’s only calnexin gene is decreased under salt stress (Sarwat and Naqvi, 2013). It is not yet clear whether the salt-induced [Ca2+]cyt signal in roots (or other parts of the plant) helps initiate or regulate the UPR. It is feasible that the salt-induced [Ca2+]cyt increase activates the root PM Phospholipase C2 (Hunt et al., 2004) because the Arabidopsis loss of function mutant is hypersensitive to tunicamycin, which can induce the UPR (Kanehara et al., 2015). With IP3 as the product of Phospholipase C activity, this would implicate IP3-mediated release of Ca2+ from stores. Indeed, for Arabidopsis seedlings the application of 2-aminoethoxydiphenyl borate as an inhibitor of IP3-mediated Ca2+ release prevented salt stress-induced transcription of BIP1/2 (encoding an ER chaperone) as a diagnostic of a UPR response (Liu et al., 2011). Intriguingly, application of La3+ as a blocker of PM Ca2+ influx prevented the upregulation of UPR gene expression which was produced by application of spermine to Arabidopsis seedlings (Sagor et al., 2015). Exogenous spermine (as with salt) can depolarise the root epidermal PM (Pottosin et al., 2014) and can also attenuate hydroxyl radical-induced cation fluxes at root epidermal PM (Zepeda-Jazo et al., 2011). Whether spermine and salt stress share a common pathway to the UPR response merits further investigation.
The hyperosmotic challenge in [Ca2+]cyt determinations is acute and does not mimic the chronic, progressive drought conditions that roots may face. Nevertheless, such studies have proved fruitful. As described above, the Arabidopsis OSCA1 PM Ca2+ influx channel drives the root’s initial hyperosmotic stress [Ca2+]cyt signal (Yuan et al., 2014). In an elegant study, heterologous expression of Arabidopsis genes in Chinese Hamster Ovary (CHO) cells containing the Fura-2 Ca2+-reporting dye lead to the identification of Calcium-permeable Stress-gated cation Channel1 (CSC1; Hou et al., 2014), a close relative of OSCA1. CSC1 has been characterized in CHO cells as a PM Ca2+-permeable channel that is activated by hyperosmotic stress. It is resident in plant PM (Hou et al., 2014) and is expressed in roots but to date an in planta role remains unreported. Targeting aequorin to the cytosolic face of the Arabidopsis vacuolar membrane has revealed the capacity of the vacuole to release Ca2+ in response to acute hyperosmotic stress, with pharmacological intervention suggesting an involvement of InsP3 (Knight et al., 1997). As with salt stress, hyperosmotic stress-induced [Ca2+]cyt increase could activate Phospholipases and initiate lipid signaling. Osmotic stress activates PLD in barley roots (Meringer et al., 2016). Although not tested directly, phosphatidic acid downstream of PLD could be involved in the activation of two sucrose non-fermenting-1 related protein kinase 2 proteins (SnRK2.4 and 2.10) in Arabidopsis roots under hyperosmotic stress. These SnRK2s are also activated under salt stress and relocate from the root epidermal cytosol to the PM; loss of function impairs root growth (McLoughlin et al., 2012). Work using Golgi-targeted aequorin in Arabidopsis seedlings has shown that an increase in Golgi [Ca2+] follows the [Ca2+]cyt increase induced by hyperosmotic stress, suggesting that this organelle helps terminate the [Ca2+]cyt signal (Ordenes et al., 2012).
The ABA produced under drought stress inhibits primary root growth and [Ca2+]cyt is likely to play a role in the signaling pathway as exogenous ABA elevates root [Ca2+]cyt, which in Arabidopsis roots is controlled by the PM Proline-rich Extensin-like Receptor Kinase4 (PERK4) (Bai et al., 2009). PERK4’s extracellular domain is wall associated and its intracellular domain has kinase activity. PM HACCs lie downstream of ABA and PERK4. The perk4 mutant is not only impaired in ABA-induced HACC activity and [Ca2+]cyt activation but also in ABA inhibition of primary root elongation (Bai et al., 2009). This implies that [Ca2+]cyt elevation acts to arrest growth. RBOHD/F may lie downstream of PERK4 and upstream of HACCs in the root ABA pathway if the HACCs were ROS-activated. The rbohd/f mutant is impaired in both ABA-induced HACC activation and [Ca2+]cyt elevation (Jiao et al., 2013). Another possibility is that CNGCs are involved as ABA can increase cGMP levels (Isner et al., 2012.). GLRs are firmly implicated in the drought response; overexpression of rice GLR1 and GLR2 enhances drought tolerance of both rice and Arabidopsis (Lu et al., 2014). The [Ca2+]cyt increase is likely to lead to a transcriptional response as Ca2+-sensing proteins are activated. ABA increases abundance and activity of Arabidopsis CPK4 and CPK11 leading to phosphorylation of the ABA-responsive transcription factors ABF1 and ABF4 and induction of drought stress genes (Zhu et al., 2007). Drought leads to CAMTA1 activity and the regulation of discrete gene sets including those for drought recovery (Pandey et al., 2013). In maize roots, drought stress triggers activity of CIPK8 (which interacts with CBL1, 4, and 9) and likely leads to adaptive transcription (Tai et al., 2016).
Hypoosmotic stress also elevates [Ca2+]cyt and is relevant to waterlogged soils. In Arabidopsis, root PM harbors two mechanosensitive Ca2+-permeable channels, MCA1 and MCA2 (Mid1-Complementing Activity; Kamano et al., 2015). MCA1 responds to hypoosmotic stress to elevate [Ca2+]cyt (Nakagawa et al., 2007). Rice only harbors an MCA1 in the PM. It is present in the root and mediates hypoosmotic shock-induced [Ca2+]cyt expression in cultured cells, probably lying upstream of NADPH oxidase activity (Kurusu et al., 2012). Abundant water not only exposes roots to potential hypoosmotic stress but also risks limiting their oxygen supply, the consequences of which are reviewed in the following section.
Oxygen deficiency (hypoxia) or absence (anoxia) causes transient increases in root [Ca2+]cyt but the signature is organ- and species-dependent (reviewed by Shabala et al., 2014). For example, when challenged by anoxia, root protoplasts from hypoxia-tolerant rice display a greater [Ca2+]cyt signature than hypoxia-intolerant wheat root protoplasts (Yemelyanov et al., 2011). The location of the Ca2+ influx also varies; use of pharmacological blockers showed that the rice signature was generated by both PM influx and store release whilst wheat appeared to rely solely on stores (Yemelyanov et al., 2011). The types of Ca2+ channels mediating the [Ca2+]cyt increase have yet to be identified in any species. However, those activated by PM voltage depolarization are implicated. As O2 deficiency lessens ATP production, activity of the PM H+-ATPase can be compromised thus resulting in a less negative (depolarized) PM voltage as H+ efflux is curtailed. The extent and duration of membrane depolarization varies with sensitivity to O2 deprivation and cell type. Values of -70 to -80 mV have been reported for O2-deprived barley root cells (Zeng et al., 2014) and theoretically these would be sufficient to activate PM depolarization-activated Ca2+ influx channels (Miedema et al., 2008) to contribute to an hypoxia/anoxia [Ca2+]cyt signature. Downstream of the [Ca2+]cyt signature, it is likely that CaM and ROPs (Rho GTPase) are activated (reviewed by Shabala et al., 2014). In Arabidopsis roots, hypoxia causes rapid upregulation of CML38 expression and this protein appears to require Ca2+ to associate with the cytosolic stress granules that form and store messenger RNA ribonucleoproteins (Lokdarshi et al., 2016).
RBOH activity is firmly implicated in the response to low O2. In Arabidopsis, RBOHD expression is induced by hypoxia and is required for transcription of hypoxia-induced genes (Yang and Hong, 2015). Hypoxia also induces ethylene production and in wheat roots this causes RBOH induction (Yamauchi et al., 2014). A further level of regulation has been found in Arabidopsis; the Hypoxia Responsive Universal Stress Protein 1 (HRU1) interacts with GTP-bound ROP2 and RBOHD (Gonzali et al., 2015). HRU1 is one of 44 putative universal stress proteins in Arabidopsis. It exists as a cytosolic dimer but anoxia promotes monomer formation and increased association with the PM. There it is thought to be part of a mobile complex with ROP2 and AtRBOHD leading to activation of that NADPH oxidase (Gonzali et al., 2015). Although RBOH activity has been linked to PM Ca2+ channel activation in several abiotic stress scenarios, hypoxia and anoxia have yet to be tested.
Ethylene has been shown to activate PM Ca2+-permeable channels (with a weak voltage dependence) in tobacco suspension cells (Zhao et al., 2007) and it remains a possibility that these may play a part in the root hypoxia [Ca2+]cyt signal with RBOH as an intermediary. Oxygen deprivation (and also sulfur and phosphate, Pi, deprivation) triggers programmed cell death (PCD) in mid-cortical cells for aerenchyma formation (Fagerstedt, 2010). This PCD is stimulated by ethylene and ROS and involves Ca2+ (Xu et al., 2013; Petrov et al., 2015). In wheat roots, anoxia causes mitochondria to release their Ca2+ and high [Ca2+]cyt causes cytochrome c release (Virolainen et al., 2002). The abnormal mitochondrial ultrastructure in Arabidopsis caused by hypoxia is partially phenocopied by loss of GLR3.5 from the inner mitochondrial membrane, suggesting that this channel must be deactivated during PCD (Teardo et al., 2015). Further channels must now be identified to understand the hypoxic/anoxic response. Recent work on Arabidopsis roots has shown distinct, cell-specific levels of [Ca2+]cyt after 24 h of hypoxia and highlighted the importance of CAX11 in controlling meristem [Ca2+]cyt (Wang et al., 2016). Loss of CAX4 function resulted in lower tolerance of hypoxia thus further demonstrating the importance of Ca2+ sequestration in this stress response (Wang et al., 2016).
Investigating [Ca2+]cyt elevation in response to nutrient deprivation or resupply is technically challenging, particularly if using aequorin. Nevertheless it is now clear that nutrient levels can induce [Ca2+]cyt changes and that downstream Ca2+ sensors regulate appropriate responses. The nutritional status of the root will have a part to play in determining the [Ca2+]cyt signatures, particularly of the endodermis as the extent of suberization is set by nutrition (Barberon et al., 2016) and suberin lamellae determine endodermal [Ca2+]cyt responses (Moore et al., 2002). To date, potassium, nitrate and boron have been studied.
Plants must maintain cytosolic K+ at around 80 mM for optimal growth even though soil concentration may be sub-millimolar and they deploy a multigene family of K+ transporters in homeostatic control (Shabala and Pottosin, 2014). As extracellular K+ decreases, the root epidermal PM hyperpolarizes and [Ca2+]cyt increases (Demidchik et al., 2002). This increase can be abolished by gadolinium, implicating PM Ca2+ influx. The genetic identities of the PM Ca2+-permeable channels that would effect the [Ca2+]cyt increase remain unknown. The hyperpolarised PM correlates with induction of HAK5 expression in tomato root (Nieves-Cordones et al., 2008). HAK5 encodes the PM High Affinity K+ transporter that facilitates K+ uptake from low external concentrations that are thermodynamically unfavorable for channel-mediated influx. In Arabidopsis roots, low K+-induction of HAK5 expression (and other K+-deprivation genes) has been shown to depend on RBOHC activity and ROS (Shin and Schachtman, 2004). At present it is unclear whether the hyperpolarization of the PM directly activates RBOHC (which as an electron exporter may be voltage-dependent) or whether ROS-activated PM Ca2+ channels are involved in the K+-deprivation [Ca2+]cyt signal. HAK5 activity in Arabidopsis roots is regulated by CIPK23-mediated phosphorylation, downstream of CBL1, CBL8, CBL9, and CBL10 (Ragel et al., 2015). The extent to which the transcriptional response to low K+ availability is governed by [Ca2+]cyt is unclear but deficiency does result in upregulation of transcripts of Ca2+ signaling proteins (CaM, CBL, CIPK) in Arabidopsis seedlings (Armengaud et al., 2004) and sugarcane roots (Zeng et al., 2015). At higher external [K+], the AKT1 channel (Arabidopsis K+ Transporter1) facilitates uptake and its activity is promoted by CIPK23-mediated phosphorylation, downstream of CBL1 and CBL9 (Li et al., 2006; Cheong et al., 2007). This regulation is recapitulated in rice roots where CIPK23/CBL1 activate AKT1 (Li et al., 2014).
