- 1State Key Laboratory of Soil Erosion and Dryland Farming on the Loess Plateau, Northwest A&F University, Yangling, China
- 2Fujian Key Laboratory of Subtropical Plant Physiology and Biochemistry, Fujian Institute of Subtropical Botany, Xiamen, China
- 3College of Life Science, Northwest A&F University, Yangling, China
- 4Key Laboratory of the Ministry of Education for Coastal and Wetland Ecosystem, College of the Environment and Ecology, Xiamen University, Xiamen, China
Hydrogen sulfide (H2S) is a newly appreciated participant in physiological and biochemical regulation in plants. However, whether H2S is involved in the regulation of plant responses to drought stress remains unclear. Here, the role of H2S in the regulation of drought stress response in Spinacia oleracea seedlings is reported. First, drought stress dramatically decreased the relative water content (RWC) of leaves, photosynthesis, and the efficiency of PSII. Moreover, drought caused the accumulation of ROS and increased the MDA content. However, the application of NaHS counteracted the drought-induced changes in these parameters. Second, NaHS application increased the water and osmotic potential of leaves. Additionally, osmoprotectants such as proline and glycinebetaine (GB) content were altered by NaHS application under drought conditions, suggesting that osmoprotectant contributes to H2S-induced drought resistance. Third, the levels of soluble sugars and polyamines (PAs) were increased differentially by NaHS application in S. oleracea seedlings. Moreover, several genes related to PA and soluble sugar biosynthesis, as well as betaine aldehyde dehydrogenase (SoBADH), choline monooxygenase (SoCMO), and aquaporin (SoPIP1;2), were up-regulated by H2S under drought stress. These results suggest that H2S contributes to drought tolerance in S. oleracea through its effect on the biosynthesis of PAs and soluble sugars. Additionally, GB and trehalose also play key roles in enhancing S. oleracea drought resistance.
Introduction
Drought induces water scarcity and osmotic stresses that restrict the growth and development of plants because water absorption by plant roots from the soil is inadequate to satisfy plant transpiration demand (Blum, 1996). Additionally, leaf cell turgor is significantly lessened by water deficiency, which in turn restricts cell expansion, the increase of leaf area, and photosynthesis, thus inhibiting the accumulation of biomass (Chaves et al., 2003). An effective strategy for plant drought adaptability has been to inhibit water scarcity caused by transpiration, which saves soil moisture and allows plants to sustain sufficient water content to maintain key physiological processes (Yoo et al., 2010).
During water deficiency and other osmotic stresses, the accumulation of compatible low-molecular-weight osmoprotectants, such as soluble sugars (including glucose, fructose, and sucrose), proline, sugar alcohols, special amino acids and glycinebetaine (GB) may confer stress tolerance to plants (García-Mata and Lamattina, 2001; Rivero et al., 2014). Additionally, the composition and content of the osmoprotectants in stressed plants can vary considerably depending on the species and environmental conditions (Rivero et al., 2014). Moreover, polyamines (PAs), including putrescine (Put), spermidine (Spd) and spermine (Spm), are ubiquitous biological organic-amines involved in plant growth, development and the response to biotic and abiotic stresses (Takahashi and Kakehi, 2010). When plants are exposed to stress conditions such as drought, heat, chilling, or salinity, PA levels significantly increase and become one of the most prominent metabolic features (Takahashi and Kakehi, 2010; Tiburcio et al., 2014). These changes are caused mainly by the biosynthesis of PAs, PA oxidation, and/or interactions with other pathways under stress conditions (Tiburcio et al., 2014). The PA metabolic pathway mediates nitrogen or carbon metabolism and interacts with other metabolites, such as stress-protective compounds including signaling molecules and hormones (Moschou et al., 2012; Tiburcio et al., 2014). As an example, the abscisic acid (ABA)-induced accumulation of PAs enhances PA oxidation, which in turn enacts protection against such stresses as against drought in plants (Toumi et al., 2010). Additionally, many previous studies have shown that PAs play an important role in salt tolerance and drought resistance in plants (Alcázar et al., 2010b; Yin et al., 2013, 2015). Under drought stress, plants with high PA contents exhibit strong adaptability, and exogenous PAs alleviate drought stress. All these results demonstrate that PA levels are positively correlated to drought resistance in plants (Capell et al., 2004). Recently, much research has indicated that the overexpression of PA biosynthesis-related genes increases PA content and improves drought tolerance or salt stress (Capell et al., 2004; Wen et al., 2008; Alcázar et al., 2010a). Moreover, knockout/knock-down mutants whose capacity to synthesize PAs were abolished or limited were more sensitive to drought or salt stress than wild-type plants (Kasinathan and Wingler, 2004). Together, these studies indicate that PAs play a vital role in regulating drought tolerance and salt resistance in plants.
The small bioactive gaseous hydrogen sulfide (H2S) exhibits both physical and functional similarities with other gasotransmitters such as carbon monoxide (CO) and nitric oxide (NO) and has been shown to be involved in different physiological processes in animals (Hosoki et al., 1997; Zhao et al., 2001; Wang, 2002; Li et al., 2006; Yang et al., 2008). NaHS, a donor of H2S, has been used to study the physiological function of H2S in animals and plants (Hosoki et al., 1997; Zhang et al., 2008; García-Mata and Lamattina, 2010). Recently, much of this research has focused on the physiological function of H2S in plants. Specifically, H2S promotes seed germination, protects against copper-induced oxidative damage, counteracts chlorophyll loss, and alleviates oxidative damage from water scarcity in sweet potato leaves (Zhang et al., 2008, 2009). Additionally, boron, salinity and aluminum toxicities were alleviated by H2S treatment (Wang et al., 2010, 2012; Zhang et al., 2010b; Chen et al., 2013, 2015a). Furthermore, low concentrations of H2S regulated flower senescence and promoted the embryonic root length of Pisum sativum (Li et al., 2010; Zhang et al., 2011). In addition, García-Mata and Lamattina (2010) reported that H2S regulated the ATP-binding cassette (ABC) transporter in guard cells and then caused stomatal closure; these processes are closely connected to the ABA signaling pathway. Our previous study also showed that H2S promoted iron availability in Zea mays seedlings and enhanced photosynthesis in S. oleracea seedlings (Chen et al., 2011, 2015b). Moreover, many previous studies have shown that H2S can effectively improve drought tolerance in many different plants (Zhang et al., 2010a; Shan et al., 2011; Jin et al., 2013). For example, Ziogas et al. (2015) reported that H2S pre-treatment led to the post-translational modification (PTM) of proteins under drought stress in citrus plants. Additionally, Li et al. (2015) showed that H2S alleviated drought-induced PSII damage due to fast D1 protein turnover. A previous study also showed that H2S regulated the expression of drought-related genes including DREB and RD29A (Jin et al., 2011). The aforementioned studies focused on only the protection against drought-induced oxidative damage H2S offered via the enhancement of non-enzymatic and enzymatic antioxidant capacities, the reduction of evaporation, or the control of stomatal conductance in plants. However, the detailed mechanisms of the effects of H2S on drought stress remain unclear, especially with respect to physiological processes in plants.
Because PAs and soluble sugars particularly enhance drought tolerance, the question of whether PAs and soluble sugars are involved in H2S-induced drought tolerance needs to be addressed. Recent studies have shown that the bioactive free radical molecule NO and PAs share certain overlapping physiological roles in plants (Yamasaki and Cohen, 2006). The synthesis of NO was induced by PAs in Arabidopsis, which indicates that NO might contribute to PA signaling during stress (Tun et al., 2006). However, whether a low concentration of H2S similar to that of NO has an effect on PA levels and further enhances the resistance of plants to stress remains unclear.
In this study, we present important data that reveals a novel effect of H2S in plant physiology, more specifically on drought stress. These results support the suggestion that H2S significantly enhances the tolerance of plants to drought stress through its effects on PA and soluble sugar contents, which change the expression levels of genes associated with PAs and sugar biosynthesis.
Materials and Methods
Plant Culture and Treatment
Seeds of Spinacia oleracea were first sterilized by immersion in 75% ethanol for 3 min followed by 10 min in a 10% sodium hypochlorite solution. Next, seeds were washed with distilled water and germinated in a soil/vermiculite (1:1) mixture. Subsequently, 1-week-old seedlings were transferred to plastic pots (15 cm × 15 cm; 25 seedlings per pot) filled with a soil/vermiculite (1:1) mixture The plants were then grown in a controlled growth chamber with a light/dark regime of 10/14 h, a relative humidity of 80%, a temperature of 21/27°C, and a photosynthetically active radiation (PAR) of 190 μmol m-2 s-1 and were watered with a ½ strength Hoagland solution every other days.
NaHS was used as the exogenous H2S donor as described by Hosoki et al. (1997). Six-week-old seedlings were treated with the ½ strength Hoagland solution containing 100 μM NaHS. The same volume ½ strength Hoagland solution was used as the control. For drought treatment, 6-week-old plants subjected to drought stress, were grown in pots by withholding water for 8 days, and then re-watered until the substrate was saturated. Treated leaves were sampled after 0, 5, and 8 days of drought stress and after 1 and 4 days of re-watering. All samples were frozen rapidly in liquid nitrogen and stored at -80°C.
Measurement of Soil Water Content, Plant Survival, Leaf Relative Water Content, and Detached Leaf Water Loss
Drought stress was imposed by withholding water from containers of soilless media (with dry weight of 58.6 ± 5.4 g) containing 25 plants (6-week-old). The containers were irrigated with water to saturation and weighed at the start of the drought stress treatment (initial weight) and then periodically throughout the treatment period. Relative soil water content (SWC) was calculated as follows: (final fresh weight-dry weight)/(initial weight-dry weight) × 100. After 8 days of drought, the plants were re-watered, and the plant survival was measured 4 days after re-watering (Yoo et al., 2010).
In a separate experiment, the relative water content (RWC) of fully expanded leaves was assessed in 6-week-old plants growing at different relative SWC. Leaves were cut and immediately weighed to obtain the leaf FW. The leaves were then placed into small bottles filled with distilled water for 24 h, blotted up to remove excess water, and then weighed to obtain the leaf turgid weight (TW). The leaves were then dried to a constant weight at 70°C and reweighed to obtain the leaf dry weight (DW). The leaf RWC was calculated as follows: (FW-DW)/(TW-DW) × 100 (Yoo et al., 2010).
The detached leaves were treated with different concentrations of NaHS (0, 10, 100, 500, and 1000 μM) for 3 h and the leaf RWC was measured according to the method of Yoo et al. (2010). Water loss in the detached leaf was determined according to the method of Whittaker (1994). Leaves were excised from plants, weighed to determine their initial weight, placed with their adaxial surfaces upward on a petri dish in a light incubator (at a temperature of 25 ± 2°C and a relative humidity of 60%) and reweighed at 30 min intervals for up to 6 h to obtain the actual time fresh weight. The water loss was calculated as follows: (initial weight-actual time fresh weight)/initial weight × 100.