Nitrate is the most important form of nitrogen for agriculture and deprivation triggers significant transcriptional and developmental responses. The effect of nitrate withdrawal on [Ca2+]cyt has yet to be reported but recently it was shown that nitrate-starved Arabidopsis roots responded to nitrate resupply with a rapid, monophasic transient increase in [Ca2+]cyt that was sensitive to lanthanides and phospholipase C (PLC) inhibition (Riveras et al., 2015). Lanthanum also blocked nitrate-induced InsP3 production, suggesting that Ca2+ influx across the PM activated a PLC. The [Ca2+]cyt and InsP3 increases were entirely dependent on the PM nitrate influx transporter NRT1.1 (Nitrate Transporter1.1; Riveras et al., 2015). By using the nrt1.1 mutant and pharmacological blockers, nitrate-induced gene transcription was also found to lie downstream of NRT1.1, and [Ca2+]cyt elevation from PM influx and InsP3-gated store release. Calcium is also key to the regulation of nitrate uptake capacity as CIPK23, which is activated by CBL9 and CBL1, and dephosphorylated by ABI2 (a member of the PP2C protein phosphatase family; Léran et al., 2015,), phosphorylates NRT1.1 under low nitrate condition, thus converting it from a low to high affinity transporter (Ho et al., 2009). By contrast CIPK8 positively regulates the low-affinity phase of the nitrate primary response which includes transcriptional regulation, but its regulation of primary root elongation is concentration independent in Arabidopsis (Hu et al., 2009). CBL7, which is upregulated under nitrate deprivation, positively regulates the nitrate-dependent induction of NTR2.4 and NTR2.5 gene expression (Ma Q. et al., 2015). Given the lack of a [Ca2+]cyt reporter line available in crops up until recently for rice, little is known about nitrate deficiency-induced [Ca2+]cyt signaling but CaM protein abundance of wheat roots declines under nitrate deficiency, suggesting a remodeling of signaling systems (Jiang et al., 2015).
Boron deficiency is widespread worldwide and particularly prevalent in China (Shorrocks, 1997). As B plays a dominant role in co-ordinating cell wall structure (Kobayashi et al., 1996), changes in cell wall stability are likely to influence the signal relayed into the cell upon B deprivation and indeed a rapid change in cell wall modulus has been observed (Goldbach et al., 2001). Early work in Vicia faba showed a release of membrane-bound Ca2+ into the apoplast (Mühling et al., 1998), raising the possibility of Ca2+ signaling during the early stages of B deficiency. Increased levels of both [Ca2+]cyt and ROS have been suggested to lead to the increased root hair growth known to occur under B deprivation (González-Fontes et al., 2016). In cultured tobacco cells, transcriptional changes in response to short-term B deprivation (1 h) were abolished when withdrawing Ca2+ from the growth medium or upon treatment with the Ca2+ channel blocker lanthanum, thus implicating PM Ca2+ influx channels in generating a [Ca2+]cyt signal (Koshiba et al., 2010). However, a transient [Ca2+]cyt signal in direct response to B withdrawal has yet to be reported. Rather, work has focused on the possible remodeling of Ca2+ transport and signaling as a consequence of B deprivation.
Challenging cultured tobacco cells with Ca2+ resulted in a higher amplitude of [Ca2+]cyt transient in B-deprived cells (1 h deprivation) than those grown under replete conditions (Koshiba et al., 2010). This suggests that B deprivation rapidly “resets” the PM’s Ca2+ transport systems to generate altered [Ca2+]cyt responses. The [Ca2+]cyt response of B-deprived cells was sensitive to lanthanum and diphenyleneiodonium, pointing to the involvement of PM Ca2+ channels and NADPH oxidases respectively (Koshiba et al., 2010). Arabidopsis roots expressing the YC3.6 [Ca2+]cyt reporter exhibited higher levels of [Ca2+]cyt at the apex than controls after 6 and 24 h of B deprivation (Quiles-Pando et al., 2013). This time course of B deprivation also resulted in significant upregulation of CNGC19 (encoding a vacuolar channel), four genes of the ACA family of PIIB-type Ca2+-ATPases (ACA1,10,12,13) and CAX3 encoding a vacuolar cation-H+ antiporter (Quiles-Pando et al., 2013). This suite of transporters could effect Ca2+ efflux from the vacuole (CNGC19) to increase [Ca2+]cyt with clearance to the apoplast by ACA10-13 and sequestration to the vacuole by CAX3. How they are regulated remains to be determined, as does the involvement of the structurally compromised wall and the consequence of this higher level of apical [Ca2+]cyt. The area of higher [Ca2+]cyt reported appears to correspond with the zone of inhibition of primary root elongation and the induction of cell death (Oiwa et al., 2013; Camacho-Cristobal et al., 2015). Once again, ethylene production appears to be upstream of NADPH oxidase-driven ROS production in growth arrest and death (Oiwa et al., 2013; Camacho-Cristobal et al., 2015).
Eight CML genes were also significantly upregulated after a day’s B deprivation of Arabidopsis roots (CML11,12,23,24, 30.37,45.47), as were three CPK genes (CPK1,28,29) all suggesting a distinct change in intracellular Ca2+ signaling (Quiles-Pando et al., 2013). This B deprivation also caused upregulation of WRKY transcription factors (TF) (WRKY38,40,46), two three MYB family TF (MYB14,15,78) and downregulation of two BZIP family TFs (bZIP34,61) (González-Fontes et al., 2016). B deprivation has also been found to promote the senescence-associated WRKY6 TF in the root tip (Kasajima et al., 2010). It has been suggested that the CMLs and CPKs upregulated by B deprivation are part of the chain of events leading to TF activation in the nucleus (González-Fontes et al., 2016) and this now requires direct testing. CIPK8 also positively regulates nitrate induced up-regulation of BOR1, a gene encoding a boron transporter responsible for xylem loading (Hu et al., 2009), suggesting that root signaling results in preservation of shoot B supply.
At the opposite end of nutritional deprivation is heavy metal stress. Industrial activity, mining and modern agricultural practices can lead to soil contamination by heavy metals (defined here as 7 g/cm3 and above). Although some of these metals (such as Zn, Cu) are required as micronutrients they can be damaging in excess whilst others (such as Cd) have no physiological role and can be deleterious even at low concentrations, often impairing mineral nutrition. The consequences of heavy metal exposure have been reviewed recently by Singh et al. (2016) and these authors explore signaling pathways (although not explicitly addressing Ca2+), intersects with hormonal responses and detoxification.
Cadmium is a particular threat to Ca2+-based processes because of its similar size. A recent review by Chmielowska-Bak et al. (2014) summarized the effects of Cd on ROS accumulation, NO accumulation, MAP kinase activation and downstream responses in a wide range of plant systems and, importantly, did this as a function of Cd exposure. With regards to Ca2+ a key question is whether Cd (as a Ca2+ “substitute”) generates a signal in its own right or whether its impairment of Ca2+ homeostasis will be, in effect, the signal. Certainly Cd depolarises the rice root epidermal PM, which would impair Ca2+ influx and results in inhibition of root elongation (Li et al., 2012). Cd can permeate guard cell PM Ca2+ channels (Perfus-Barbeoch et al., 2002) and may compete with Ca2+ for entry into rice root hairs through PM HACC, thus limiting Ca2+ influx (Li et al., 2012). Competitive effects appear likely given the ability of exogenous Ca2+ addition to alleviate Cd inhibition of root growth of both terrestrial and aquatic plants, implying competition for uptake (Zhang et al., 2012; Rodriguez-Hernandez et al., 2015). Additionally, competitive inhibition by Cd of Ca2+ uptake through the wheat LCT1 transporter expressed in yeast has been observed (Clemens et al., 1998). Root hair growth in Arabidopsis is inhibited by Cd; it inhibits Ca2+ influx and so dissipates the apical [Ca2+]cyt gradient needed for growth (Fan et al., 2011). This could reflect block of root hair Ca2+ influx channels or Cd outcompeting Ca2+ for that influx pathway. However, Yeh et al. (2007) observed an increase in rice root [Ca2+]cyt following 15 min of Cd exposure, with a more tolerant variety sustaining a greater increase than a sensitive variety. In roots of the aquatic plant Typha latifolia, Cd exposure increases transcription of TPC1 which suggests that vacuoles might release Ca2+ to the cytosol (Rodriguez-Hernandez et al., 2015). Inhibitor treatments indicated involvement of NADPH oxidases, hydroxyl radicals and CIPK upstream of a MAP kinase induction that linked to tolerance (Yeh et al., 2007). Cd stimulates expression and activity of NADPH oxidases in cucumber roots but it is not known if these responses are Ca2+-dependent (Jakubowska et al., 2015). It is worth noting that it is not only an increase in [Ca2+]cyt that could stimulate NADPH oxidase activity but that also the restriction of Ca2+ entry to the cytosol could cause stimulation (Mortimer et al., 2008). It seems likely that in Arabidopsis roots it would be CAXs predominating in terminating a Cd-induced increase in [Ca2+]cyt. CAX4 mediates Cd and Ca2+ transport into Arabidopsis thaliana vacuoles and is needed for correct growth under Cd stress (Mei et al., 2009). CAX1 expression is higher in roots of the Cd-tolerant A. halleri compared to the sensitive A. thaliana (Baliardini et al., 2015).
A key point for future studies is the intersect between Cd and hormones in relation to [Ca2+]cyt. Exogenous Ca2+ can ameliorate Cd’s inhibition of Arabidopsis root growth by counteracting effects of NO on auxin homeostasis (Hu et al., 2013; Li P. et al., 2016; Yuan and Huang, 2016). Cd also interferes with auxin homeostasis in barley roots (Zelinova et al., 2015). Auxin itself can increase Arabidopsis root [Ca2+]cyt and this increase is mediated by CNGC14 at the PM, downstream of an unidentified auxin receptor (Shih et al., 2015). This begs the question of whether CNGC14 is an entry route for Cd and the pathway to disrupted auxin homeostasis. Additionally, Cd has been described as a “metallohormone” in that it triggers expression of brassinosteroid-regulated genes in Arabidopsis roots (Villiers et al., 2012). Brassinosteroids are themselves capable of transiently elevating [Ca2+]cyt in Arabidopsis roots (through PM Ca2+ influx) and activate a possible DACC in wheat root PM (Straltsova et al., 2015). Again this raises the question of channel identity to help elucidate the relationship between Cd and brassinosteroid signaling and combat the effects of this potent soil contaminant.
In contrast to Cd, transition heavy metals could be capable of generating ROS directly and so perturb [Ca2+]cyt signaling. Transition metals can catalyze production of hydroxyl radicals from superoxide anion and hydrogen peroxide through the Haber-Weiss reaction, with Cu+ and Fe2+ catalyzing hydroxyl radical production from hydrogen peroxide through the Fenton reaction (Richards et al., 2015). Unless levels of these catalytic metals are tightly controlled, production of hydroxyl radicals (the most potent of the ROS) could inflict significant oxidative damage. Taking Cu as the exemplar, it plays a positive role in hydroxyl radical-activated cell wall loosening for root elongation (Fry et al., 2002) and this could be coupled in Arabidopsis roots to hydroxyl radical-activation of PM Annexin1-mediated Ca2+ influx to stimulate exocytosis and growth (Foreman et al., 2003; Laohavisit et al., 2012). This model proposes regulation by extracellular hydroxyl radicals while Rodrigo-Moreno et al. (2013) have proposed that Cu+ binding at the intracellular face of the PM in Arabidopsis root tips catalyzes hydroxyl radical production to regulate ion flux. This would allow coupling of Cu transport into the root to regulation of PM Ca2+ influx channels. Certainly, low levels of Cu can promote elongation of Arabidopsis primary root and this effect is diminished by blocking PM Ca2+ influx channels (Demidchik et al., 2003). The effects of extracellular Cu on PM Ca2+ channels and therefore on [Ca2+]cyt signaling are likely to be complex. Electrophysiological analysis of Arabidopsis root epidermal PM has shown that Cu not only activates Ca2+ channels through ROS production (Annexin 1; Laohavisit et al., 2012) but also blocks channels, the molecular identity of which remains unknown (Demidchik et al., 2003).
Catalytic production of ROS by Cu is not the only route to modulating [Ca2+]cyt. Longer-term exposure to Cu can stimulate exocytosis-mediated ROS production. Lin et al. (2013) found that inhibiting vesicle traffic with brefeldin also inhibited Cu-stimulated ROS production in rice roots. Whether this involved NADPH oxidase or other ROS generators remains to be determined. Rice root [Ca2+]cyt increases in response to Cu addition and could link to NADPH oxidases and CIPK activity leading to MAP kinase activation (Yeh et al., 2007). It triggers oxidative stress in Populus roots that leads to regulation of CaM genes, implicating perturbation of [Ca2+]cyt (Guerra et al., 2009).
In common with Cd, excess Cu also alters auxin homeostasis in roots and interferes with NO signaling (Lequeux et al., 2010; Kolbert et al., 2012). In excess, Cu stunts Arabidopsis root and root hair elongation and can inhibit lateral root outgrowth (Kolbert et al., 2012). Accumulation of lignin has been observed in both Arabidopsis and rice roots (Lequeux et al., 2010; Liu et al., 2015) and in the former accumulation is associated with the endodermis. It would now be interesting to ascertain whether such wall modification changes [Ca2+]cyt signals in central cells or affects [Ca2+]cyt propagative signaling to the shoot. Root tip cell death has also been observed (Huang et al., 2007; Lequeux et al., 2010; Rodrigo-Moreno et al., 2013). Cu-induced cell death in rice roots was attenuated by chelating extracellular Ca2+ thus implicating Ca2+ influx across the PM (Huang et al., 2007) while in Arabidopsis roots cell death was attenuated by addition of Gd3+ or verapamil as PM Ca2+ channel blockers (Rodrigo-Moreno et al., 2013).
Gd3+ is routinely used as a PM Ca2+ channel blocker, for example with proven efficacy against Arabidopsis root epidermal and root hair HACC, DACC and VICC (Véry and Davies, 2000; Demidchik et al., 2002; Miedema et al., 2008). It is also effective against the root PM hydroxyl radical-activated and H2O2-activated Ca2+ influx channels (Demidchik et al., 2003, 2007) and the Annexin1-mediated pathway (Laohavisit et al., 2012). As a consequence Gd3+ lowers Ca2+ influx (measured with 45Ca; Demidchik et al., 2002). From this it can be anticipated that Gd3+ would have the capacity to dampen stress-induced [Ca2+]cyt signaling in roots, including that mediated by oxidative stress (Demidchik et al., 2003, 2007) and elicited by NaCl (Laohavisit et al., 2013). Pb is less well studied in terms of [Ca2+]cyt. Exogenous Ca2+ can protect against root Pb accumulation, suggesting competitive uptake (Rodriguez-Hernandez et al., 2015). However, Pb was found to evoke Ca2+ accumulation and diphenyleneiodinium-sensitive ROS increase in rice roots, the latter leading to MAPK activation (Huang and Huang, 2008). Entry of Pb into Arabidopsis roots involves CNGC1 as its deletion confers greater tolerance but whether Pb permeates this channel is unknown (Sunkar et al., 2000). Pb has also been shown to increase expression of TPC1 in Typha roots (Rodriguez-Hernandez et al., 2015) but whether this results in increased [Ca2+]cyt in this or other roots is not known yet.
Roots experience a range of mechanical stimuli, induced as they encounter soil particles or neighboring roots. An increase in [Ca2+]cyt together with an apoplastic alkanisation are early effects induced by mechanical stimuli. One of the known downstream events from the increase in [Ca2+]cyt is the upregulation of touch-sensitive genes such as CML12 and CML24 (Braam, 1992). Mechanically triggered [Ca2+]cyt changes are dependent on the type of stimulus and the responding tissue (Legue et al., 1997; Monshausen et al., 2009). For example, manually bending an Arabidopsis root induces a rapid, biphasic increase in [Ca2+]cyt on the convex side of the roots where cells are stretched (Monshausen et al., 2009) while previously compressed cells on the concave side of the roots show a monophasic and less intense increase in [Ca2+]cyt upon return to their resting position. The source of Ca2+ is likely to be extracellular (Monshausen et al., 2009; Richter et al., 2009; Kurusu et al., 2012); with internal stores being mobilized to amplify the response (Chehab et al., 2009; Toyota and Gilroy, 2013).
Over-expressing MCA1 in Arabidopsis lead to an enhanced [Ca2+]cyt transient post mechanical stimulation by the addition of a membrane crenator, however, the mca1 mutant showed no difference from the wild type (Nakagawa et al., 2007). Accordingly, Shih et al. (2014) showed no change in mca1 apoplastic alkalinisation following root bending, which is closely related to the [Ca2+]cyt signature. Thus some levels of compensation occur in mutants deficient in mechanosensitive Ca2+ channels. In contrast to the MCAs, MSLs (MscS-Like) were identified in Arabidopsis due to their sequence similarity to the bacterial Mechanosensitive channels of small conductance (MscS) (Haswell et al., 2008). These are encoded by a multiple gene family of 10 members, with MSL9 and MSL10 found in the PM of root cells and required for a mechanosensitive channel activity. They are predominantly anion channels and their relationship to mechano-stimulated [Ca2+]cyt increase has yet to be shown but may be through PM voltage regulation. At this point, it is unclear whether these channels represent mechano-sensors or act downstream of yet unknown mechano-sensors.
Recently, the PM receptor-like kinase FERONIA has been implicated in regulating the Arabidopsis mechano-stimulated [Ca2+]cyt increase and downstream transcriptional regulation (Shih et al., 2014). feronia mutants lack the second peak of the biphasic increase in [Ca2+]cyt elicited in stretched cells by manual bending. However, the mechanism by which FERONIA can regulate [Ca2+]cyt remains unclear as its kinase activity is not essential and targets are unknown. As far as we know, this study was the first in which a mutant with an aberrant mechano-stimulated [Ca2+]cyt increase also showed a root skewing phenotype. Root skewing (deviation from the vertical when grown on vertical or inclined agar) may be influenced by mechano-sensing. At this point, it is unclear whether FERONIA also plays a role in the mechanical induction of lateral root formation (Richter et al., 2009).
Cold plays a key role in the regulation of physiology and development; the signaling processes relaying non-stressful temperatures (12°C and above) have been reviewed by Wigge (2013). The signaling cascades activated by cold stress (typically 4°C experimentally) and their relations with hormonal signaling have been reviewed by Knight and Knight (2012), Jeon and Kim (2013), Shi et al. (2015) and Eremina et al. (2016). Most studies on the effect of cold stress on [Ca2+]cyt report on seedlings or leaves. From these it is well established that [Ca2+]cyt elevation involves both Ca2+ influx across the PM and release from predominantly vacuolar stores (e.g., Knight et al., 1996; Mazars et al., 1997; Knight and Knight, 2000: Zhu et al., 2013). By using an extracellular Ca2+-reporting microelectrode, Sulaiman et al. (2012) confirmed that Ca2+ influx from the extracellular space is a component of the Arabidopsis root response to cold stress. For Arabidopsis roots, the [Ca2+]cyt response to cold stress measured using aequorin is lower in amplitude and of much shorter duration than leaves (Zhu et al., 2013). Using aequorin Plieth et al. (1999) determined that the faster the rate of cooling Arabidopsis roots, the greater the [Ca2+]cyt response. Sensitivity and magnitude of the [Ca2+]cyt cooling response were enhanced by low temperature but repeated exposure to cold lead to desensitizing of the response. These results imply the existence of Ca2+ transport systems that could be regulated at the post-translational and possibly transcriptional levels. In Arabidopsis, cold-induced [Ca2+]cyt elevation would activate a root PM calcium/calmodulin-regulated receptor-like kinase (CRLK1; Yang et al., 2010). This would then activate a specific mitogen-activated protein kinase kinase kinase (MEKK1) that then targets protein kinase kinase2 (MKK2; Furuya et al., 2013). This pathway leads to gene activation and freezing tolerance (Furuya et al., 2014). [Ca2+]cyt elevation would also activate CIPK3, that acts an intersect with ABA signaling (Kim et al., 2003). Part of the transcriptional response to cold in Arabidopsis could be activated by increased [Ca2+]cyt through CAMTA3 regulation of the transcriptional regulator CBF2 (C-Repeat/Dehydration Responsive Element Binding Factor2) (Doherty et al., 2009).
Cold stress has been found to depolarise the PM of root cells from cucumber and Triana bogotensis (Lyalin and Ktitorova, 1969; Minorsky and Spanswick, 1989), consistent with the effect of Ca2+ influx across the PM. A modeling exercise revealed the possible importance of a PM DACC in cold stress-induced [Ca2+]cyt elevation of roots (White, 2009). Cold has been shown to activate a PM DACC in leaf protoplasts (Carpaneto et al., 2007) but this has not yet been shown for roots. As microtubule destabilization activates the Arabidopsis root PM DACC (Thion et al., 1998), this could link to the increased cold-induced [Ca2+]cyt signal observed when microtubules are disrupted in Nicotiana plumbaginifolia leaf protoplasts (Mazars et al., 1997). Actin depolymerization is implicated in cold-induced Ca2+ influx across the PM into Medicago sativa suspension cells (Orvar et al., 2000). This in turn could relate to the cold-induced activation of pear pollen PM HACC that involves actin depolymerization (Wu et al., 2012). It would be timely therefore to investigate root PM for cold effects on HACC and DACC, exploring also the involvement of the cytoskeleton. In common with mechanical stress, cold stress could perturb the PM bilayer sufficiently to change Ca2+ channel activity. Work on M. sativa suspension cells showed that increasing membrane fluidity at 4°C prevented the Ca2+ influx across the PM that is necessary to trigger gene expression for freezing tolerance (Orvar et al., 2000). Conversely, imposing membrane rigidity at normal temperature triggers activation of the Ca2+-dependent MEKK1-MKK2-MPK4 pathway in Arabidopsis seedlings (Furuya et al., 2014). The possibility that mechanosensitive channels are involved in cold-induced [Ca2+]cyt signaling merits investigation. However, recent work on rice roots indicates another route to Ca2+ influx. COLD1 has been identified as a PM activator of the heterotrimeric G protein subunit α (Ma Y. et al., 2015). It is required for cold-activated Ca2+ influx to roots (measured using extracellular Ca2+-reporting microelectrode) and elevation of root [Ca2+]cyt (aequorin and cameleon determinations). This indicates that heterotrimeric G proteins lie upstream of PM Ca2+ channels in the cold response. In common with other stress responses, NADPH oxidase activity also appears in cold stress and needs to be placed in relation to Ca2+ channels. Arabidopsis roots exposed to cold stress use AtSRC2 (Soybean gene Regulated by Cold2) to enhance Ca2+ activation of the AtRBOHF NADPH Oxidase (Kawarazaki et al., 2013). This suggests that NADPH oxidase activity can lie downstream of Ca2+ channel activity. Curtailing cold-induced [Ca2+]cyt elevation could involve vacuolar CAX1 activity in Arabidopsis (Catala et al., 2003).
A repeated message from this review is how incomplete our knowledge is of the channels mediating stress-induced [Ca2+]cyt increases, and by extension those of organelles. Many members of channel gene families still await characterization. The identification of new families of channels is challenging and will require different approaches linking forward and reverse genetics to electrophysiology. Targets of Ca2+-binding and interacting proteins also require further study. Components common to different abiotic stresses are emerging such as Arabidopsis CIPK23 in K+ and nitrate deprivation. These common regulatory components are likely to represent critical steps where complex stress signals encountered in the soil are integrated in unified responses. Receptor like kinases such as FERONIA or PERK4 have emerged as new components in [Ca2+]cyt signaling and perhaps other related proteins will be found to have a role in abiotic stress signaling. Remodeling of calcium signaling machinery after stress is also apparent with the possibility of components common to different stresses. For example, Arabidopsis CNGC19 is upregulated under B limitation and salinity stress (Kugler et al., 2009). Finally, [Ca2+]cyt-dependent transcriptional responses can be delineated and future work could include the impact of stress-induced calcium signaling on epigenetic inheritance (Sani et al., 2013; Probst and Scheid, 2015).
All authors listed, have made substantial, direct and intellectual contribution to the work, and approved it for publication. KW, EM, SS, and JD wrote the review. KW produced the figures.
Funding for this work was from the BBSRC (BB/K009869/1 and Doctoral Training Programme) and the University of Cambridge Broodbank Trust.
The authors declare that the research was conducted in the absence of any commercial or financial relationships that could be construed as a potential conflict of interest.