Measurement of Endogenous H2S Concentration
Endogenous H2S was measured by the formation of methylene blue from dimethyl-p-phenylenediamine in H2SO4 according to the method described by Sekiya et al. (1982) and Zhang et al. (2009) with some little modifications. Leaves (0.3 g) were ground and then extracted with 3 ml of phosphate buffer solution (pH 6.8, 50 mM) including 0.2 M ascorbic acid and 0.1 M EDTA. Subsequently, 1 M HCl (0.5 ml) was added to the homogenate in a test tube for releasing H2S, and 0.5 ml of 1% (w/v) zinc acetate was used to absorb H2S. After 30 min of reaction, 5 mM dimethyl-p-phenylenediamine (0.3 ml) dissolved in 3.5 mM H2SO4 was added to the trap. Then, 50 mM ferric ammonium sulfate in 100 mM H2SO4 was injected into the trap. The amount of H2S in the zinc acetate traps was determined at OD 667 nm after incubating the mixture for 15 min at room temperature. Blanks were prepared using the same procedures without the zinc acetate solution.
Gas Exchange and Chlorophyll Fluorescence Quenching Analyses
The gas exchange of fully expanded leaves (the number leaves of a plant was about 12–14), including transpiration, stomatal conductance, and net CO2 assimilation, was determined using a portable photosynthesis system (Li-6400, Li-Cor, Lincoln, NE, USA). Plants were grown for 6 weeks under short-day conditions (10 h light). Such conditions resulted in plants with leaves large enough to fill the 6-cm2 Li-6400 chamber. Gas exchange was measured at PAR levels of 1200, 800, 600, 400, 200, 100, 50, 20, 10, and 0 μmol m-2 s-1. All measurements were conducted in the morning (9:00–11:30) to avoid the high temperature and air vapor pressure deficits in the afternoon. Light was supplemented using an LED light system. Using non-rectangular hyperbola modeling, the response of leaf Pn to PAR was calculated with respect to the apparent dark respiration (Rd), light compensation point (Lcp), light saturation point (Lsp), apparent quantum yield (AQE) and maximal net photosynthetic rate (Pmax), as described by Prioul and Chartier (1977).
where 𝜃 is the convexity, quantum efficiency is the initial slope of the curve, and Rd is the point at which the curve crosses the y axis at PAR = 0, and the Lcp at which the curve crosses the x axis. The vapor pressure deficit (VPD) during measurement was ∼1 kPa. The instantaneous water use efficiency (WUE) was calculated as the net CO2 assimilation rate divided by the transpiration rate.
Chlorophyll fluorescence is considered a tool for interpreting the stress tolerance of plants by evaluating the physiological status of the plant and the state of Photosystem II (PSII) (Krause and Weis, 1991; Peeva and Cornic, 2009). The chlorophyll fluorescence of S. oleracea was measured using a Plant Efficiency Analyzer (Hansatech Instruments Ltd., Norfolk, England). The ratio of variable (Fv) to maximum fluorescence (Fm) was measured in four seedlings per pot. The leaves were dark-adapted for more than 30 min prior to the measurement. The minimum fluorescence (Fo), the Fm, the variable fluorescence (Fv = Fm-Fo) and the ratio of Fv/Fm were recorded for 15 s at a 100% intensity level of the photon flux density (4000 μmol m-2 s-1). Additionally, the steady-state fluorescence level reached upon continuous illumination (Fs′), the maximal fluorescence level induced by a saturating light pulse at the steady-state (Fm′), and the minimum fluorescence level after a 3 s period of far-red light required to oxidize the plastoquinone pool (Fo′) were measured and used to calculate other parameters as follows: The quantum yield of PSII photochemistry (PSII) was calculated as 1-(Fs′/Fm′). The electronic transport ratio (ETR) was calculated as PAR×PSII×0.85×0.5. Non-photochemical quenching (NPQ) was calculated as (Fm/Fm′)-1. Photochemical quenching (qP) was calculated as (Fm′-Fs)/(Fm′-Fo′) (Krause and Weis, 1991).
Measurement of Leaf Water Potential and Osmotic Potential
Leaf water potential was measured after plants were subjected to drought for 0, 5 and 8 days and re-watered for 1 and 4 days. The fully expanded leaves were selected and measured between 10:00 and 11:00 A.M. using a pressure chamber (Model 1000, PMS Instrument Co., Corvallis, OR, USA). The osmotic potential was measured using a dew point microvolt meter (Model 5520, Wescor, Logan, UT, USA). Leaf water and osmotic potentials were calculated according to the method of Yin et al. (2013). Each treatment included six replicates.
Measurement of Superoxide Radical, Hydrogen Peroxide and Lipid Peroxidation
The production of superoxide radical () was measured using the method of Wang and Luo (1990) with some modifications. Hydrogen peroxide (H2O2) was measured spectrophotometrically according to Alexieva et al. (2001). The lipid peroxidation level was determined in terms of malondialdehyde (MDA) content via the thiobarbituric acid (TBA) reaction as described by Dhindsa et al. (1981).
Measurement of Osmolyte Content
Proline content was determined according to the method of Bates et al. (1973). Glycinebetaine content was measured using an HPLC Shimadzu-V analytical procedure according to the method of Bessieres et al. (1999) with some modifications. Frozen leaf samples in liquid nitrogen were homogenized with 4 ml water: chloroform: methanol (3: 5: 12 v/v/v) solution and incubated overnight at 4°C. The upper methanolic phase (1 ml) of the extract was purified using BioRad AG1-X8 ion exchange resin. The ion exchange resin was removed by centrifugation at 5000 g for 10 min, and the supernatant was filtered using a 0.22 μm membrane filter before being loaded into the HPLC system. A Nucleogel RP column (RP-S 100-8, 300 × 7.7 mm) proceeded by a guard column was used, and the mobile phase (15 mM KH2PO4) was delivered by an analytical isocratic pump at a flow rate of 0.7 ml min-1 at 70°C. The GB content was measured using a UV detector at 192 nm, and quantification was performed by comparing the peak surface areas.
Measurement of Glucose, Fructose, Sucrose, and Trehalose Content
Glucose and sucrose were measured according to the method of Zhao et al. (2004) using the Shimadzu sugar analysis system (HPLC, Shimadzu, Kyoto, Japan). Leaves were homogenized in 7 ml 85% (v/v) ethanol, the first 5 ml aliquot was adjusted to 1 mM with respect to lactose and used as an internal standard. The slurry was transferred to a 10 ml tube, and the mortar was washed with an additional 2 ml 80% ethanol, which was added to the tube. The suspensions were heated at 80°C for 30 min and centrifuged at 12000 g for 20 min, and then the supernatant was collected. The supernatant was heated at 95 °C for 2 h to remove ethanol, and the final sample (∼500 μl) was frozen at -80°C for 24 h. Subsequently, the sample was lyophilized to dryness, reconstituted in 500 μl distilled, deionized water, and centrifuged at 12000 g for 20 min. Then, the supernatant was passed through a membrane filter (pore size 0.22 μm) for analysis. Sugars in the extracts were injected into an eluent of 19 mM NaOH delivered at 1 ml min-1 using a Waters 1525 HPLC pump (Waters 1525/VIS 2424 Detector, BreezeTM, USA) and separated by anion exchange using an XBridgeTM amide C18 column (4.6 × 250 mm, 3.5 μm particle size) with guard column. Each treatment included four replicates.
Fructose and trehalose contents were estimated using fructose and trehalose reagent kits, respectively (Shanghai Solarbio Biocompany, Shanghai, China). The absorbances of fructose and trehalose were measured at 530 and 520 nm, respectively, following the manufacturer’s instructions.
Measurement of Polyamines Content
Polyamines (PAs) were extracted according to the method of Flores and Galston (1982) and Duan et al. (2008). Briefly, leaves were ground in 1 ml of 5% (v/v) cold perchloric acid and then incubated at 4°C for 1 h. After incubation, the homogenate was centrifuged at 12000 g for 30 min at 4°C. To measure the conjugated PAs, the plant extract residues were washed with 5% HClO4 and then subjected to hydrolysis in 6 M HCl at 110°C for 16 h. The filtered hydrolysates were allowed to evaporate to dryness, and the residues were dissolved in 5% HClO4 for the measurement of conjugated PAs. Subsequently, the supernatant and the suspension residues were benzoylated as follows: A 500 μl volume of supernatant was treated with 2 ml of 2 N NaOH and 10 μl benzoyl chloride, and the mixture was then vortexed for 30 s and incubated for 30 min at 37°C. The reaction was terminated by adding 2 ml saturated NaCl solution. Next, the benzoyl polyamine was extracted with 2 ml cold diethyl. Ultimately, 1 ml ether phase was evaporated to dryness and dissolved in 1 ml of methanol for the determination of endogenous polyamines. Eventually, high performance liquid chromatography (Waters 1525/UVD 2489 Detector, BreezeTM, USA) was used to analyze the endogenous PA content. Briefly, 20 μl of benzoyl PAs were injected into the loop and then loaded onto a Waters Symmetry C18 column (7.5 cm × 4.6 mm, 3.5 μm particle size). The column temperature was maintained at 30°C for PAs. The mobile phase was methanol-H2O (59:41, v:v). The PA peaks were detected with a UV detector at 230 nm at a flow rate of 0.7 ml min-1.
Total RNA Extraction and Gene Expression Analysis
Frozen leaves (0.5 g) were ground in liquid nitrogen with 2% polyvinylpyrrolidone and extracted with an RNA purification reagent (Invitrogen Inc., Carlsbad, CA, USA) according to the manufacturer’s instructions. The RNA content was determined with an ultraviolet spectrophotometer (Cary 50, Varian, Agilent), and the integrity of the RNA was evaluated by 1% agarose gel electrophoresis. Total RNA was reverse-transcribed into first-strand cDNAs using M-MLV reverse transcriptase (TaKaRa, Dalian, China). A 10-μl real-time PCR reaction contained the following: 0.6 μl of forward and reverse primers for the sugar biosynthesis-related genes, PA biosynthesis-related genes, betaine aldehyde dehydrogenase (SoBADH), choline monooxygenase (SoCMO), and aquaporin (SoPIP1;2) (listed in Supplementary Table S1); 1 μl of cDNA (equivalent to 10 ng of mRNA) and 5 μl of FastStart Universal SYBR Green Master Mix (ROX, Mannheim, Germany). The amplification and detection of the dsDNA synthesis of these genes were performed using the PCR conditions described in Supplementary Table S1. Three independent replicates were performed for each sample. The comparative threshold cycle (Ct) method was used to determine the relative amount of gene expression. The glyceraldehyde-3-phosphate dehydrogenase gene (SoGADPH) was used as an internal control. The mRNA transcriptional abundance value of the genes was expressed as 2-ΔΔCt (Livak and Schmittgen, 2001). A LightCycler 480II Real-Time PCR System (Roche, Bern, Switzerland) was used to perform qRT-PCR.
Statistical Analysis
At least six leaves were used to measure gas exchange and chlorophyll fluorescence quenching. At least three replicates were conducted for physiological and biochemical analyses. One-way or two-way ANOVA procedure with the SPSS 19.0 software (SPSS Inc., Chicago, IL, USA) were used for analyzing statistical significances, and the results are expressed as the mean values ± SE. Post hoc comparisons were tested using the Tukey test at a significance level of P< 0.05.