Armengaud, P., Beitling, R., and Amtmann, A. (2004). The potassium-dependent transcriptome of Arabidopsis reveals a prominent role of jasmonic acid in nutrient signaling. Plant Physiol. 136, 2556–2576. doi: 10.1104/pp.104.046482
Bai, L., Zhang, G., Zhou, Y., Zhang, Z., Wang, W., Du, Y., et al. (2009). Plasma membrane-associated proline-rich extension-like receptor kinase 4, a novel regulator of Ca2+ signaling, is required for abscisic acid responses in Arabidopsis thaliana. Plant J. 60, 314–327. doi: 10.1111/j.1365-313X.2009.03956.x
Baliardini, C., Meyer, C.-L., Salis, P., Saumitou-Laprade, P., and Verbruggen, N. (2015). CATION EXCHANGER1 cosegregates with cadmium tolerance in the metal hyperaccumulator Arabidopsis halleri and plays a role in limiting oxidative stress in Arabidopsis Spp. Plant Physiol. 169, 549–559. doi: 10.1104/pp.15.01037
Barberon, M., Vermeer, J. E. M., De Bellis, D., Wang, P., Naseer, S., Anderson, T. G., et al. (2016). Adaptation of root function by nutrient-induced plasticity of endodermal differentiation. Cell 164, 447–459. doi: 10.1016/j.cell.2015.12.021
Batelli, G., Verslues, P. R., Agius, F., Qiu, Q., Fujii, H., Pan, S., et al. (2007). SOS2 promotes salt tolerance in part by interacting with the vacuolar H+-ATPase and upregulating its transport activity. Mol. Cell Biol. 27, 7781–7790. doi: 10.1128/MCB.00430-07
Behera, S., Wang, N., Zhang, C., Schmitz-Thom, I., Strohkamp, S., Schueltke, S., et al. (2015). Analyses of Ca2+ dynamics using a ubiquitin-10 promoter-driven Yellow Cameleon 3.6 indicator reveal reliable transgene expression and differences in cytoplasmic Ca2+ responses in Arabidopsis and rice (Oryza sativa) roots. New Phytol. 206, 751–760. doi: 10.1111/nph.13250
Bonza, M. C., Loro, G., Behera, S., Wong, A., Kudla, J., and Costa, A. (2013). Analyses of Ca2+ accumulation and dynamics in the endoplasmic reticulum of Arabidopsis root cells using a genetically encoded cameleon sensor. Plant Physiol. 163, 1230–1241. doi: 10.1104/pp.113.226050
Bonza, M. C., Luoni, L., Olivari, C., and DeMichelis, M. I. (2016). Plant type 2B Ca2+-ATPases: the diversity of isoforms of the model plant Arabidopsis thaliana. Adv. Biochem. Health Dis. 14, 227–241.
Boursiac, Y., and Harper, J. F. (2007). The origin and function of calmodulin regulated Ca2+ pumps in plants. J. Bioen. Biomem. 39, 409–414. doi: 10.1007/s10863-007-9104-z
Braam, J. (1992). Regulated expression of the calmodulin-related TCH genes in cultured Arabidopsis cells – induction by calcium and heat shock. Proc. Natl. Acad. Sci. U.S.A. 89, 3213–3216. doi: 10.1073/pnas.89.8.3213
Camacho-Cristobal, J. J., Martin-Rejano, E. M., Begona Herrera-Rodriguez, M., Teresa Navarro-Gochicoa, M., Rexach, J., and Gonzalez-Fontes, A. (2015). Boron deficiency inhibits root cell elongation via an ethylene/auxin/ROS-dependent pathway in Arabidopsis seedlings. J. Expt. Bot. 66, 3831–3840. doi: 10.1093/jxb/erv186
Carpaneto, A., Ivashikina, N., Levchenko, V., Krol, E., Jeworutzki, E., Zhu, J.-K., et al. (2007). Cold transiently activates calcium-permeable channels in Arabidopsis mesophyll cells. Plant Physiol. 143, 487–494. doi: 10.1104/pp.106.090928
Carroll, A. D., Moyen, C., Van Kesteren, P., Tooke, F., Battey, N. H., and Brownlee, C. (1998). Ca2+, annexins, and GTP modulate exocytosis from maize root cap protoplasts. Plant Cell 10, 1267–1276. doi: 10.1105/tpc.10.8.1267
Catala, R., Santos, E., Alonso, J. M., Ecker, J. R., Martinez-Zapater, J. M., and Salinas, J. (2003). Mutations in the Ca2+/H+ transporter CAX1 increase CBF/DREB1 expression and the cold-acclimation response in Arabidopsis. Plant Cell 15, 2940–2951. doi: 10.1105/tpc.015248
Chehab, E. W., Eich, E., and Braam, J. (2009). Thigmomorphogenesis: a complex plant response to mechanostimulation. J. Expt. Bot. 60, 43–56. doi: 10.1093/jxb/ern315
Cheng, N. H., Pittman, J. K., Zhu, J. K., and Hirschi, K. D. (2004). The protein kinase SOS2 activates the Arabidopsis H+/Ca2+ antiporter CAX1 to integrate calcium transport and salt tolerance. J. Biol. Chem. 279, 2922–2926. doi: 10.1074/jbc.M309084200
Cheong, Y. H., Pandey, G. K., Grant, J. J., Batistic, O., Li, L., Kim, B.-G., et al. (2007). Two calcineurin B-like calcium sensors, interacting with protein kinase CIPK23, regulate leaf transcription and root potassium uptake in Arabidopsis. Plant J. 52, 223–239. doi: 10.1111/j.1365-313X.2007.03236.x
Chmielowska-Bak, J., Gryl, J., Rucinska-Sobkowiak, R., Arasimowicz-Jelonek, M., and Deckert, J. (2014). The new insights into cadmium sensing. Front. Plant Sci. 5:245. doi: 10.3389/fpls.2014.00245
Choi, W.-G., Toyota, M., Kim, S.-H., Hilleary, R., and Gilroy, S. (2014). Salt stress-induced Ca2+ waves are associated with rapid, long-distance root-to-shoot signaling in plants. Proc. Natl. Acad. Sci. U.S.A. 111, 6497–6502. doi: 10.1073/pnas.1319955111
Christensen, A., Svensson, K., Persson, S., Jung, J., Michalak, M., Widell, S., et al. (2008). Functional characterization of Arabidopsis calreticulin1a: A key alleviator of endoplasmic reticulum. Plant Cell Physiol. 49, 912–924. doi: 10.1093/pcp/pcn065
Chung, J. S., Zhu, J. K., Bressan, R. A., Hasagawa, P. M., and Shi, H. (2008). Reactive oxygen species mediate Na+-induced SOS1 mRNA stability in Arabidopsis. Plant J. 53, 554–565. doi: 10.1111/j.1365-313X.2007.03364.x
Clemens, S., Antosiewicz, D. M., Ward, J. M., Schachtman, D. B., and Schroeder, J. I. (1998). The plant cDNA LCT1 mediates the uptake of calcium and cadmium in yeast. Proc. Natl. Acad. Sci. U.S.A. 95, 12043–12048. doi: 10.1073/pnas.95.20.12043
Connorton, J. M., Webster, R. E., Cheng, N. H., and Pittman, J. K. (2012). Knockout of multiple Arabidopsis cation/H+ exchangers suggests isoform-specific roles in metal stress response, germination and see mineral nutrition. PLoS ONE 7:e47455. doi: 10.1371/journal.pone.0047455
Dark, A. M., Demidchik, V., Richards, S. L., Shabala, S. N., and Davies, J. M. (2011). Release of extracellular purines from plant roots and effect on ion fluxes. Plant Signal. Behav. 6, 1855–1857. doi: 10.4161/psb.6.11.17014
Davies, J. M. (2014). Annexin-mediated calcium signaling in plants. Plants 3, 128–140. doi: 10.3390/plants3010128
Demidchik, V., Bowen, H. C., Maathuis, F. J. M., Shabala, S. N., Tester, M. A., White, P. J., et al. (2002). Arabidopsis thaliana root non-selective cation channels mediate calcium uptake and are involved in growth. Plant J. 32, 799–808. doi: 10.1046/j.1365-313X.2002.01467.x
Demidchik, V., Cuin, T. A., Svistunenko, D., Smith, S. J., Miller, A. J., Shabala, S., et al. (2010). Arabidopsis root K+-efflux conductance activated by hydroxyl radicals: single-channel properties, genetic basis and involvement in stress-induced death. J. Cell Sci. 123, 1468–1479. doi: 10.1242/jcs.064352
Demidchik, V., Shabala, S. N., Coutts, K. B., Tester, M. A., and Davies, J. M. (2003). Free oxygen radicals regulate plasma membrane Ca2+ and K+-permeable channels in plant root cells. J. Cell Sci. 116, 81–88. doi: 10.1242/jcs.00201
Demidchik, V., Shabala, S. N., and Davies, J. M. (2007). Spatial variation in H2O2 response of Arabidopsis thaliana epidermal Ca2+ flux and plasma membrane Ca2+ channels. Plant J. 49, 377–386. doi: 10.1111/j.1365-313X.2006.02971.x
Demidchik, V., Shang, Z., Shin, R., Thompson, E. P., Rubio, L., Laohavisit, A., et al. (2009). Plant extracellular ATP signalling by plasma membrane NADPH oxidase and Ca2+ channels. Plant J. 58, 903–913. doi: 10.1111/j.1365-313X.2009.03830.x
Deng, Y., Srivatava, R., and Howell, S. H. (2013). Protein kinase and ribonuclease domians of IRE1 confer stress tolerance, vegetative growth and reproductive development. Proc. Natl. Acad. Sci. U.S.A. 110, 19633–19638. doi: 10.1073/pnas.1314749110
DeWald, D. B., Torabinejad, J., Jones, C. A., Shope, J. C., Cangelosi, A. R., Thompson, J. E., et al. (2001). Rapid accumulation of phosphatidylinositol 4,5-bisphosphate and inositol 1,4,5-trisphosphate correlates with calcium mobilization in salt-stressed Arabidopsis. Plant Physiol. 126, 759–769. doi: 10.1104/pp.126.2.759
Dinneny, J. R., Long, T. A., Wang, J. Y., Jung, J. W., Mace, D., Pointer, S., et al. (2008). Cell identity mediates the response of Arabidopsis roots to abiotic stress. Science 320, 942–945. doi: 10.1126/science.1153795
Doherty, C. J., Van Buskirk, H. A., Myers, S. J., and Thomashow, M. F. (2009). Roles for Arabidopsis CAMTA transcription factors in cold-regulated gene expression and freezing tolerance. Plant Cell 21, 972–984. doi: 10.1105/tpc.108.063958
Donaldson, L., Ludidi, N., Knight, M. R., Gehring, C., and Denby, K. (2004). Salt and osmotic stress cause rapid increases in Arabidopsis thaliana cGMP levels. FEBS Letts. 569, 317–320. doi: 10.1016/j.febslet.2004.06.016
Drerup, M. M., Schluecking, K., Hashimoto, K., Manishankar, P., Steinhorst, L., Kuchitsu, K., et al. (2013). The Calcineurin B-Like calcium sensors CBL1 and CBL9 together with their interacting protein kinase CIPK26 regulate the Arabidopsis NADPH oxidase RBOHF. Mol. Plant 6, 559–569. doi: 10.1093/mp/sst009
Dressler, L., Golbik, R., and Ulbrich-Hofmann, R. (2014). Lanthanides as substitutes for calcium ions in the activation of plant alpha-type phospholipase D. Biol. Chem. 395, 791–799. doi: 10.1515/hsz-2014-0112
Eremina, M., Rozhon, W., and Poppenberger, B. (2016). Hormonal control of cold stress responses in plants. Cell. Mol. Life Sci. 73, 797–810. doi: 10.1007/s00018-015-2089-6
Fagerstedt, K. V. (2010). “Programmed cell death and aerenchyma formation under hypoxia,” in Waterlogging Signalling and Tolerance in Plants, eds S. Mancuso and S. Shabala (Berlin: Springer), 99–118.