Results
Effect of H2S Donor on Plant Water Stress Responses
Under drought conditions, and thus under low relative SWC, S. oleracea seedlings treated with 100 μM NaHS, a donor of H2S, survived better than untreated control plants (Figure 1A). Most of the control plants wilted at 10 ± 1.5% SWC, while most of the NaHS-treated plants presented less severe leaf wilting symptoms (Figure 1A). Additionally, on the first day after re-watering, the NaHS-treated seedlings recover more quickly than the control plants (Figure 1A). Moreover, more than 80% of the NaHS-treated plants and <10% of the control plants survived to the fourth day after re-watering (Figure 1B). Increased drought survival of the NaHS-treated plants was associated with a higher capacity to maintain RWC than the control plants at 29 ± 2.5% SWC (Figure 1C). To understand the physiological mechanism through which the NaHS-treated plants acquired water stress tolerance by maintaining higher leaf water content under low soil moisture conditions, we analyzed the effect of different concentrations of NaHS on the RWC of detached leaves of S. oleracea seedlings. The RWC of detached S. oleracea seedling leaves treated with various concentrations of NaHS (10–1000 μM) were significantly higher than that of control plants, with an optimal NaHS concentration of 100 μM (Supplementary Figure S1A). Indeed, the detached leaves treated with 100 μM NaHS exhibited the highest leaf RWC and the lowest leaf water loss ratio of all plants (Supplementary Figures S1B,C). The leaf water loss of NaHS-treated plants decreased by ∼10% compared with that of the control plants (Supplementary Figure S1D).
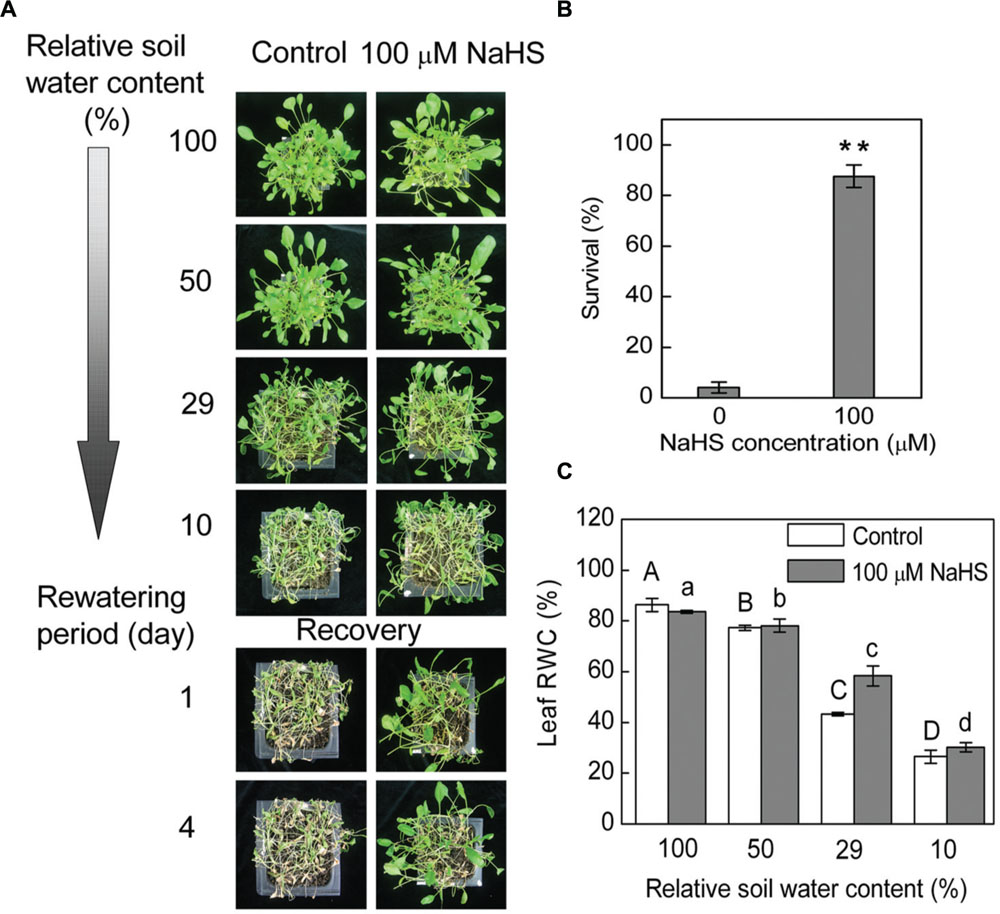
FIGURE 1. Plant water stress response was analyzed in 6-week-old NaHS-treated Spinacia oleracea seedlings grown under drought and rewatering conditions. Four containers of each treatments were determined in three independent experiments. Relative soil water content is the soil water relative to the soil water at day 0 of withholding water and is the average of four containers. The photograph in (A) illustrates a representative result of one replicate from one experiment. Four days after rewatering, plant survival was determined (mean ± SE, n = 4) (B). In a separate experiment, 6-week-old seedlings were exposed to water deficit stress by withholding water. Leaf RWC (mean ± SE, n = 6) was determined (C). In (B), the significant level of the difference between control and treatment is indicated by two asterisks **P< 0.01. In (C), the columns labeled with different letters indicate significant differences at P < 0.05.
Effect of H2S Donor on the Endogenous H2S Concentration
To determine whether exogenous H2S affects endogenous H2S concentrations in S. oleracea seedlings suffering from drought stress, we measured the endogenous H2S concentration of S. oleracea seedling leaves. As shown in Figure 2, 8 days of drought stress promoted endogenous H2S production in S. oleracea seedlings. Moreover, exogenously applied NaHS also increased the production of endogenous H2S under drought stress. However, re-watering for 4 days inhibited the drought-induced endogenous production of H2S in S. oleracea seedling leaves (Figure 2).
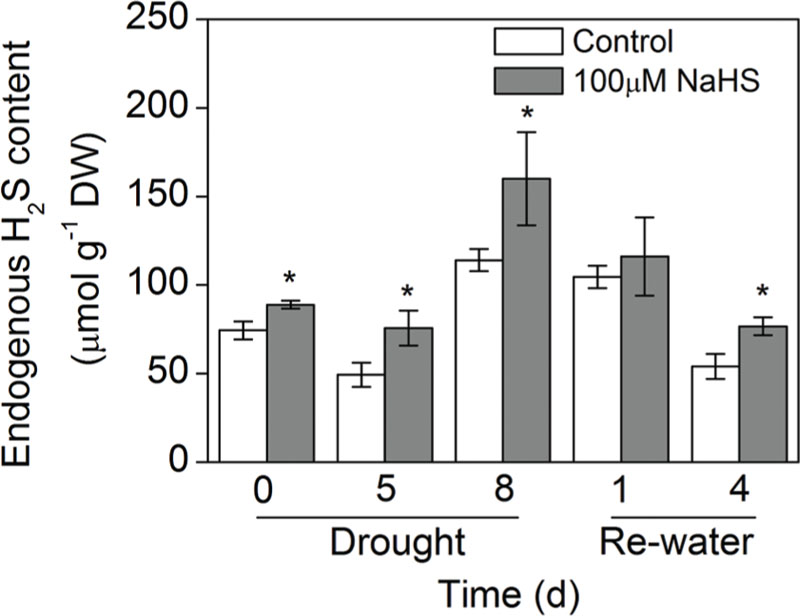
FIGURE 2. Effect of H2S donor NaHS on the endogenous H2S concentration in 6-week-old S. oleracea seedlings leaves after drought for 0, 5, and 8 days and rewatering for 1 and 4 days. The significant level of the difference between control and treatment is indicated by an asterisk ∗P< 0.05.
Effect of the H2S Donor on Leaf Photosynthesis and Electron Transport Efficiency of PSII
There is a close relationship between water stress and photosynthesis in plants. Accordingly, we measured the photosynthesis of S. oleracea seedlings treated with NaHS under drought condition. The net CO2 assimilation rate of the NaHS-treated plants was significantly increased compared with that of control plants (Figure 3A). Additionally, the stomatal conductance of the NaHS-treated plants was somewhat higher than that of the control plants, although the difference was not statistically significant (Figure 3B). The internal CO2 concentration in the leaves of NaHS-treated plants was higher than in those of the control plants (Figure 3C). Additionally, at saturating light levels, the leaf transpiration rate of the NaHS-treated plants was higher than that of the control plants (Figure 3D). However, the instantaneous WUE values of the NaHS-treated plants and the control plants were similar (Figure 3E). Additionally, the VPD of the NaHS-treated plants was higher than that of the control plants (Figure 3F). Moreover, the Rd, Lcp, Lsp, AQE, and Pmax of S. oleracea plants were calculated by modeling the response of the leaf Pn to PAR via a non-rectangular hyperbola (Table 1). Lcp, Lsp, and Pmax were notably higher in the NaHS-treated plants than in the controls. These results demonstrate that NaHS treatment increased the photosynthesis of S. oleracea seedlings under drought condition.
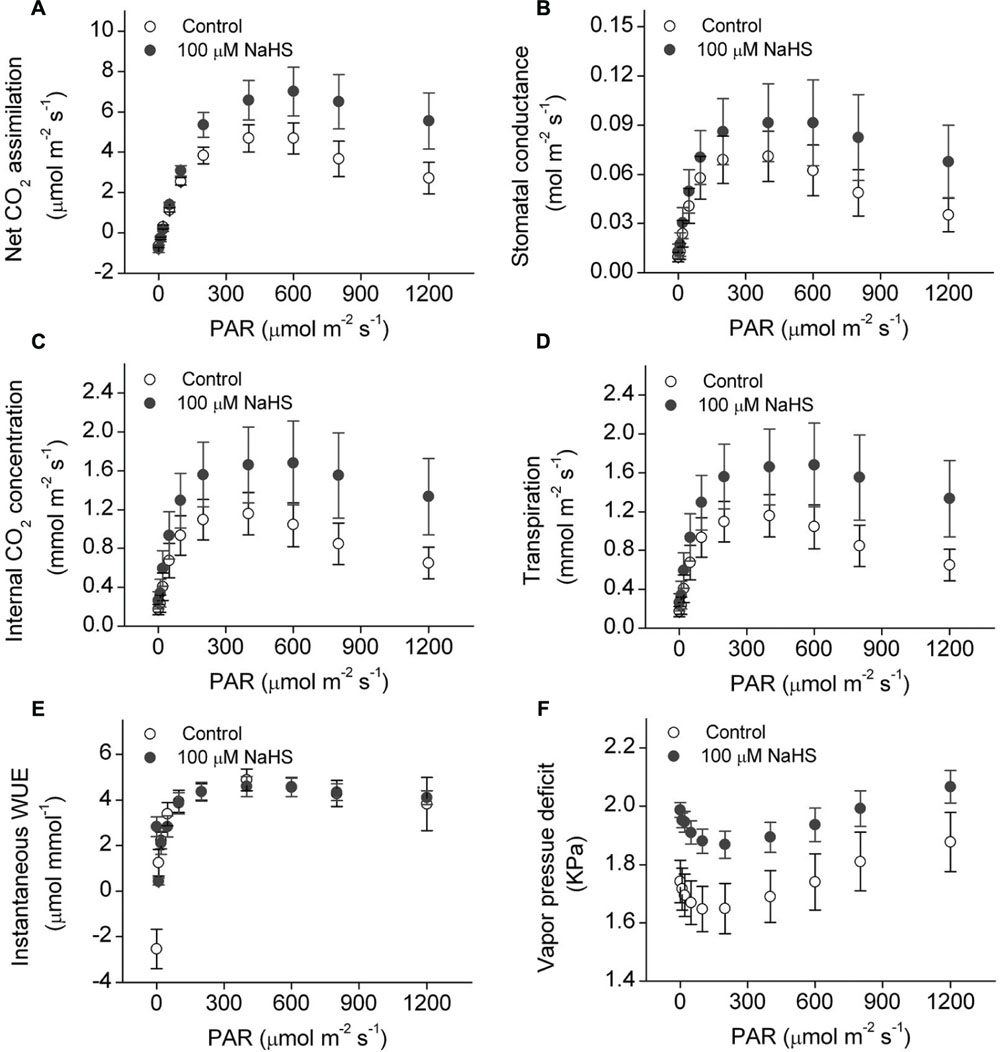
FIGURE 3. Effect of H2S donor NaHS on net CO2 assimilation (A), stomatal conductance (B), internal CO2 concentration (C), transpiration (D), instantaneous WUE (E), and vapor pressure deficit (F) of 6-week-old S. oleracea seedlings leaves under drought condition. Each value represents the mean ± SE (n = 6).