Fan, J.-L., Wei, X.-Z., Wan, L.-C., Zhang, L.-Y., Zhao, X.-Q., Liu, W.-Z., et al. (2011). Disarrangement of actin filaments and Ca2+ gradient by CdCl2 alters cell wall construction in Arabidopsis thaliana root hairs by inhibiting vesicular trafficking. J. Plant Physiol. 168, 1157–1167. doi: 10.1016/j.jplph.2011.01.031
Fischer, C., Kugler, A., Hoth, S., and Dietrich, P. (2013). An IQ domain mediates the interaction with calmodulin in a plant cyclic nucleotide-gated channel. Plant Cell Physiol. 54, 573–584. doi: 10.1093/pcp/pct021
Foreman, J., Demidchik, V., Bothwell, J. H. F., Mylona, P., Miedema, H., Torres, M. A., et al. (2003). Reactive oxygen species produced by NADPH oxidase regulate plant cell growth. Nature 422, 442–446. doi: 10.1038/nature01485
Fry, S. C., Miller, J. G., and Dumville, J. C. (2002). A proposed role for copper ions in cell wall loosening. Plant Soil 247, 57–67. doi: 10.1023/A:1021140022082
Furuya, T., Matsuoka, D., and Nanmori, T. (2013). Phosphorylation of Arabidopsis thaliana MEKK1 via Ca2+ signaling as a part of the cold stress response. J. Plant Res. 126, 833–840. doi: 10.1007/s10265-013-0576-0
Furuya, T., Matsuoka, D., and Nanmori, T. (2014). Membrane rigidification functions upstream of the MEKK1-MKK2-MPK4 cascade during cold acclimation in Arabidopsis thaliana. FEBS Lett. 588, 2025–2030. doi: 10.1016/j.febslet.2014.04.032
Gobert, A., Park, G., Amtmann, A., Sanders, D., and Maathuis, F. J. M. (2006). Arabidopsis thaliana cyclic nucleotide-gated channel 3 forms a non-selective ion transporter involved in germination and cation transport. J. Expt. Bot. 57, 791–800. doi: 10.1093/jxb/erj064
Goldbach, H. E., Yu, Q., Wingender, R., Schulz, M., Wimmer, M., Findeklee, P., et al. (2001). Rapid response reactions of roots to boron deprivation. J. Plant Nutr. Soil Sci. 164, 173–181. doi: 10.1002/1522-2624(200104)164:2<173::AID-JPLN173>3.3.CO;2-6
González-Fontes, A., Herrera-Rodriguez, M. B., Martin-Rejano, E. M., Navarro-Gochicoa, M. T., Rexach, J., and Camacho-Cristobal, J. J. (2016). Root responses to boron deficiency mediated by ethylene. Front. Plant Sci. 6:1103. doi: 10.3389/fpls.2015.01103
Gonzali, S., Loreti, E., Cardarelli, F., Novi, G., Parlanti, S., Pucciariello, C., et al. (2015). Universal stress protein HRU1 mediates ROS homeostasis under anoxia. Nat. Plants 1, 15151. doi: 10.1038/NPLANTS.2015.151
Guerra, F., Duplessis, S., Kohler, A., Martin, F., Tapia, J., Lebed, P., et al. (2009). Gene expression analysis of Populus deltoides roots subjected to copper stress. Environ. Exp. Bot. 67, 335–344. doi: 10.1016/j.envexpbot.2009.08.004
Guo, J., Zeng, W., Chen, Q., Lee, C., Chen, L., Yang, Y., et al. (2016). Structure of the voltage-gated two-pore channel TPC1 from Arabidopsis thaliana. Nature 531, 196–201. doi: 10.1038/nature16446
Guo, K.-M., Babourina, O., Christopher, D. A., Borsics, T., and Rengel, Z. (2008). The cyclic nucleotide-gated channel, AtCNGC10, influences salt tolerance in Arabidopsis. Physiol. Plant. 134, 499–507. doi: 10.1111/j.1399-3054.2008.01157.x
Haswell, E. S., Peyronnet, R., Barbier-Brygoo, H., Meyerowitz, E. M., and Frachisse, J. M. (2008). Two MscS homologs provide mechanosensitive channel activities in the Arabidopsis root. Curr. Biol. 18, 730–734. doi: 10.1016/j.cub.2008.04.039
Ho, C.-H., Lin, S.-H., Hu, H.-C., and Tsay, Y.-F. (2009). CHL1 functions as a nitrate sensor in plants. Cell 138, 1184–1194. doi: 10.1016/j.cell.2009.07.004
Hossain, M. A., Henriquez-Valencia, C., Gómez-Páez, M., Medina, J., Orellana, A., Vicente-Carbajosa, J., et al. (2016). Identification of novel components of the unfolded protein response in Arabidopsis. Front. Plant Sci. 7:650. doi: 10.3389/fpls.2016.00650
Hou, C. C., Tian, W., Kleist, T., He, K., Garcia, V., Bai, F., et al. (2014). DUF221 proteins are a family of osmosensitive calcium-permeable cation channels conserved across eukaryotes. Cell Res. 24, 632–635. doi: 10.1038/cr.2014.14
Hou, Q., Ufer, G., and Bartels, D. (2016). Lipid signalling in plant responses to abiotic stress. Plant Cell Environ. 39, 1029–1048. doi: 10.1111/pce.12666
Hu, H. C., Wang, Y. Y., and Tsay, Y. F. (2009). AtCIPK8, a CBL-interacting protein kinase, regulates the low-affinity phase of the primary nitrate response. Plant J. 57, 264–278. doi: 10.1111/j.1365-313X.2008.03685.x
Hu, Y. F., Zhou, G., Na, X. F., Yang, L., Nan, W. B., Liu, X., et al. (2013). Cadmium interferes with maintenance of auxin homeostasis in Arabidopsis seedlings. J. Plant Physiol. 170, 965–975. doi: 10.1016/j.jplph.2013.02.008
Hua, B. G., Mercier, R. W., and Zielinski, R. E. (2003). Functional interaction of calmodulin with a plant cyclic nucleotide gated cation channel. Plant Physiol. Biochem. 41, 945–954. doi: 10.1016/j.plaphy.2003.07.006
Huang, T.-L., and Huang, H.-J. (2008). ROS and CDPK-like kinase-mediated activation of MAP kinase in rice roots exposed to lead. Chemosphere 71, 1377–1385. doi: 10.1016/j.chemosphere.2007.11.031
Huang, W.-C., Huang, D.-D., Chien, P.-S., Yeh, C.-M., Chen, P.-Y., Chi, W.-C., et al. (2007). Protein tyrosine dephosphorylation during copper-induced cell death in rice roots. Chemosphere 69, 55–62. doi: 10.1016/j.chemosphere.2007.04.073
Hunt, L., Otterhag, L., Lee, J. C., Lasheen, T., Hunt, J., Seki, M., et al. (2004). Gene-specific expression and calcium activation of Arabidopsis thaliana phospholipase C isoforms. New Phytol. 162, 643–654. doi: 10.1111/j.1469-8137.2004.01069.x
Isner, J. C., Nühse, T., and Maathuis, F. J. M. (2012). The cyclic nucleotide cGMP is involved in plant hormone signalling and alters phosphorylation of Arabidopsis thaliana root proteins. J. Expt. Bot. 63, 3199–3205. doi: 10.1093/jxb/ers045
Jakubowska, D., Janicka-Russak, M., Kabala, K., Migocka, M., and Reda, M. (2015). Modification of plasma membrane NADPH oxidase activity in cucumber seedling roots in response to cadmium stress. Plant Sci. 234, 50–59. doi: 10.1016/j.plantsci.2015.02.005
Jeon, J., and Kim, J. (2013). Cold stress signaling networks in Arabidopsis. J. Plant Biol. 56, 69–76. doi: 10.1007/s12374-013-0903-y
Jiang, H. B., Wang, S. F., Yang, F., Zhang, Z. H., Qiu, H. C., Yi, Y., et al. (2015). Plant growth, nitrate content and Ca signaling in wheat (Triticum aestivum L.) roots under different nitrate supply. Plant Sci. J. 33, 362–368.
Jiao, Y., Sun, L., Song, Y., Wang, L., Liu, L., Zhang, L., et al. (2013). AtrbohD and AtrbohF positively regulate abscisic acid-inhibited primary root growth by affecting Ca2+ signalling and auxin response of roots in Arabidopsis. J. Expt. Bot. 64, 4183–4192. doi: 10.1093/jxb/ert228
Jin, Y. K., Jing, W., Zhang, Q., and Zhang, W. H. (2015). Cyclic nucleotide gated channel 10 negatively regulates salt tolerance by mediating Na+ transport in Arabidopsis. J. Plant Res. 128, 211–220. doi: 10.1007/s10265-014-0679-2
Kamano, S., Kume, S., Iida, K., Lei, K.-J., Nakano, M., Nakayama, Y., et al. (2015). Transmembrane topologies of Ca2+-permeable mechanosensitive channels MCA1 and MCA2 in Arabidopsis thaliana. J. Biol. Chem. 290, 30901–30909. doi: 10.1074/jbc.M115.692574
Kanehara, K., Yu, C.-Y., Cho, Y., Cheong, W.-F., Torta, F., Shui, G., et al. (2015). Arabidopsis AtPLC2 is a primary phosphoinositide-specific phospholipase C in phopshoinositide metabolism and the endoplasmic reticulum stress response. PLOS Genet. 11:e1005511. doi: 10.1371/journal.pgen.1005511
Kasajima, I., Ide, Y., Hirai, M. Y., and Fujiwara, T. (2010). WRKY6 is involved in the response to boron deficiency in Arabidopsis thaliana. Physiol. Plant. 139, 80–92. doi: 10.1111/j.1399-3054.2010.01349.x
Kawarazaki, T., Kimura, S., Iizuka, A., Hanamata, S., Nibori, H., Michikawa, M., et al. (2013). A low temperature-inducible protein AtSRC2 enhances the ROS-producing activity of NADPH oxidase AtRbohF. Biochim. Biophys. Acta Mol. Cell Res. 1833, 2775–2780. doi: 10.1016/j.bbamcr.2013.06.024
Kiegle, E., Moore, C. A., Haseloff, J., Tester, M. A., and Knight, M. R. (2000). Cell-type-specific calcium responses to drought, salt and cold in the Arabidopsis root. Plant J. 23, 267–278. doi: 10.1046/j.1365-313x.2000.00786.x
Kim, K. N., Cheong, Y. H., Grant, J. J., Pandey, G. K., and Luan, S. (2003). CIPK3, a calcium sensor-associated protein kinase that regulates abscisic acid and cold signal transduction in Arabidopsis. Plant Cell 15, 411–423. doi: 10.1105/tpc.006858
Kim, Y., Wang, M., Bai, Y., Zeng, Z., Guo, F., Han, N., et al. (2014). Bcl-2 suppresses activation of VPEs by inhibiting cytosolic Ca2+ level with elevated K+ efflux in NaCl-induced PCD in rice. Plant Physiol. Biochem. 80, 168–175. doi: 10.1016/j.plaphy.2014.04.002
Kintzer, A. F., and Stroud, R. M. (2016). Structure, inhibition and regulation of two-pore channel TPC1 from Arabidopsis thaliana. Nature 531, 258–264. doi: 10.1038/nature17194
Knight, H., and Knight, M. R. (2000). Imaging spatial and cellular characteristics of low temperature calcium signature after cold acclimation in Arabidopsis. J. Expt. Bot. 51, 1679–1686. doi: 10.1093/jexbot/51.351.1679
Knight, H., Trewavas, A. J., and Knight, M. R. (1996). Cold calcium signaling in Arabidopsis involves two cellular pools and a change in calcium signature after acclimation. Plant Cell 8, 489–503. doi: 10.1105/tpc.8.3.489
Knight, H., Trewavas, A. J., and Knight, M. R. (1997). Calcium signalling in Arabidopsis thaliana responding to drought and salinity. Plant J. 12, 1067–1078. doi: 10.1046/j.1365-313X.1997.12051067.x
Knight, M. R., and Knight, H. (2012). Low-temperature perception leading to gene expression and cold tolerance in higher plants. New Phytol. 195, 737–751. doi: 10.1111/j.1469-8137.2012.04239.x
Kobayashi, B., Matoh, T., and Azuma, J. (1996). Two chains of rhamnogalacturonan II are cross-linked by borate-diol ester bonds in higher plant cell walls. Plant Physiol. 110, 1017–1020.