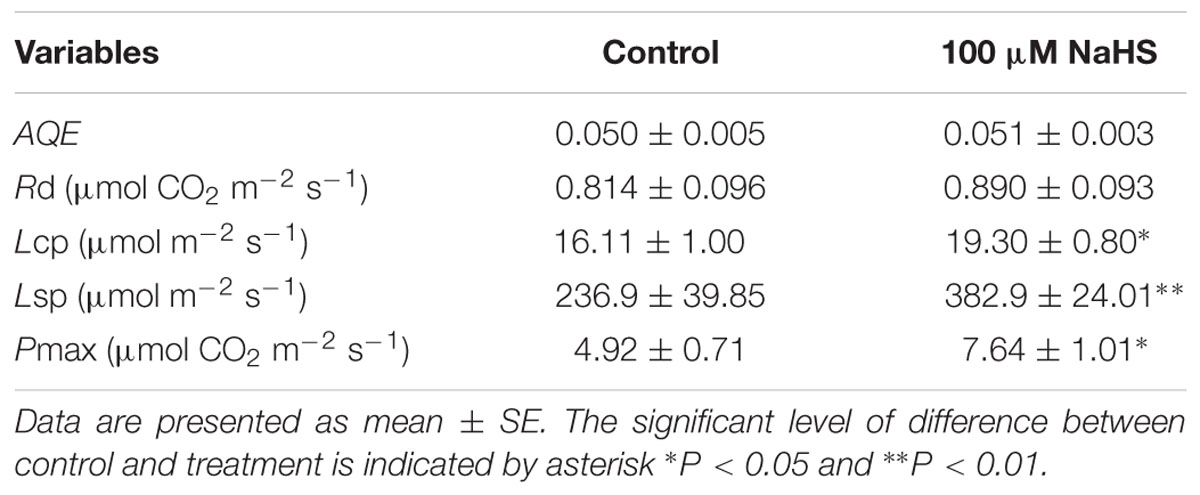
TABLE 1. Effects of H2S on apparent quantum yield (AQE), dark respiration (Rd), low light compensation point (Lcp), high light saturation point (Lsp), and maximum net photosynthetic rate (Pmax) of S. oleracea seedlings under drought condition.
Chlorophyll fluorescence is considered a tool for interpreting stress tolerance in plants. Therefore, we measured this key trait in S. oleracea seedlings under drought stress. Parameters indicative of chlorophyll fluorescence (PSII, ETR, Fv/Fm, Fv′/Fm′ and qP) were significantly decreased in the NaHS-treated and the control plants when the seedlings were under drought condition for 5 and 8 days compared with the water sufficient plants (0 day) (Figures 4A–D,F). Interestingly, PSII and Fv′/Fm′ in the NaHS-treated plants were higher than in the control plants on the first and fourth day after re-watering (Figures 4A,D). The ETR of the NaHS-treated plants was obviously increased compared with that of control plant throughout the entire treatment period, including 8 days of drought and 4 days of re-watering (Figure 4B). In contrast, NPQ in the NaHS-treated plants was significantly decreased compared with that of control plants throughout the entire treatment period (Figure 4E). The above results show that compared with the control plants, the NaHS-treated S. oleracea plants exhibited the high electron transport efficiency under drought condition.
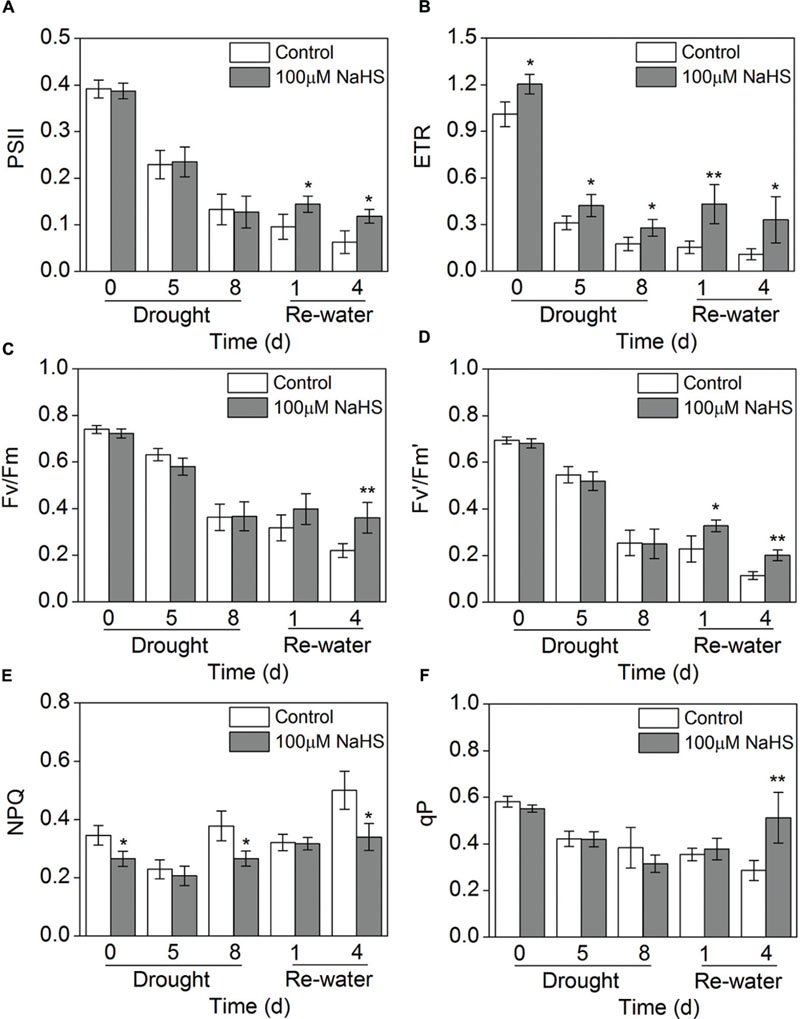
FIGURE 4. Effect of H2S donor NaHS on PSII (A), ETR (B), Fv/Fm (C), Fv′/Fm′(D), NPQ (E) and qP (F) in 6-week-old S. oleracea seedlings leaves after drought for 0, 5, and 8 days and rewatering for 1 and 4 days. The significant level of the difference between control and treatment is indicated by an asterisk ∗P< 0.05 and ∗∗P< 0.01.
Effect of H2S Donor on Leaf Water Potential and Osmotic Potential
The leaf water potential of the NaHS-treated plants and the control plants grown under drought condition underwent a significant decrease, especially on the eighth day after drought, when the NaHS-treated plant exhibited a higher leaf water potential than the control plants. After re-watering, the leaf water potential experienced a sharp increase in the NaHS-treated and control plants, and the NaHS-treated plants again showed a higher leaf water potential than the control plants (Figure 5A). However, the leaf osmotic potential of the NaHS-treated plants and the control plants showed no significant difference under drought and re-watering conditions except for the drought at 0 and 8 days (Figure 5B).
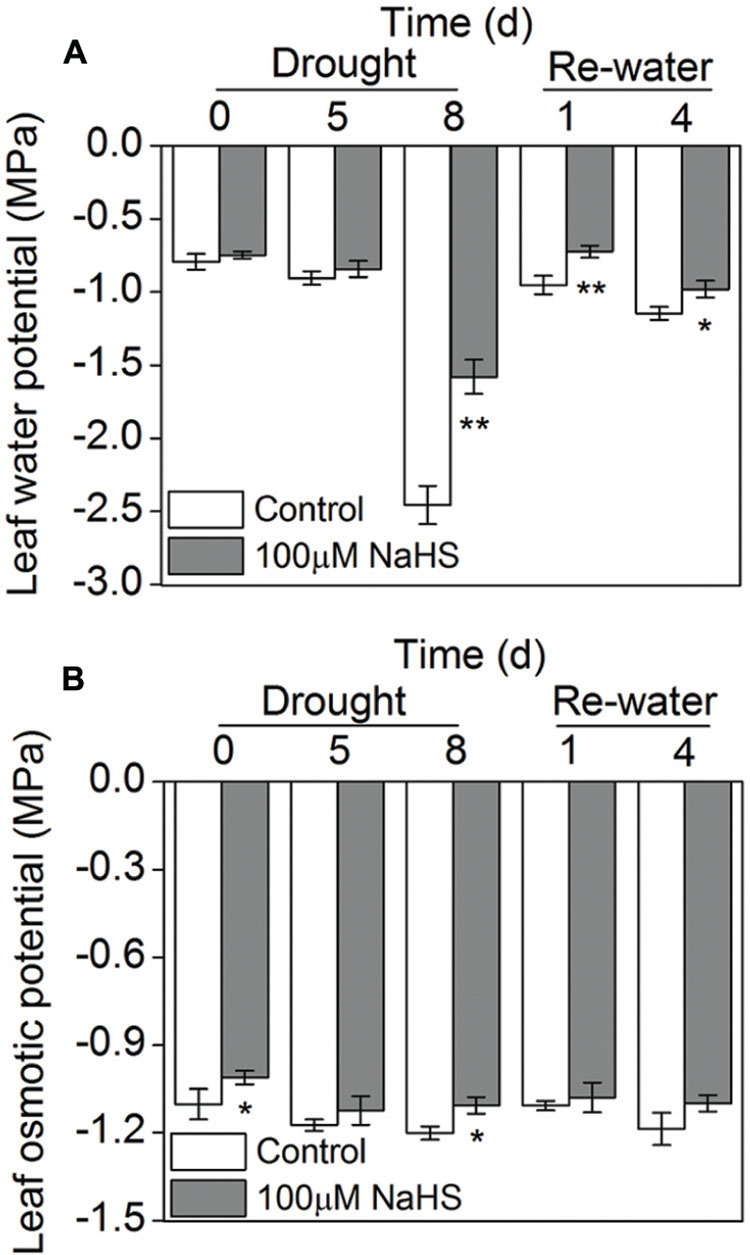
FIGURE 5. Effect of H2S donor NaHS on leaf water potential (A) and leaf osmotic potential (B) in 6-week-old S. oleracea seedlings leaves after drought for 0, 5, and 8 days and rewatering for 1 and 4 days. The significant level of the difference between control and treatment is indicated by an asterisk ∗P< 0.05 and ∗∗P< 0.01.
Effect of H2S Donor on Superoxide Radical Production, Hydrogen Peroxide Production, and Lipid Peroxidation Content
To further confirm the protective role of H2S in the plant response to drought stress, some simple physiological parameters were measured in S. oleracea seedlings. The production rate of superoxide radical () had no obvious difference between the NaHS-treated plants and the control plants under drought condition. However, on the first day after re-watering, the content significantly decreased in the NaHS-treated plants (Figure 6A). Additionally, there was a significant decrease in the accumulation of H2O2 in NaHS-treated plants compared with the control plants throughout the treatment period, except for 4 days after re-watering (Figure 6B). Similarly, under drought condition, the content of lipid peroxidation (MDA) underwent a sharp decrease in the NaHS-treated plants compared with the control plants, but the MDA content was not significantly different between treatments after re-watering (Figure 6C). These results show that H2S can protect S. oleracea seedlings against drought through the inhibition of oxidative stress.
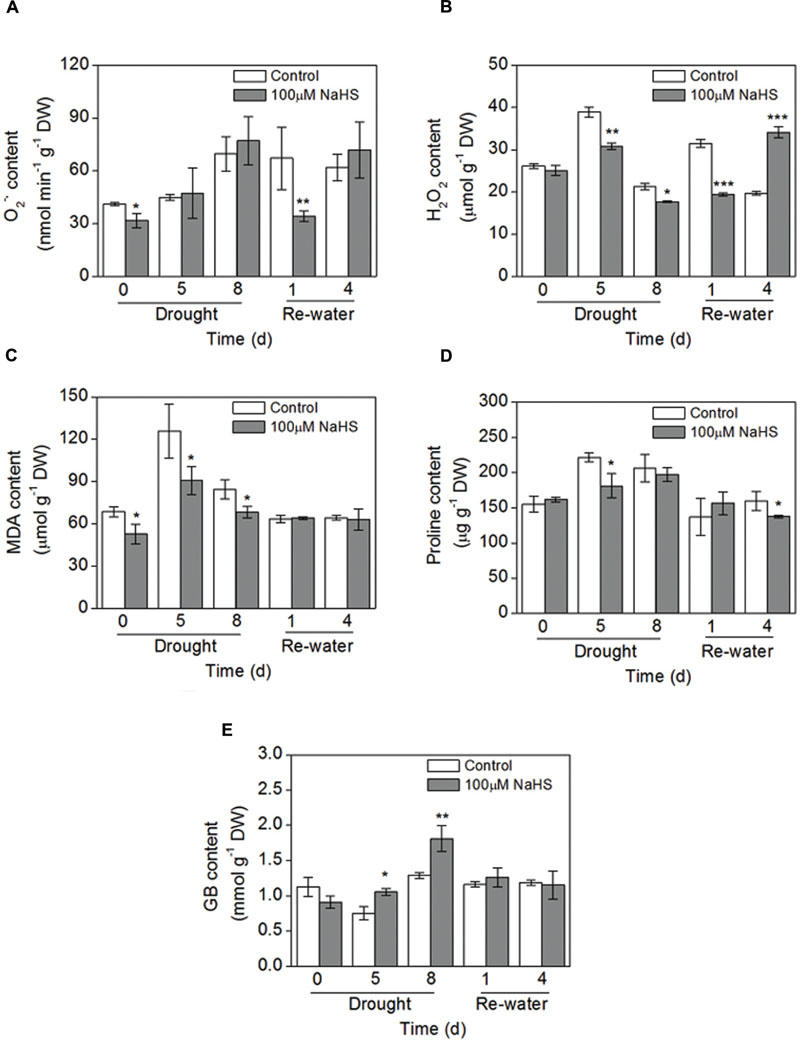
FIGURE 6. Effect of H2S donor NaHS on the production rate of (A), H2O2 content (B), MDA content (C), proline content (D) and glycinebetaine (GB) content (E) in 6-week-old S. oleracea seedlings leaves after drought for 0, 5, and 8 days and rewatering for 1 and 4 days. The significant level of the difference between control and treatment is indicated by an asterisk ∗P< 0.05, ∗∗P< 0.01, and ∗∗∗P< 0.001.
Effect of H2S Donor on Proline and GB Content
NaHS treatment had no obvious effect on the proline content under drought condition, but on the fourth day after re-watering the proline content had an obvious decrease in the NaHS-treated plants (Figure 6D). Furthermore, GB content in NaHS-treated plants had obvious increase under drought stress for 5 and 8 days compared with the control plants, respectively (Figure 6E). However, NaHS had no significant effect on the GB content under re-watering for 1 and 4 days compared with the control plants, respectively (Figure 6E). Besides, we also found that drought stress for 8 days could increase the GB content of S. oleracea seedlings leaves compared with the control plants (0 day) (Figure 6E).
Effect of H2S Donor on Sugar Biosynthesis
The glucose content in the NaHS-treated plants decreased to varying degrees compared with the control plants under drought and re-watering conditions (Figure 7A). In contrast, the fructose content in the NaHS-treated plants increased considerably compared with that of control plants under the same drought and re-watering conditions (Figure 7B). The sucrose content in the NaHS-treated plants decreased significantly compared with that of control plants under drought condition, but the content of this sugar increased notably on the first day of re-watering (Figure 7C). Similarly, the trehalose content in the NaHS-treated plants experienced a significant decrease compared with the control plants under drought condition, but after re-watering the trehalose content sharply increased in the NaHS-treated plants (Figure 7D).
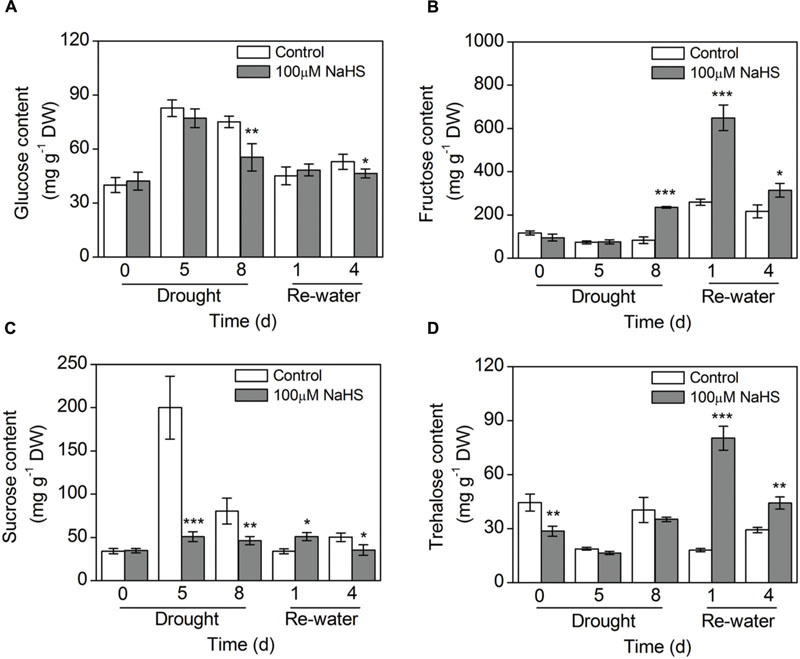
FIGURE 7. Effect of H2S donor NaHS on glucose content (A), fructose content (B), sucrose content (C), and trehalose content (D) of 6-week-old S. oleracea seedlings leaves after drought for 0, 5, and 8 d, and rewatering for 1 and 4 days. The significant level of the difference between control and treatment is indicated by an asterisk ∗P< 0.05, ∗∗P< 0.01, and ∗∗∗P< 0.001.
Effect of H2S Donor on Polyamine Biosynthesis
The concentration of total free and conjugated polyamines (PAs) was increased in leaves of the NaHS-treated plants compared with that of control plants under similar drought and re-watering conditions (Figure 8D; Supplementary Figure S2D). In particular, this difference was most evident on the fourth day of re-watering (Figure 8D). Moreover, the free Put content was significantly increased in the NaHS-treated plants after 5 days of drought and 1 day of re-watering (Figure 8A). Similarly, the free Spd content increased substantially in the NaHS-treated plants after 5 days of drought and 1 day of re-watering (Figure 8B). In contrast, NaHS treatment significantly decreased the free Spm content after 5 days of drought, but on the fourth day of re-watering, the Spm content increased notably (Figure 8C). Moreover, the conjugated Put content was significantly increased in the NaHS-treated plants after 1 and 4 days of re-watering (Supplementary Figure S2A). Similarly, the conjugated Spd content increased notably in the NaHS-treated plants under drought and re-watering conditions (Supplementary Figure S2B). Additionally, NaHS treatment altered the conjugated Spm content under drought and re-watering conditions (Supplementary Figure S2C).
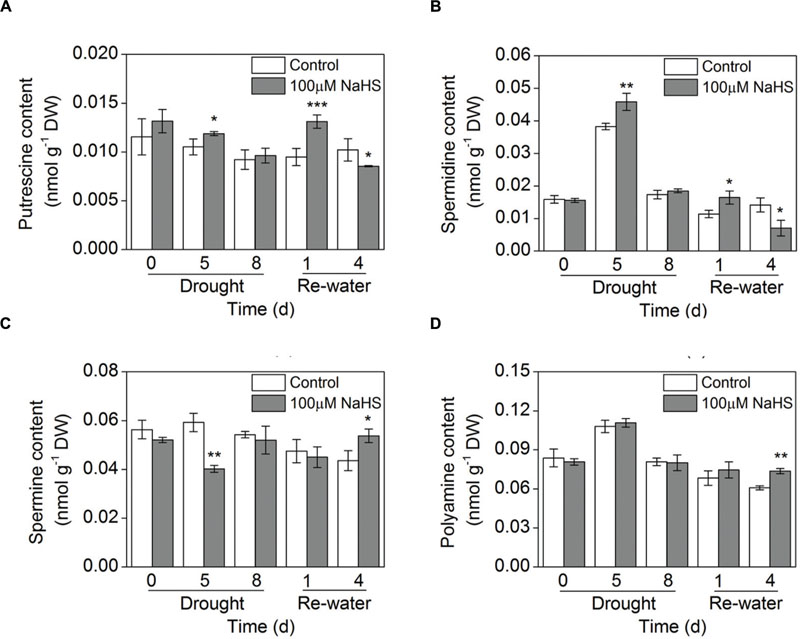
FIGURE 8. Effect of H2S donor NaHS on free polyamine including putrescine content (A), spermidine content (B), spermine content (C), and the total polyamine content (D) of 6-week-old S. oleracea seedlings leaves after drought for 0, 5, and 8 d and rewatering for 1 and 4 days. The significant level of the difference between control and treatment is indicated by an asterisk ∗P< 0.05, ∗∗P< 0.01, and ∗∗∗P< 0.001.