Kolbert, Z., Petö, A., Lehotai, N., Feigl, G., and Erdei, L. (2012). Long-term copper (Cu2+) exposure impacts on auxin, nitric oxide (NO) metabolism and morphology of Arabidopsis thaliana L. Plant Growth Regul. 68, 151–159. doi: 10.1007/s10725-012-9701-7
Koshiba, T., Ishihara, A., and Matoh, T. (2010). Boron nutrition of cultured Tobacco BY-2 Cells. VI. Calcium is involved in early responses to boron deprivation. Plant Cell Physiol. 51, 323–327. doi: 10.1093/pcp/pcp179
Kugler, A., Koehler, B., Palme, K., Wolff, P., and Dietrich, P. (2009). Salt-dependent regulation of a CNG channel subfamily in Arabidopsis. BMC Plant Biol. 9:140. doi: 10.1186/1471-2229-9-140
Kurusu, T., Nishikawa, D., Yamazaki, Y., Gotoh, M., Nakano, M., Hamada, H., et al. (2012). Plasma membrane protein OsMCA1 is involved in regulation of hypo-osmotic shock-induced Ca2+ influx and modulates generation of reactive oxygen species in cultured rice cells. BMC Plant Biol. 12:11. doi: 10.1186/1471-2229-12-11
Laohavisit, A., and Davies, J. M. (2011). Annexins. New Phytol. 189, 40–53. doi: 10.1111/j.1469-8137.2010.03533.x
Laohavisit, A., Richards, S. L., Shabala, L., Chen, C., Colaço, R., Swarbreck, S. M., et al. (2013). Salinity-induced calcium signaling and root adaptation in Arabidopsis require the regulatory protein annexin1. Plant Physiol. 163, 253–262. doi: 10.1104/pp.113.217810
Laohavisit, A., Shang, Z.-L., Rubio, L., Cuin, T. A., Véry, A. A., Wang, A. H., et al. (2012). Arabidopsis annexin 1 mediates the radical-activated plasma membrane Ca2+ and K+-permeable conductance in root cells. Plant Cell 24, 1522–1533. doi: 10.1105/tpc.112.097881
Latz, A., Mehlmer, N., Zapf, S., Mueller, T. D., Wurzinger, B., Pfister, B., et al. (2013). Salt stress triggers phosphorylation of the Arabidopsis vacuolar K+ channel TPK1 by calcium-dependent protein kinases (CDPKs). Mol. Plant 6, 1274–1289. doi: 10.1093/mp/sss158
Legue, V., Blancaflor, E., Wymer, C., Perbal, G., Fahtin, D., and Gilroy, S. (1997). Cytoplasmic free calcium in Arabidopsis roots changes in response to touch but not gravity. Plant Physiol. 114, 789–800. doi: 10.1104/pp.114.3.789
Lequeux, H., Hermans, C., Lutts, S., and Verbruggen, N. (2010). Response to copper excess in Arabidopsis thaliana: impact on the root system architecture, hormone distribution, lignin accumulation and mineral profile. Plant Physiol. Biochem. 48, 673–682. doi: 10.1016/j.plaphy.2010.05.005
Léran, S., Edel, K. H., Pervent, M., Hashimoto, K., Corratge-Faillie, C., Offenborn, J. N., et al. (2015). Nitrate sensing and uptake in Arabidopsis are enhanced by ABI2, a phosphatase inactivated by the stress hormone abscisic acid. Sci. Signal. 8, ra43. doi: 10.1126/scisignal.aaa4829
Li, G., Boudsocq, M., Hem, S., Vialaret, J., Rossignol, M., Maurel, C., et al. (2015). The calcium-dependent protein kinase CPK7 acts on root hydraulic conductivity. Plant Cell Environ. 38, 1312–1320. doi: 10.1111/pce.12478
Li, J., Long, Y., Qi, G. N., Xu, Z. J., Wu, W. H., and Wang, Y. (2014). The OsAKT1 channel is critical for K+ uptake in rice roots and is modulated by the rice CBL1-CIPK23 complex. Plant Cell 26, 3387–3402. doi: 10.1105/tpc.114.123455
Li, L., Kim, B.-G., Cheong, Y. H., Pandey, G. K., and Luan, S. (2006). A Ca2+ signalling pathway regulates a K+ channel for low K+ response in Arabidopsis. Proc. Natl. Acad. Sci. U.S.A. 103, 12625–12630. doi: 10.1073/pnas.0605129103
Li, P., Zhao, C. Z., Zhang, Y. Q., Wang, X. M., Wang, X. Y., Wang, J. F., et al. (2016). Calcium alleviates cadmium-induced inhibition on root growth by maintaining auxin homeostasis in Arabidopsis seedlings. Protoplasma 253, 185–200. doi: 10.1007/s00709-015-0810-9
Li, P. H., Zhang, G. Y., Gonzales, N., Guo, Y. Q., Hu, H. H., Park, S. H., et al. (2016). Ca2+-regulated and diurnal rhythm-regulated Na+/Ca2+ exchanger AtNCL affects flowering time and auxin signalling in Arabidopsis. Plant Cell Environ. 39, 377–392. doi: 10.1111/pce.12620
Li, S., Yu, J., Zhu, M., Zhao, F., and Luan, S. (2012). Cadmium impairs ion homeostasis by altering K+ and Ca2+ channel activities in rice root hairs. Plant Cell Environ. 35, 1998–2013. doi: 10.1111/j.1365-3040.2012.02532.x
Lin, C. Y., Trinh, N. N., Fu, S. F., Hsiung, Y. C., Chia, L. C., Lin, C. W., et al. (2013). Comparison of early transcriptome responses to copper and cadmium in rice roots. Plant Mol. Biol. 81, 507–522. doi: 10.1007/s11103-013-0020-9
Liu, J. P., Ishitani, M., Halfter, U., Kim, C. S., and Zhu, J. K. (2000). The Arabidopsis thaliana SOS2 gene encodes a protein kinase that is required for salt tolerance. Proc. Natl. Acad. Sci. U.S.A. 97, 3730–3734. doi: 10.1073/pnas.97.7.3730
Liu, J.-X., Srivastava, R., Che, P., and Howell, S. H. (2007). Salt stress responses in Arabidopsis utilize a signal transduction pathway related to endoplasmic reticulum stress signaling. Plant J. 51, 897–909. doi: 10.1111/j.1365-313X.2007.03195.x
Liu, L., Li, Q., Yin, B., Zhang, H., Lin, B., Wu, Y., et al. (2011). The endoplasmic reticulum-associated degradation is necessary for plant salt tolerance. Cell Res. 21, 957–969. doi: 10.1038/cr.2010.181
Liu, Q., Zheng, L., He, F., Zhao, F.-J., Shen, Z., and Zheng, L. (2015). Transcriptional and physiological analyses identify a regulatory role for hydrogen peroxide in the lignin biosynthesis of copper-stressed rice roots. Plant Soil 387, 323–336. doi: 10.1007/s11104-014-2290-7
Lokdarshi, A., Conner, W. C., McClintock, C., Li, T., and Roberts, D. M. (2016). Arabidopsis CML38, a calcium sensor that localizes to ribonucleoprotein complexes under hypoxia stress. Plant Physiol. 170, 1046–1059. doi: 10.1104/pp.15.01407
Lu, G., Wang, X., Liu, J., Yu, K., Gao, Y., Liu, H., et al. (2014). Application of T-DNA activation tagging to identify glutamate receptor-like genes that enhance drought tolerance in plants. Plant Cell Rep. 33, 617–631. doi: 10.1007/s00299-014-1586-7
Lyalin, O. O., and Ktitorova, I. N. (1969). Resting potential of the root hair of Trianea bogotensis. Sov. Plant Phys. 16, 214–221.
Ma, L., Zhang, H., Sun, L., Jiao, Y., Zhang, G., Miao, C., et al. (2012). NADPH oxidase AtrbohD and AtrbohF function in ROS-dependent regulation of Na+/K+ homeostasis in Arabidopsis under salt stress. J. Expt. Bot. 63, 305–317. doi: 10.1093/jxb/err280
Ma, Q., Tang, R. J., Zheng, X. J., Wang, S. M., and Luan, S. (2015). The calcium sensor CBL7 modulates plant responses to low nitrate in Arabidopsis. Biochem. Biophys. Res. Commun. 468, 59–65. doi: 10.1016/j.bbrc.2015.10.164
Ma, Y., Dai, X. Y., Xu, Y. Y., Luo, W., Zheng, X. M., Zeng, D. L., et al. (2015). COLD1 confers chilling tolerance in rice. Cell 160, 1209–1221. doi: 10.1016/j.cell.2015.01.046
Maathuis, F. J. M. (2014). Sodium in plants: perception, signalling and regulation of sodium fluxes. J. Expt. Bot. 65, 849–858. doi: 10.1093/jxb/ert326
Mazars, C., Thion, L., Thuleau, P., Graziana, A., Knight, M. R., Moreau, M., et al. (1997). Organization of cytoskeleton controls the changes in cytosolic calcium of cold-shocked Nicotiana plumbaginifolia protoplasts. Cell Calcium 22, 413–420. doi: 10.1016/S0143-4160(97)90025-7
McAinsh, M. R., and Pittman, J. K. (2009). Shaping the calcium signature. New Phytol. 181, 275–294. doi: 10.1111/j.1469-8137.2008.02682.x
McLoughlin, F., Galvan-Ampudia, C. S., Julkowska, M. M., Caarls, L., van der Does, D., Lauriere, C., et al. (2012). The Snf1-related protein kinases SnRK2.4 and SnRK2.10 are involved in maintenance of root system architecture during salt stress. Plant J. 72, 436–449. doi: 10.1111/j.1365-313X.2012.05089.x
Mehlmer, N., Wurzinger, B., Stael, S., Hofmann-Rodrigues, D., Csaszar, E., Pfister, B., et al. (2010). The Ca2+-dependent protein kinase CPK3 is required for MAPK-independent salt-stress acclimation in Arabidopsis. Plant J. 63, 484–498. doi: 10.1111/j.1365-313X.2010.04257.x
Mei, H., Cheng, N. H., Zhao, J., Park, S., Escareno, R. A., Pittman, J. K., et al. (2009). Root development under metal stress in Arabidopsis thaliana requires the H+/cation antiporter CAX4. New Phytol. 183, 95–105. doi: 10.1111/j.1469-8137.2009.02831.x
Meringer, M. V., Villasuso, A. L., Peppino, M. M., Usorach, J., Pasquare, S. J., Giusto, N. M., et al. (2016). Saline and osmotic stresses stimulate PLD/diacylglycerol kinase activities and increase the level of phosphatidic acid and proline in barley roots. Environ. Expt. Bot. 128, 69–78. doi: 10.1016/j.envexpbot.2016.03.011
Miedema, H., Demidchik, V., Véry, A.-A., Bothwell, J. H. F., Brownlee, C., and Davies, J. M. (2008). Two voltage-dependent calcium channels co-exist in the apical plasma membrane of Arabidopsis thaliana root hairs. New Phytol. 179, 378–385. doi: 10.1111/j.1469-8137.2008.02465.x
Miller, G., Schlauch, K., Tam, R., Cortes, D., Torres, M. A., Shulaev, V., et al. (2009). The plant NADPH oxidase RBOHD mediates rapid systemic signaling in response to diverse stimuli. Sci. Signal. 2, ra45. doi: 10.1126/scisignal.2000448
Minorsky, P. V., and Spanswick, R. M. (1989). Electrophysiological evidence for a role for calcium in temperature sensing by roots of cucumber seedlings. Plant Cell Env. 12, 137–143. doi: 10.1111/j.1365-3040.1989.tb01925.x
Monshausen, G. B., Bibikova, T. N., Weisenseel, M. H., and Gilroy, S. (2009). Ca2+ regulates reactive oxygen species production and pH during mechanosensing in Arabidopsis roots. Plant Cell 21, 2341–2356. doi: 10.1105/tpc.109.068395
Moore, C. A., Bowen, H. C., Scrase-Field, S., Knight, M. R., and White, P. J. (2002). The deposition of suberin lamellae determines the magnitude of cytosolic Ca2+ elevations in root endodermal cells subjected to cooling. Plant J. 30, 457–465. doi: 10.1046/j.1365-313X.2002.01306.x
Mortimer, J. C., Laohavisit, A., Miedema, H., and Davies, J. M. (2008). Voltage, reactive oxygen species and the influx of calcium. Plant Sig. Behav. 3, 698–699. doi: 10.4161/psb.3.9.6405
Mühling, K. H., Wimmer, M., and Goldbach, H. E. (1998). Apoplastic and membrane associated Ca2+ in leaves and roots as affected by boron deficiency. Physiol. Plant. 102, 179–184. doi: 10.1034/j.1399-3054.1998.1020204.x
Nakagawa, Y., Katagiri, T., Shinozaki, K., Qi, Z., Tatsumi, H., Furuichi, T., et al. (2007). Arabidopsis plasma membrane protein crucial for Ca2+ influx and touch sensing in roots. Proc. Natl. Acad. Sci. U.S.A. 104, 3639–3644. doi: 10.1073/pnas.0607703104