Effect of H2S Donor on Transcriptional Expression of Sugar Biosynthesis-Related Genes
To further study the molecular mechanism of H2S-alleviated drought stress in plants, we examined the expression levels of the sugar biosynthesis-related genes, GB biosynthesis-related genes such as SoBADH, SoCMO, and SoPIP1;2 using RT-qPCR analysis in S. oleracea seedlings. The expression level of sucrose phosphate synthase (SoSPS1) in the NaHS-treated plants was significantly increased compared to that of control plants after 0 day of drought and 1 and 4 days of re-watering (Figure 9A). Additionally, the expression level of fructose-1,6-bisphosphatase (SoFBPase) increased to varying degrees in the NaHS-treated plants (Figure 9B). Moreover, the expression level of trehalose-6-phosphate synthase (SoT6PS) increased clearly in the NaHS-treated plants after 5 days of drought, but significantly decreased after 1 day of re-watering (Figure 9C). Furthermore, the expression level of SoBADH in the NaHS-treated plants increased significantly compared with that of control plants after 8 days of drought and 1 and 4 day of re-watering, but the expression level of SoBADH decreased sharply in the NaHS-treated plants after 5 days of drought (Figure 9D). Additionally, the expression abundance of SoCMO in the NaHS-treated plants was evidently enhanced compared with that in control plants after 8 days of drought and 4 days of re-watering, but no significant difference were detected during other treatment periods (Figure 9E). Interestingly, the expression level of SoPIP1;2 in the NaHS-treated plants was significantly increased compared with that in the control plants after 5 and 8 days of drought, and an analogous result was also observed after 1 and 4 days of re-watering (Figure 9F).
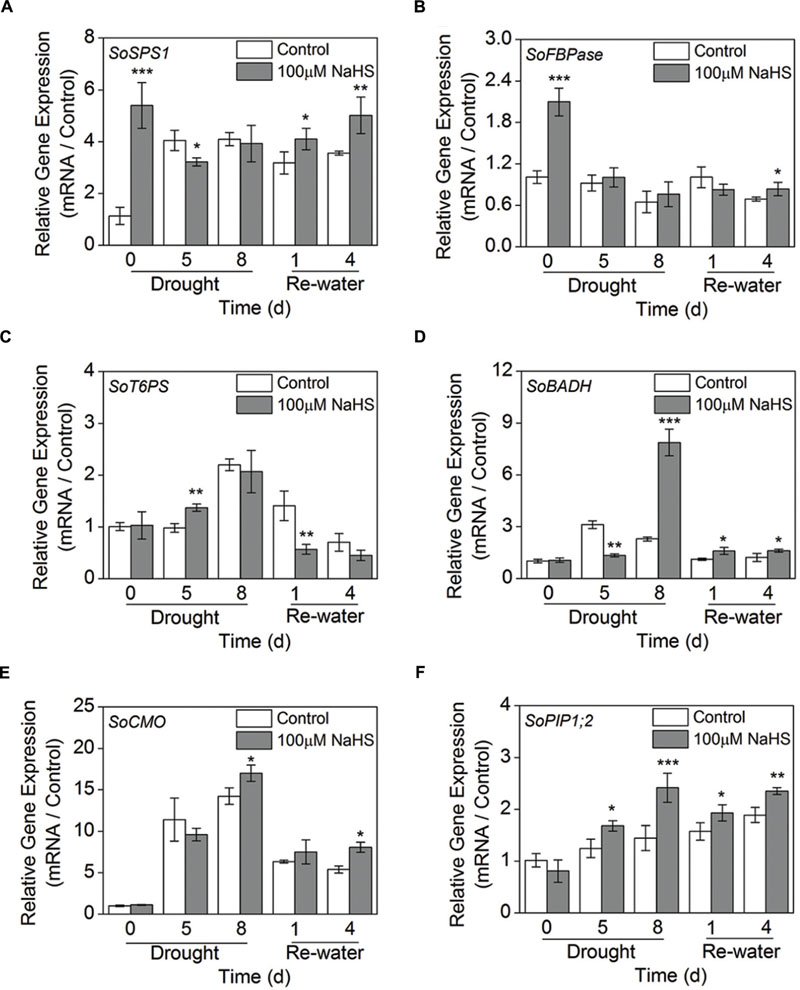
FIGURE 9. Effect of H2S donor NaHS on the relative transcript abundance of SoSPS1 (A), SoFBPase (B), SoT6PS (C), SoBADH (D), SoCMO (E), and SoPIP1;2 (F) mRNA accumulation in the leaves of S. oleracea seedlings after drought for 0, 5, and 8 days and rewatering for 1 and 4 days. Transcriptional expression of the six genes was measured using real-time qPCR and were normalized with a reference gene (SoGADPH). Mean values ± SE were calculated from three independent experiments. The significant level of the difference between control and treatment is indicated by an asterisk ∗P< 0.05, ∗∗P< 0.01, and ∗∗∗P< 0.001.
Effect of H2S Donor on Transcriptional Expression of Polyamines Biosynthesis-Related Genes
We examined the expression levels of 5 genes involved in PA biosynthesis in S. oleracea leaves: arginine decarboxylase (SoADC), N-carbamoylputrescine amidohydrolase (SoCPA), ornithine decarboxylase (SoODC), S-adenosyl-Met-decarboxylase (SoSAMD), and spermidine synthase (SoSPDS) using RT-qPCR analysis. The expression level of SoADC significantly increased in the NaHS-treated plants compared with the control plants after 0, 5, and 8 days of drought, and a comparable result was also observed after 4 days of re-watering (Figure 10A). Moreover, NaHS treatment significantly increased the expression level of SoCPA throughout the experiment in S. oleracea seedlings (Figure 10B). Similarly, NaHS treatment also clearly increased the expression level of SoODC after 0 and 8 days of drought and 4 days of re-watering in S. oleracea seedlings (Figure 10C). On the contrary, the expression level of SoSAMD had a significant decrease in the NaHS-treated plants after 0 and 5 days of drought, but no significant difference was observed at other treatment periods (Figure 10D). Furthermore, the expression level of SoSPDS in the NaHS-treated S. oleracea seedlings increased to varying degrees compared with that of the control plants, particularly after 8 days of drought (Figure 10E).
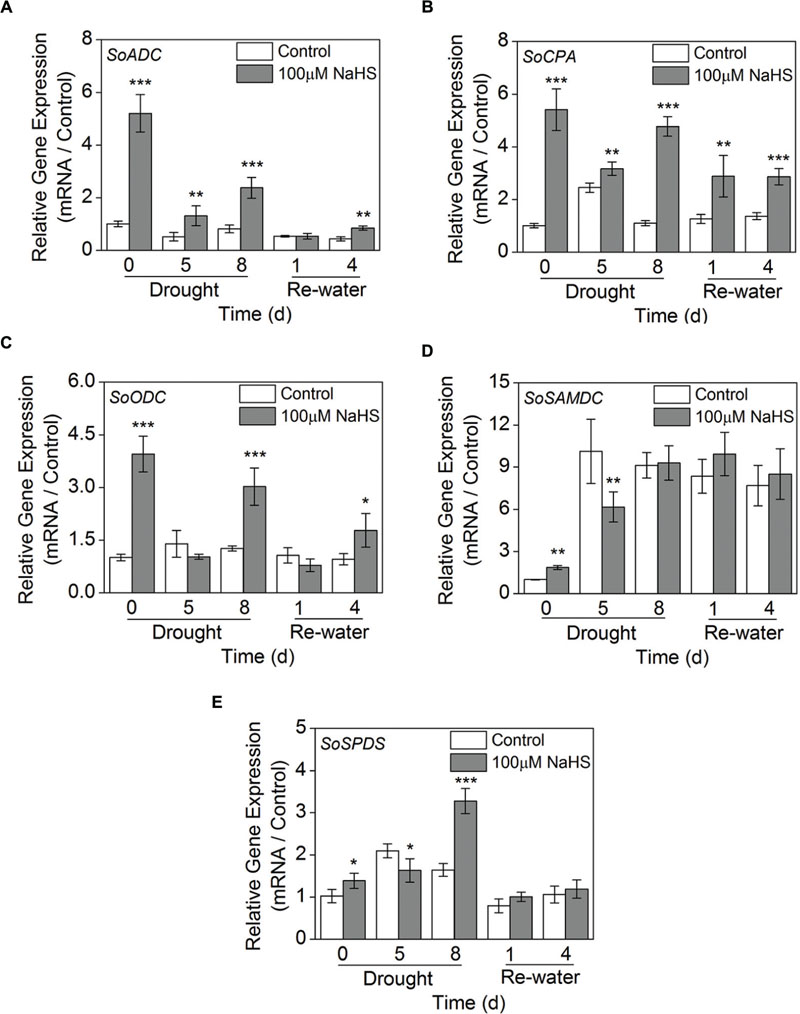
FIGURE 10. Effect of H2S donor NaHS on the relative transcript abundance of SoADC (A), SoCPA (B), SoODC (C), SoSAMDC (D), and SoSPDS (E) mRNA accumulation in the leaves of S. oleracea seedlings after drought for 0, 5, and 8 days and rewatering for 1 and 4 days. Transcriptional expression of the six genes was measured using real-time qPCR and were normalized with a reference gene (SoGADPH). Mean values ± SE were calculated from three independent experiments. The significant level of the difference between control and treatment is indicated by an asterisk ∗P< 0.05, ∗∗P< 0.01, and ∗∗∗P< 0.001.
Discussion
H2S Attenuates Drought Stress-Induced Water Scarcity
Plants have evolved a variety of strategies to resist or avoid drought stress and reduce the damage caused by lack of water. These strategies include regulating stomatal closure and reducing transpiration, inhibiting the growth rates of leaves or stems, maintaining or increasing the hydraulic conductance of roots and shoots, synthesizing osmotic solutes to maintain cell turgor, and producing antioxidant proteins to retard chlorophyll decomposition (Wilkinson and Davies, 2010). In the present study, under drought stress condition, the survival ratio of plants, the leaf RWC, and photosynthesis were higher in the NaHS-treated plants than in the control plants (Figures 1 and 3, Supplementary Figure S1). In most plants, the essential goals of drought resistance strategies are to increase water uptake from roots or reduce water loss from the leaves (Yin et al., 2013). In the present study, NaHS-treated plants exhibited significantly less water loss than the control plants (Supplementary Figures S1C,D). Moreover, although the transpiration rate and stomatal conductance were higher in the NaHS-treated plants than in the control plants, the NaHS-treated plants were able to maintain a higher leaf RWC than the control plants, indicating that the alleviation of water stress was not due to the regulation of stomatal closure in this study (Figures 1C and 3B,D). In this study, under drought stress, the NaHS-treated plants exhibited a high photosynthetic rate compared with the control plants. Under drought stress, the H2S concentration was found that to be higher in NaHS-treated plants than in the untreated plants (Figure 2). These results show that NaHS application enhanced S. oleracea seedling resistance to drought stress. Thus, our results indicate that H2S controlled plant the RWC of plant leaves under drought stress. Comparable results have also been observed with silicon, but although silicon alleviated drought stress, the RWC and stomatal conductance were higher in silicon-treated plants than in the untreated plants (Yin et al., 2013). Importantly, in contrast to the above finding, Jin et al. (2013) reported that H2S plays a vital role in the regulation of stomatal closure in the plant response to drought stress. Some possible explanations for this discrepancy include the identity of the plant species, the stress applied, the treatment time and the specific environmental conditions used. The above results showed that H2S can attenuate drought stress in S. oleracea seedlings. However, the precise mechanism by which H2S attenuates drought stress in S. oleracea seedlings remains unclear.
H2S Enhances the Tolerance of Plants to Drought Stress by Increasing Osmoprotectants Content
Under stress condition, plants that have evolved in high-temperature, saline or drought environments usually accumulate three types of osmoprotectants: non-structural sugars and polyols, betaines and allied compounds, and amino acids such as proline. A previous study indicated that salinity decreased cell expansion in tomato plants, which was related to decreases in osmotic and water potentials (Romero-Aranda et al., 2001). These results are consistent with those observed in our experiments (Figures 5A,B). The specific composition of osmoprotectants in plant leaves under drought conditions could be implicated in the specific response observed here. In the present study, the accumulation of soluble sugars, including glucose and sucrose, in the NaHS-treated S. oleracea leaves was lower than in the control plants, but the fructose and trehalose contents were significantly increased compared with those of the control plants, suggesting that NaHS enhances drought resistance by regulating the accumulation of soluble sugars, especially the contents of fructose and trehalose (Figure 7). However, under drought and re-watering condition, the expression levels of SoSPS1 and SoFBPase in the NaHS-treated plants increased to varying degrees compared with those in the control plants (Figures 9A,B). Similarly, Rivero et al. (2014) reported that salt and heat treatment specifically up-regulated the expression levels of the FBPase gene. Moreover, the function of this enzyme in the pathway of gluconeogenesis may be a limiting step in the synthesis of starch and trehalose because the activity of FBPase is very important for glucose regeneration, which is the main carbon skeleton in trehalose and starch (Rivero et al., 2014).
Additionally, the function of trehalose in stress response remains controversial, though it has been shown stabilize membranes and protect proteins in desiccated tissues and has been suggested to function as a chemical chaperone (Rivero et al., 2014). However, trehalose levels in nature and even in engineered plants usually remain well below 1 mg g-1 FW, suggesting that trehalose does not act as a compatible solute, but has an alternative function (Garg et al., 2002). The molecular relevance and roles that have been proposed and partially demonstrated for trehalose in plants have been reviewed by Ponnu et al. (2011). Additionally, trehalose-6-phosphate (T6P), a precursor of trehalose, has been proven to play a key role in regulating carbohydrate metabolism by inducing starch synthesis in the plastid. Furthermore, trehalose has also been linked to, plant development, cell growth and the induction of photosynthesis capacity. Previous studies have shown that a decrease in the T6P concentration results in the induction of genes involved in photosynthesis-related processes, so that more carbon is used for the growing cell, to supplement the lack of carbon (Ponnu et al., 2011). In the present study, the trehalose content in the NaHS-treated plants significantly increased after 1 and 4 days of re-watering (Figure 7D). Moreover, the NaHS-treated plants exhibited obvious increases in photosynthesis and PSII efficiency compared with the control plants. Our results show that the increased in photosynthesis capacity and PSII efficiency may also be explained by the regulatory role of trehalose during this process. Furthermore, Garg et al. (2002) suggested that a modulated carbohydrate metabolism could explain the lowered photo-oxidative damage associated with the high trehalose levels, which is consistent with our findings.
It is well established that various abiotic stresses such as salinity, drought, cold, and high-temperatures can lead to the accumulation of ROS. Osmoprotective compounds can directly remove ROS or cause the protection of antioxidant enzymes. In this regard, proline has been found to be a singlet oxygen quencher during osmotic stress because it can reduce the damage of ROS in different plants. As an example, the soaking of buds in proline and GB solutions greatly reduces the production of H2O2, improves the accumulation of soluble sugar and protects the developing tissues from the effects of stress. Similarly, our results also showed that the NaHS-treated plants could accumulate high GB contents and the up-regulated expression of the SoBADH and SoCMO genes under drought conditions (Figures 6E and 9D,E), which result in lower H2O2 accumulation and MDA contents in S. oleracea leaves (Figures 6B,C). However, NaHS treatment had no significant effect on the content of proline in S. oleracea seedlings (Figure 6D). A possible explanation for this discrepancy is that proline accumulation results from the activation of its synthesis and an inhibition of its degradation at both the transcriptional and post-transcriptional levels. Therefore, our results indicate that NaHS plays an important role in protecting plants from drought stress by influencing the accumulation of osmoprotective compounds and related gene expression.
H2S Enhances the Tolerance of Plants to Drought Stress by Increasing Polyamine Biosynthesis
Changes in PA levels and the accumulation of osmoprotective compounds are a common plant response to the lack of water and are associated with plant drought tolerance (Yin et al., 2014). The role of PAs in enhancing drought tolerance has been determined such as increasing the ability of antioxidants, taking effect as stress-signaling regulators, inducing stomatal closure and enhancing leaf water content (Shi et al., 2010; Yin et al., 2014; Muilu-Makela et al., 2015). In addition, the activation of ADC and/or ODC may also stimulate PA biosynthesis in plant tissues because these two enzymes catalyzed the production of Put. Generally, under stress conditions, the pathway of ADC is easily activated; in contrast, ODC is essential for cell proliferation and the biosynthesis of root Put (Tiburcio et al., 2014). Additionally, in this study, the application of NaHS under drought condition resulted in the increased biosynthesis of free and conjugated PAs biosynthesis (Figure 8; Supplementary Figure S2). Moreover, to further study how H2S regulates PA biosynthesis, the transcriptional abundances of PA biosynthesis-related genes were determined. Our results revealed that the transcription level of SoADC was up-regulated by NaHS under drought condition (Figure 10A). Many previous studies have reported that the ADC pathway is closely related to drought stress and that ADC can regulate the biosynthesis of Put (Yin et al., 2014; Lulai et al., 2015). In fact, the two enzymes agmatine iminohydrolase (AIH) and CPA are also involved in Put biosynthesis in the ADC pathway. Here, the expression level of SoCPA was up-regulated by NaHS (Figure 10B). Interestingly, the transcription levels of SoODC were significantly increased in the NaHS-treated plants grown under drought condition (Figure 10C). Accordingly, these results show that H2S mediated Put production through both the ADC and ODC pathways in S. oleracea seedlings. Additionally, SAMDC is a rate-limiting enzyme in Spd and Spm biosynthesis, because SAMDC has a short half-life. The transcriptional level of SAMDC is induced under different abiotic stresses, and the overexpression of SAMDC increases PA levels and enhances stress tolerance. However, in this study, the transcription of SoSAMDC in the NaHS-treated plants was up-regulated under normal condition, but was down-regulated by NaHS after 5 days of drought, and there was no obvious difference between the treatment periods (Figure 10D). Notably, the Spd level increased markedly after 5 days of drought, whereas the Spm level decreased significantly, suggesting that SAMDC expression did not correlate with the levels of Spd/or Spm, probably as a result of the tight regulation of enzyme activity (Figures 8B,D). However, it is of note that the transcription level of SoSPDS in the NaHS-treated plants underwent a significant increase under drought condition (Figure 10E). These results may explain the changes in the Spd and Spm levels observed under drought or re-watering conditions.
Abiotic stress often enhances senescence processes, where valuable resources, accumulated during prior development are recycled for use in other growing parts of the plant. Additionally, previous studies have shown that the increase of PA contents may prevent chlorophyll loss and the inhibition of photosynthesis and thus delay leaf senescence under stress conditions (Shi et al., 2010; Glaubitz et al., 2015). In this study, under drought condition, NaHS was observed to significantly increase photosynthesis in the plants (Figure 3A). Meanwhile, PA contents were also increased to varying degrees, which suggests that PAs may play an important role in the maintenance of photosynthesis and plant growth and additionally may participate in H2S-induced drought tolerance. In contrast, if senescence leads to programmed cell death, PAs may play a vital role as a carbon and nitrogen reservoir in plants under stress condition (Moschou et al., 2012). Therefore, PA homoeostasis may be was regulated by H2S to protect plants from drought damage.
Pathway of H2S-Induced Drought Resistance
Based on the above results and the current knowledge concerning the mechanisms of plant response to drought stress, a signaling pathway by which H2S influences the biosynthesis of total PAs and sugar has been proposed. As shown in Figure 11, H2S may promote the growth and photosynthesis of plants under drought stress. Under drought stress, H2S up-regulates the transcription levels of SoADC, SoCPA, SoODC, and SoSPDS, leading to the increased biosynthesis and accumulation of PAs in plant tissues. Under drought stress, H2S may also increase the accumulation of osmoprotective compounds including soluble sugars by changing the expression levels of sugar biosynthesis-related genes. Together, high levels of PAs and soluble sugars enhance drought tolerance in plants. Our results show that H2S regulates drought stress response in plants by mediating certain important metabolic processes and is not limited to a mere mechanical barrier, as was previously assumed. Importantly, our study also opens new avenues of research for the growth and development of plants with enhanced tolerance to abiotic stress conditions.
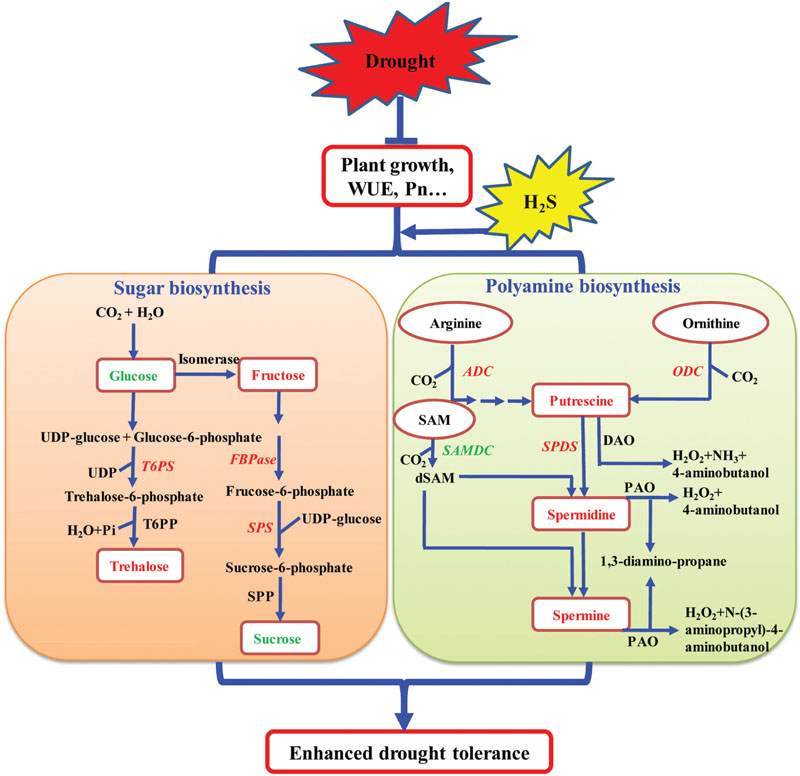
FIGURE 11. A model depicting how H2S regulates the enhanced drought tolerance by mediating polyamine and sugar biosynthesis. Under drought stress, on the one hand, the application of NaHS up-regulated the expression of polyamine biosynthetic genes encoding arginine decarboxylase (ADC), ornithine decarboxylase (ODC), and N-carbamoylputrescine amidohydrolase (CPA) (indicated by red and bold font) and down-regulated the expression of S- adenosyl-Met-decarboxylase (SAMDC) (indicated by green and bold font), resulting in elevated polyamine levels in plant tissues. On the other hand, the application of NaHS up-regulated the expression of sugar biosynthetic genes encoding trehalose-6-phosphate synthase (T6PS), fructose-1,6-bisphosphatase (FBPase), and sucrose phosphate synthase (SPS1) (indicated by red and bold font), resulting in the alteration of the sugar content, including elevation of the fructose and trehalose content (indicated by red and bold font) and reduction of the glucose and sucrose content (indicated by green and bold font) in plant tissues.