Nieves-Cordones, M., Miller, A. J., Aleman, F., Martinez, V., and Rubio, F. (2008). A putative role for the plasma membrane potential in the control of the expression of the gene encoding the tomato high-affinity potassium transporter HAK5. Plant Mol. Biol. 68, 521–532. doi: 10.1007/s11103-008-9388-3
Oiwa, Y., Kitayama, K., and Matoh, T. (2013). Boron deprivation immediately causes cell death in growing roots of Arabidopsis thaliana (L.) Heynh. Soil Sci. Plant Nutr. 59, 621–627. doi: 10.1080/00380768.2013.813382
Ordenes, V. R., Moreno, I., Maturana, D., Norambuena, L., Trewavas, A. J., and Orellana, A. (2012). In vivo analysis of the calcium signature in the plant Golgi apparatus reveals unique dynamics. Cell Calcium 52, 397–404. doi: 10.1016/j.ceca.2012.06.008
Orvar, B. L., Sangwan, V., Omann, F., and Dhindsa, R. S. (2000). Early steps in cold sensing by plant cells: the role of actin cytoskeleton and membrane fluidity. Plant J. 23, 785–794. doi: 10.1046/j.1365-313x.2000.00845.x
Pandey, N., Ranjan, A., Pant, P., Tripathi, R. K., Ateek, F., Pandey, H. P., et al. (2013). CAMTA 1 regulates drought responses in Arabidopsis thaliana. BMC Genomics 14:216. doi: 10.1186/1471-2164-14-216
Peiter, E., Maathuis, F. J. M., Mills, L. N., Knight, H., Pelloux, J., Hetherington, A. M., et al. (2005). The vacuolar Ca2+-activated channel TPC1 regulates germination and stomatal movement. Nature 434, 404–408. doi: 10.1038/nature03381
Perfus-Barbeoch, L., Leonhardt, N., Vavasseur, A., and Forestier, C. (2002). Heavy metal toxicity: cadmium permeates through calcium channels and disturbs the plant water status. Plant J. 32, 539–548. doi: 10.1046/j.1365-313X.2002.01442.x
Petrov, V., Hille, J., Mueller-Roeber, B., and Gechev, T. S. (2015). ROS-mediated abiotic stress-induced programmed cell death in plants. Front. Plant Sci. 6:69. doi: 10.3389/fpls.2015.00069
Plieth, C., Hansen, U. P., Knight, H., and Knight, M. R. (1999). Temperature sensing by plants: the primary characteristics of signal perception and calcium response. Plant J. 18, 491–497. doi: 10.1046/j.1365-313X.1999.00471.x
Pottosin, I., Maria Velarde-Buendia, A., Bose, J., Fuglsang, A. T., and Shabala, S. (2014). Polyamines cause plasma membrane depolarization, activate Ca2+-, and modulate H+-ATPase pump activity in pea roots. J. Expt. Bot. 65, 2463–2472. doi: 10.1093/jxb/eru133
Probst, A. V., and Scheid, O. M. (2015). Stress-induced structural changes in plant chromatin. Curr. Opin. Plant Biol. 27, 8–16. doi: 10.1016/j.pbi.2015.05.011
Qin, W. S., Pappan, K., and Wang, X. M. (1997). Molecular heterogeneity of phospholipase D (PLD) – Cloning of PLD gamma and regulation of plant PLD gamma, -beta, and -alpha by polyphosphoinositides and calcium. J. Biol. Chem. 272, 28267–28273. doi: 10.1074/jbc.272.45.28267
Qiu, Q. S., Guo, Y., Quintero, F. J., Pardo, J. M., Schumaker, K. S., and Zhu, J. K. (2004). Regulation of vacuolar Na+/H+ exchange in Arabidopsis thaliana by the salt-overly-sensitive (SOS) pathway. J. Biol. Chem. 279, 207–215. doi: 10.1074/jbc.M307982200
Quiles-Pando, C., Rexach, J., Navarro-Gochicoa, M. T., Camacho-Cristóbal, J. J., Herrera-Rodríguez, M. B., and González-Fontes, A. (2013). Boron deficiency increases the level of cytosolic Ca2+ and expression of Ca2+-related genes in Arabidopsis thaliana roots. Plant Physiol. Biochem. 65, 55–60. doi: 10.1016/j.plaphy.2013.01.004
Quintero, F. J., Martinez-Atienza, J., Villalta, I., Jiang, X., Kim, W. Y., Ali, Z., et al. (2011). Activation of the plasma membrane Na/H antiporter Salt-Overly-Sensitive 1 (SOS1) by phosphorylation of an auto-inhibitory C-terminal domain. Proc. Natl. Acad. Sci. U.S.A. 108, 2611–2616. doi: 10.1073/pnas.1018921108
Ragel, P., Rodenas, R., Garcia-Martin, E., Andres, Z., Villalta, I., Nieves-Cordones, M., et al. (2015). SOS the CBL-interacting protein kinase CIPK23 regulates HAK5-mediated high-affinity K+ uptake in Arabidopsis roots. Plant Physiol. 169, 2863–2873. doi: 10.1104/pp.15.01401
Richards, S. L., Wilkins, K. A., Swarbreck, S. M., Anderson, A., Habib, N., McAinsh, M., et al. (2015). The hydroxyl radical; from seed to seed. J. Expt. Bot. 66, 37–46. doi: 10.1093/jxb/eru398
Richter, G. L., Monshausen, G. B., Krol, A., and Gilroy, S. (2009). Mechanical stimuli modulate lateral root organogenesis. Plant Physiol. 151, 1855–1866. doi: 10.1104/pp.109.142448
Riveras, E., Alvarez, J. M., Vidal, E. A., Oses, C., Vega, A., and Gutierrez, R. A. (2015). The calcium ion is a second messenger in the nitrate signaling pathway of Arabidopsis. Plant Physiol. 169, 1397–1404. doi: 10.1104/pp.15.00961
Rodrigo-Moreno, A., Andres-Colas, N., Poschenrieder, C., Gunse, B., Penarrubia, L., and Shabala, S. (2013). Calcium- and potassium-permeable plasma membrane transporters are activated by copper in Arabidopsis root tips: linking copper transport with cytosolic hydroxyl radical production. Plant Cell Environ. 36, 844–855. doi: 10.1111/pce.12020
Rodriguez-Hernandez, M. C., Bonifas, I., Alfaro-De la Torre, M. C., Flores-Flores, J. I., Bañuelos-Hernández, B., and Patiño-Rodríguez, O. (2015). Increased accumulation of cadmium and lead under Ca and Fe deficiency in Typha latifolia: a case study of two pore channel (TPC1) gene. Environ. Exp. Bot. 115, 38–48. doi: 10.1016/j.envexpbot.2015.02.009
Roy, S. J., Gilliham, M., Berger, B., Essah, P. A., Cheffings, C., Miller, A. J., et al. (2008). Investigating glutamate receptor-like gene co-expression in Arabidopsis thaliana. Plant Cell Environ. 31, 861–871. doi: 10.1111/j.1365-3040.2008.01801.x
Ruberti, C., Kim, S. J., Stefano, G., and Brandizzi, F. (2015). Unfolded protein responses in plants: one master, many questions. Curr. Opin. Plant Biol. 27, 59–66. doi: 10.1016/j.pbi.2015.05.016
Ruelland, E., Kravets, V., Derevyanchuk, M., Martinec, J., Zachowski, A., and Pokotylo, I. (2015). Role of phospholipid signalling in plant environmental responses. Environ. Exp. Bot. 114, 129143. doi: 10.1016/j.envexpbot.2014.08.009
Sagor, G. H. M., Chawla, P., Kim, D. W., Berberich, T., Kojima, S., Niitsu, M., et al. (2015). The polyamine spermine induces the unfolded protein response via the MAPK cascade in Arabidopsis. Front. Plant Sci. 6:687. doi: 10.3389/fpls.2015.00687
Sani, E., Herzyk, P., Perrella, G., Colot, V., and Amtmann, A. (2013). Hyperosmotic priming of Arabidopsis seedlings establishes a long-term somatic memory accompanied by specific changes of the epigenome. Genome Biol. 14, R59. doi: 10.1186/gb-2013-14-6-r59
Sarwat, M., and Naqvi, A. R. (2013). Heterologous expression of rice calnexin (OsCNX) confers drought tolerance. Mol. Biol. Rep. 40, 5451–5464. doi: 10.1007/s11033-013-2643-y
Shabala, S., and Pottosin, I. (2014). Regulation of potassium transport in plants under hostile conditions: implications for abiotic and biotic stress tolerance. Physiol. Plant 151, 257–279. doi: 10.1111/ppl.12165
Shabala, S., Shabala, L., Barcelo, J., and Poschenrieder, C. (2014). Membrane transporters mediating root signalling and adaptive responses to oxygen deprivation and soil flooding. Plant Cell Environ. 37, 2216–2233. doi: 10.1111/pce.12339
Shabala, S., Wu, H., and Bose, J. (2015). Salt stress sensing and early signalling in plant roots: current knowledge and hypothesis. Plant Sci. 241, 109–119. doi: 10.1016/j.plantsci.2015.10.003
Shi, Y. T., Ding, Y. L., and Yang, S. H. (2015). Cold signal transduction and its interplay with phytohormones during cold acclimation. Plant Cell Physiol. 56, 7–15. doi: 10.1093/pcp/pcu115
Shih, H. W., Depew, C. L., Miller, N. D., and Monshausen, G. B. (2015). The cyclic nucleotide-gated channel CNGC14 regulates root gravitropism in Arabidopsis thaliana. Curr. Biol. 25, 1–7. doi: 10.1016/j.cub.2015.10.025
Shih, H.-W., Miller, N. D., Dai, C., Spalding, E. P., and Monshausen, G. B. (2014). The receptor-like kinase FERONIA is required for mechanical signal transduction in Arabidopsis seedlings. Curr. Biol. 24, 1887–1892. doi: 10.1016/j.cub.2014.06.064
Shin, R., and Schachtman, D. P. (2004). Hydrogen peroxide mediates plant root cell response to nutrient deprivation. Proc. Natl. Acad. Sci. U.S.A. 101, 8827–8832. doi: 10.1073/pnas.0401707101
Shorrocks, V. M. (1997). The occurrence and correction of boron deficiency. Plant Soil 193, 121–148. doi: 10.1023/A:1004216126069
Singh, S., Parihar, P., Singh, R., Singh, V. P., and Prasad, S. M. (2016). Heavy metal tolerance in plants: role of transcriptomics, proteomics, metabolomics, and ionomics. Front. Plant Sci. 6:1143. doi: 10.3389/fpls.2015.01143
Stael, S., Wurzinger, B., Mair, A., Mehlmer, N., Vothknecht, U. C., and Teige, M. (2012). Plant organellar calcium signalling: an emerging field. J. Expt. Bot. 63, 1525–1542. doi: 10.1093/jxb/err394
Straltsova, D., Chykun, P., Subramaniam, S., Sosan, A., Kolbanov, D., Sokolik, A., et al. (2015). cation channels are involved in brassinosteroid signalling in higher plants. Steroids 97, 98–106. doi: 10.1016/j.steroids.2014.10.008
Sulaiman, Y., Knight, M. R., and Kataky, R. (2012). Non-invasive monitoring of temperature stress in Arabidopsis thaliana roots, using ion amperometry. Anal. Meth. 4, 1656–1661. doi: 10.1039/c2ay05747f
Sunkar, R., Kaplan, B., Bouche, N., Arazi, T., Dolev, D., Talke, I. N., et al. (2000). Expression of a truncated tobacco NtCBP4 channel in transgenic plants and disruption of the homologous Arabidopsis CNGC1 gene confer Pb2+ tolerance. Plant J. 24, 533–542. doi: 10.1046/j.1365-313x.2000.00901.x
Swarbreck, S. M., Colaço, R., and Davies, J. M. (2013). Update on plant calcium-permeable channels. Plant Physiol. 163, 1514–1522. doi: 10.1104/pp.113.220855
Tai, F., Yuan, Z., Li, S. P., Wang, Q., Liu, F., and Wang, W. (2016). ZmCIPK8, a CBL-interacting protein kinase, regulates maize response to drought stress. Plant Cell Tiss. Org. Cul. 124, 459–469. doi: 10.1007/s11240-015-0906-0
Teardo, E., Carraretto, L., De Bortoli, S., Costa, A., Behera, S., and Wagner, R. (2015). Alternative splicing-mediated targeting of the Arabidopsis GLUTAMATE RECEPTOR3.5 to mitochondria affects organelle morphology. Plant Physiol. 167, 216–227. doi: 10.1104/pp.114.242602
Thion, L., Mazars, C., Nacry, P., Bouchez, D., Moreau, M., Ranjeva, R., et al. (1998). Plasma membrane depolarization-activated calcium channels, stimulated by microtubule-depolymerizing drugs in wild-type Arabidopsis thaliana protoplasts, display constitutively large activities and a longer half-life in ton 2 mutant cells affected in the organization of cortical microtubules. Plant J. 13, 603–610.
Thoday-Kennedy, E. L., Jacobs, A. J., and Roy, S. J. (2015). The role of the CBL-CIPK calcium signalling network in regulating ion transport in response to abiotic stress. Plant Growth Regul. 76, 3–12. doi: 10.1007/s10725-015-0034-1
Toyota, M., and Gilroy, S. (2013). Gravitropism and mechanical signalling in plants. Am. J. Bot. 100, 111–125. doi: 10.3732/ajb.1200408
Tracy, F. E., Gilliham, M., Dodd, A. N., Webb, A. A. R., and Tester, M. (2008). NaCl-induced changes in cytosolic free Ca2+ in Arabidopsis thaliana are heterogeneous and modified by external ionic composition. Plant Cell Environ. 31, 1063–1073. doi: 10.1111/j.1365-3040.2008.01817.x
Véry, A. A., and Davies, J. M. (2000). Hyperpolarization-activated calcium channels at the tip of Arabidopsis root hairs. Proc. Natl. Acad. Sci. U.S.A. 97, 9801–9806. doi: 10.1073/pnas.160250397
Villiers, F., Jourdain, A., Bastien, O., Leonhardt, N., Fujioka, S., Tichtincky, G., et al. (2012). Evidence for functional interaction between brassinosteroids and cadmium response in Arabidopsis thaliana. J. Expt. Bot. 63, 1185–1200. doi: 10.1093/jxb/err335
Virdi, A. S., Singh, S., and Singh, P. (2015). Abiotic stress responses in plants: roles of calmodulin-regulated proteins. Front. Plant Sci. 6:809. doi: 10.3389/fpls.2015.00809
Virolainen, E., Blokhina, O., and Fagerstedt, K. (2002). Ca2+-induced high amplitude swelling and cytochrome c release from wheat (Triticum aestivum L.) mitochondria under anoxic stress. Ann. Bot. 90, 509–516. doi: 10.1093/aob/mcf221
Wagner, S., Behera, S., De Bortoli, S., Logan, D. C., Fuchs, P., and Carraretto, L. (2015). The EF-Hand Ca2+-binding protein MICU choreographs mitochondrial Ca2+ dynamics in Arabidopsis. Plant Cell 27, 3190–3212. doi: 10.1105/tpc.15.00509
Wan, S., and Jiang, L. (2016). Endoplasmic reticulum (ER) stress and the unfolded protein response (UPR) in plants. Protoplasma 273, 753–764. doi: 10.1007/s00709-015-0842-1
Wang, F. F., Chen, Z. H., Liu, X. H., Colmer, T. D., Zhou, M. X., and Shabala, S. (2016). Tissue-specific root ion profiling reveals essential roles of the CAX and ACA calcium transport systems in response to hypoxia in Arabidopsis. J. Expt. Bot. 67, 3747–3762. doi: 10.1093/jxb/erw034
Wang, P., Li, Z. W., Wei, J. S., Zhao, Z. L., Sun, D. Y., and Cui, S. J. (2012). A Na+/Ca2+ exchanger-like protein (AtNCL) involved in salt stress in Arabidopsis. J. Biol. Chem. 287, 44062–44070. doi: 10.1074/jbc.M112.351643
Weiland, M., Mancuso, S., and Baluska, F. (2016). Signalling via glutamate and GLRs in Arabidopsis thaliana. Funct. Plant Biol. 43, 1–25.
Whalley, H. J., and Knight, M. R. (2013). Calcium signatures are decoded by plants to give specific gene responses. New Phytol. 197, 690–693. doi: 10.1111/nph.12087
Whalley, H. J., Sargeant, A. W., Steele, J. F., Lacoere, T., Lamb, R., Saunders, N. J., et al. (2011). Transcriptomic analysis reveals calcium regulation of specific promoter motifs in Arabidopsis. Plant Cell 23, 4079–4095. doi: 10.1105/tpc.111.090480
White, P. J. (2009). Depolarization-activated calcium channels shape the calcium signatures induced by low-temperature stress. New Phytol. 183, 7–8. doi: 10.1111/j.1469-8137.2009.02857.x
Wigge, P. A. (2013). Ambient temperature signalling in plants. Curr. Opn. Plant Biol. 16, 661–666. doi: 10.1016/j.pbi.2013.08.004
Wu, J. Y., Jin, C., Qu, H. Y., Tao, S. T., Xu, G. H., Wu, J., et al. (2012). Low temperature inhibits pollen viability by alteration of actin cytoskeleton and regulation of pollen plasma membrane ion channels in Pyrus pyrifolia. Environ. Expt. Bot. 78, 70–75. doi: 10.1016/j.envexpbot.2011.12.021
Xu, Q. T., Fan, H. Y., Jiang, Z., Zhou, Z. Q., Yang, L., Mei, F. Z., et al. (2013). Cell wall degradation and the dynamic changes of Ca2+ and related enzymes in the developing aerenchyma of wheat (Triticum aestivum L.) under waterlogging. Acta Biol. Hung. 64, 328–340. doi: 10.1556/ABiol.64.2013.3.6
Yamada, N., Theerawitaya, C., Ca-Um, S., Kirdmanee, C., and Takabe, T. (2014). Expression and functional analysis of putative vacuolar Ca2+-transporters (CAXs and ACAs) in roots of salt tolerant and sensitive rice cultivars. Protoplasma 251, 1067–1075. doi: 10.1007/s00709-014-0615-2
Yamauchi, T., Watanabe, K., Fukazawa, A., Mori, H., Abe, F., Kawaguchi, K., et al. (2014). Ethylene and reactive oxygen species are involved in root aerenchyma formation and adaptation of wheat seedlings to oxygen-deficient conditions. J. Expt. Bot. 65, 261–273. doi: 10.1093/jxb/ert371
Yang, C.-Y., and Hong, C.-P. (2015). The NADPH oxidase RbohD is involved in primary hypoxia signalling and modulates expression of hypoxia-inducible genes under hypoxic stress. Env. Expt. Bot. 115, 63–72. doi: 10.1016/j.envexpbot.2015.02.008
Yang, T. B., Chaudhuri, S., Yang, L. H., Du, L. Q., and Poovaiah, B. W. (2010). A calcium/calmodulin-regulated member of the receptor-like kinase family confers cold tolerance in plants. J. Biol. Chem. 285, 7119–7126. doi: 10.1074/jbc.M109.035659
Yeh, C. M., Chien, P. S., and Huang, H. J. (2007). Distinct signalling pathways for induction of MAP kinase activities by cadmium and copper in rice roots. J. Expt. Bot. 58, 659–671. doi: 10.1093/jxb/erl240
Yemelyanov, V. V., Shishova, M. F., Chirkova, T. V., and Lindberg, S. M. (2011). Anoxia-induced elevation of cytosolic Ca2+ concentration depends on different Ca2+ sources in rice and wheat protoplasts. Planta 234, 271–280. doi: 10.1007/s00425-011-1396-x
Yu, H. Q., Yong, T. M., Li, H. J., Liu, Y. P., Zhou, S. F., Fu, F. L., et al. (2015). Overexpression of a phospholipase D alpha gene from Ammopiptanthus nanus enhances salt tolerance of phospholipase D alpha 1-deficient Arabidopsis mutant. Planta 242, 1495–1509. doi: 10.1007/s00425-015-2390-5
Yu, L. J., Nie, J. N., Cao, C. Y., Jin, Y. K., Yan, M., Wang, F. Z., et al. (2010). Phosphatidic acid mediates salt stress response by regulation of MPK6 in Arabidopsis thaliana. New Phytol. 188, 762–773. doi: 10.1111/j.1469-8137.2010.03422.x
Yu, Y. Q., and Assmann, S. M. (2015). The heterotrimeric G-protein beta subunit, AGB1, plays multiple roles in the Arabidopsis salinity response. Plant Cell Environ. 38, 2143–2156. doi: 10.1111/pce.12542
Yuan, F., Yang, H., Xue, Y., Kong, D., Ye, R., Li, C., et al. (2014). OSCA1 mediates osmotic-stress-evoked Ca2+ increases vital for osmosensing in Arabidopsis. Nature 514, 367–371. doi: 10.1038/nature13593
Yuan, H.-M., and Huang, X. (2016). Inhibition of root meristem growth by cadmium involves nitric oxide-mediated repression of auxin accumulation and signalling in Arabidopsis. Plant Cell Environ. 39, 120–135. doi: 10.1111/pce.12597
Yuen, C. C. Y., and Christopher, D. A. (2013). The group IV-A cyclic nucleotide-gated channels, CNGC19 and CNGC 20, localize to the vacuole membrane in Arabidopsis thaliana. AoB Plants 5, plt012. doi: 10.1186/1471-2164-15-853
Zelinova, V., Alemayehu, A., Bocova, B., Huttova, J., and Tamas, L. (2015). Cadmium-induced reactive oxygen species generation, changes in morphogenic responses and activity of some enzymes in barley root tip are regulated by auxin. Biologia 70, 356–364. doi: 10.1515/biolog-2015-0035
Zeng, F., Konnerup, D., Shabala, L., Zhou, M., Colmer, T. D., Zhang, G., et al. (2014). Linking oxygen availability with membrane potential maintenance and K+ retention of barley roots: implications for waterlogging stress tolerance. Plant Cell Environ. 37, 2325–2338. doi: 10.1111/pce.12422
Zeng, Q., Ling, Q., Fan, L., Li, Y., Hu, F., Chen, J., et al. (2015). Transcriptome profiling of sugarcane roots in response to potassium stress. PLoS ONE 10:e0126306. doi: 10.1371/journal.pone.0126306
Zepeda-Jazo, I., Maria Velarde-Buendia, A., Enriquez-Figueroa, R., Bose, J., Shabala, S., Muniz-Murguia, J., et al. (2011). Polyamines interact with hydroxyl radicals in activating Ca2+ and K+ transport across the root epidermal plasma membranes. Plant Physiol. 157, 2167–2180. doi: 10.1016/j.plaphy.2012.09.002
Zhang, L., Chen, Z., and Zhu, C. (2012). Endogenous nitric oxide mediates alleviation of cadmium toxicity induced by calcium in rice seedlings. J. Environ. Sci. 24, 940–948. doi: 10.1016/S1001-0742(11)60978-9
Zhang, X., Shen, Z. D., Sun, J., Yu, Y. C., Deng, S. R., and Li, Z. Y. (2015). NaCl-elicited, vacuolar Ca2+ release facilitates prolonged cytosolic Ca2+ signaling in the salt response of Populus euphratica cells. Cell Calcium 57, 348–365. doi: 10.1016/j.ceca.2015.03.001
Zhang, Y., Wang, Y., Taylor, J. L., Jiang, Z., Zhang, S., Mei, F., et al. (2015). Aequorin-based luminescence imaging reveals differential calcium signalling responses to salt and reactive oxygen species in rice roots. J. Expt. Bot. 66, 2535–2545. doi: 10.1093/jxb/erv043
Zhao, M. G., Tian, Q. Y., and Zhang, W. H. (2007). Ethylene activates a plasma membrane Ca2+-permeable channel in tobacco suspension cells. New Phytol. 174, 507–515. doi: 10.1111/j.1469-8137.2007.02037.x
Zhao, R., Sun, H. M., Zhao, N., Jing, X. S., Shen, X., and Chen, S. (2015). The Arabidopsis Ca2+-dependent protein kinase CPK27 is required for plant response to salt-stress. Gene 563, 203–214. doi: 10.1016/j.gene.2015.03.024
Zhao, Y., Pan, Z., Zhang, Y., Qu, X. L., Zhang, Y. G., and Yang, Y. G. (2013). The Actin-Related Protein2/3 complex regulates mitochondrial-associated calcium signaling during salt stress in Arabidopsis. Plant Cell 25, 4544–4559. doi: 10.1105/tpc.113.117887
Zhu, S.-Y., Yu, X.-C., Wang, X.-J., Zhao, R., Li, Y., Fan, R.-C., et al. (2007). Two calcium-dependent protein kinases, CPK4 and CPK11, regulate abscisic acid signal transduction in Arabidopsis. Plant Cell 19, 3019–3036. doi: 10.1105/tpc.107.050666
Keywords: abiotic stress, calcium, heavy metal, hypoxia, nutrition, salinity, signaling
Citation: Wilkins KA, Matthus E, Swarbreck SM and Davies JM (2016) Calcium-Mediated Abiotic Stress Signaling in Roots. Front. Plant Sci. 7:1296. doi: 10.3389/fpls.2016.01296
Received: 24 May 2016; Accepted: 12 August 2016;
Published: 29 August 2016.
Edited by:
Janin Riedelsberger, University of Talca, ChileReviewed by:
Girdhar Kumar Pandey, University of Delhi, IndiaCopyright © 2016 Wilkins, Matthus, Swarbreck and Davies. This is an open-access article distributed under the terms of the Creative Commons Attribution License (CC BY). The use, distribution or reproduction in other forums is permitted, provided the original author(s) or licensor are credited and that the original publication in this journal is cited, in accordance with accepted academic practice. No use, distribution or reproduction is permitted which does not comply with these terms.
*Correspondence: Julia M. Davies, am1kMzJAY2FtLmFjLnVr
Disclaimer: All claims expressed in this article are solely those of the authors and do not necessarily represent those of their affiliated organizations, or those of the publisher, the editors and the reviewers. Any product that may be evaluated in this article or claim that may be made by its manufacturer is not guaranteed or endorsed by the publisher.
Research integrity at Frontiers
Learn more about the work of our research integrity team to safeguard the quality of each article we publish.