Author Contributions
JC designed the experiment and wrote the manuscript, Y-TS and X-YC conducted the experiment, W-HW and E-MH helped in data analysis and presentation, and ZS and H-LZ revised the manuscript.
Supplementary Material
The Supplementary Material for this article can be found online at: http://journal.frontiersin.org/article/10.3389/fpls.2016.01173
Conflict of Interest Statement
The authors declare that the research was conducted in the absence of any commercial or financial relationships that could be construed as a potential conflict of interest.
Acknowledgments
We are grateful to Bing-Bo Li for valuable assistance with the experiments. This study was financially supported by the Natural Science Foundation of China (NSFC) (31501822 and 31401362), the Postdoctoral Science Foundation of China (2015M580876 and 2016T90948).
References
Alcázar, R., Altabella, T., Marco, F., Bortolotti, C., Reymond, M., Koncz, C., et al. (2010a). Polyamines: molecules with regulatory functions in plant abiotic stress tolerance. Planta 231, 1237–1249. doi: 10.1007/s00425-010-1130-0
Alcázar, R., Planas, J., Saxena, T., Zarza, X., Bortolotti, C., Cuevas, J., et al. (2010b). Putrescine accumulation confers drought tolerance in transgenic Arabidopsis plants over-expressing the homologous Arginine decarboxylase 2 gene. Plant Physiol. Biochem. 48, 547–552. doi: 10.1016/j.plaphy.2010.02.002
Alexieva, V., Sergiev, I., Mapelli, S., and Karanov, E. (2001). The effect of drought and ultraviolet radiation on growth and stress markers in pea and wheat. Plant Cell Environ. 24, 1337–1344. doi: 10.1046/j.1365-3040.2001.00778.x
Bates, L. S., Waldren, R. P., and Teare, I. D. (1973). Rapid determination of free proline for water-stress studies. Plant Soil 39, 205–207. doi: 10.1007/BF00018060
Bessieres, M. A., Gibbon, Y., Lefeuvre, J. C., and Larher, F. (1999). A single-step purification for glycine betaine determination in plant extracts by isocratic HPLC. J. Agric. Food. Chem. 47, 3718–3722. doi: 10.1021/jf990031h
Blum, A. (1996). Crop responses to drought and the interpretation of adaptation. Plant Growth Regul. 20, 135–148. doi: 10.1007/BF00024010
Capell, T., Bassie, L., and Christou, P. (2004). Modulation of the polyamine biosynthetic pathway in transgenic rice confers tolerance to drought stress. Proc. Natl. Acad. Sci. U.S.A. 101, 9909–9914. doi: 10.1073/pnas.0306974101
Chaves, M. M., Maroco, J. P., and Pereira, J. S. (2003). Understanding plant responses to drought – from genes to the whole plant. Funct. Plant Biol. 30, 239–264. doi: 10.1071/FP02076
Chen, J., Wang, W. H., Wu, F. H., He, E. M., Liu, X., Shangguan, Z. P., et al. (2015a). Hydrogen sulfide enhances salt tolerance through nitric oxide-mediated maintenance of ion homeostasis in barley seedling roots. Sci. Rep. 5:12516. doi: 10.1038/srep12516
Chen, J., Wang, W. H., Wu, F. H., You, C. Y., Liu, T. W., Dong, X. J., et al. (2013). Hydrogen sulfide alleviates aluminum toxicity in barley seedlings. Plant Soil 362, 301–318. doi: 10.1007/s11104-012-1275-7
Chen, J., Wu, F. H., Shang, Y. T., Wang, W. H., Hu, W. J., Simon, M., et al. (2015b). Hydrogen sulphide improves adaptation of Zea mays seedlings to iron deficiency. J. Exp. Bot. 66, 6605–6622. doi: 10.1093/jxb/erv368
Chen, J., Wu, F. H., Wang, W. H., Zheng, C. J., Lin, G. H., Dong, X. J., et al. (2011). Hydrogen sulphide enhances photosynthesis through promoting chloroplast biogenesis, photosynthetic enzyme expression, and thiol redox modification in Spinacia oleracea seedlings. J. Exp. Bot. 62, 4481–4493. doi: 10.1093/jxb/err145
Dhindsa, R. S., Plumb-Dhindsa, P., and Thorpe, T. A. (1981). Leaf senescence: correlated with increased levels of membrane permeability and lipid peroxidation, and decreased levels of superoxide dismutase and catalase. J. Exp. Bot. 32, 93–101. doi: 10.1093/jxb/32.1.93
Duan, J. J., Li, J., Guo, S. R., and Kang, Y. Y. (2008). Exogenous spermidine affects polyamine metabolism in salinity-stressed Cucumis sativus roots and enhances short-term salinity tolerance. J. Plant Physiol. 165, 1620–1635. doi: 10.1016/j.jplph.2007.11.006
Flores, H. E., and Galston, A. W. (1982). Analysis of polyamines in higher plants by high performance liquid chromatography. Plant Physiol. 69, 701–706. doi: 10.1104/pp.69.3.701
García-Mata, C., and Lamattina, L. (2001). Nitric oxide induces stomatal closure and enhances the adaptive plant responses against drought stress. Plant Physiol. 126, 1196–1204. doi: 10.1104/pp.126.3.1196
García-Mata, C., and Lamattina, L. (2010). Hydrogen sulphide, a novel gasotransmitter involved in guard cell signalling. New Phytol. 188, 977–984. doi: 10.1111/j.1469-8137.2010.03465.x
Garg, A. K., Kim, J. K., Owens, T. G., Ranwala, A. P., Choi, Y. D., Kochian, L. V., et al. (2002). Trehalose accumulation in rice plants confers high tolerance levels to different abiotic stresses. Proc. Natl. Acad. Sci. U.S.A. 99, 15898–15903. doi: 10.1073/pnas.252637799
Glaubitz, U., Erban, A., Kopka, J., Hincha, D. K., and Zuther, E. (2015). High night temperature strongly impacts TCA cycle, amino acid and polyamine biosynthetic pathways in rice in a sensitivity-dependent manner. J. Exp. Bot. 66, 6385–6397. doi: 10.1093/jxb/erv352
Hosoki, R., Matsuki, N., and Kimura, H. (1997). The possible role of hydrogen sulfide as an endogenous smooth muscle relaxant in synergy with nitric oxide. Biochem. Biophys. Res. Commun. 237, 527–531. doi: 10.1006/bbrc.1997.6878
Jin, Z., Shen, J., Qiao, Z., Yang, G., Wang, R., and Pei, Y. (2011). Hydrogen sulfide improves drought resistance in Arabidopsis thaliana. Biochem. Biophys. Res. Commun. 414, 481–486. doi: 10.1016/j.bbrc.2011.09.090
Jin, Z., Xue, S., Luo, Y., Tian, B., Fang, H., Li, H., et al. (2013). Hydrogen sulfide interacting with abscisic acid in stomatal regulation responses to drought stress in Arabidopsis. Plant Physiol. Biochem. 62, 41–46. doi: 10.1016/j.plaphy.2012.10.017
Kasinathan, V., and Wingler, A. (2004). Effect of reduced arginine decarboxylase activity on salt tolerance and on polyamine formation during salt stress in Arabidopsis thaliana. Physiol. Plant 121, 101–107. doi: 10.1111/j.0031-9317.2004.00309.x
Krause, G., and Weis, E. (1991). Chlorophyll fluorescence and photosynthesis: the basics. Annu. Rev. Plant Biol. 42, 313–349. doi: 10.1146/annurev.pp.42.060191.001525
Li, D., Xiao, Z., Liu, L., Wang, J., Song, G., and Bi, Y. (2010). Effects of exogenous hydrogen sulfide (H2S) on the root tip and root border cells of Pisum sativum. Chin. Bull. Bot. 45, 354–362.
Li, H., Gao, M. Q., Xue, R. L., Wang, D., and Zhao, H. J. (2015). Effect of hydrogen sulfide on D1 protein in wheat under drought stress. Acta Physiol. Plant 37, 1–9. doi: 10.1007/s11738-015-1975-8
Li, L., Bhatia, M., and Moore, P. K. (2006). Hydrogen sulphide – a novel mediator of inflammation? Curr. Opin. Pharmacol. 6, 125–129. doi: 10.1016/j.coph.2005.10.007
Livak, K. J., and Schmittgen, T. D. (2001). Analysis of relative gene expression data using teal-time quantitative PCR and the 2-ΔΔCt method. Methods 25, 402–408. doi: 10.1006/meth.2001.1262
Lulai, E. C., Neubauer, J. D., Olson, L. L., and Suttle, J. C. (2015). Wounding induces changes in tuber polyamine content, polyamine metabolic gene expression, and enzyme activity during closing layer formation and initiation of wound periderm formation. J. Plant Physiol. 176, 89–95. doi: 10.1016/j.jplph.2014.12.010
Moschou, P. N., Wu, J., Cona, A., Tavladoraki, P., Angelini, R., and Roubelakis-Angelakis, K. A. (2012). The polyamines and their catabolic products are significant players in the turnover of nitrogenous molecules in plants. J.Exp. Bot. 63, 5003–5015. doi: 10.1093/jxb/ers202
Muilu-Makela, R., Vuosku, J., Laara, E., Saarinen, M., Heiskanen, J., Haggman, H., et al. (2015). Water availability influences morphology, mycorrhizal associations, PSII efficiency and polyamine metabolism at early growth phase of Scots pine seedlings. Plant Physiol. Biochem. 88, 70–81. doi: 10.1016/j.plaphy.2015.01.009
Peeva, V., and Cornic, G. (2009). Leaf photosynthesis of Haberlea rhodopensis before and during drought. Environ. Exp. Bot. 65, 310–318. doi: 10.1016/j.envexpbot.2008.09.009
Ponnu, J., Wahl, V., and Schmid, M. (2011). Trehalose-6-Phosphate: connecting plant metabolism and development. Front. Plant Sci. 2:70. doi: 10.3389/fpls.2011.00070
Prioul, J. L., and Chartier, P. (1977). Partitioning of transfer and carboxylation components of intracellular resistance to photosynthetic CO2 fixation: a critical analysis of the methods used. Ann. Bot. 41, 789–800.
Rivero, R. M., Mestre, T. C., Mittler, R. O. N., Rubio, F., Garcia-Sanchez, F., and Martinez, V. (2014). The combined effect of salinity and heat reveals a specific physiological, biochemical and molecular response in tomato plants. Plant Cell Environ. 37, 1059–1073. doi: 10.1111/pce.12199
Romero-Aranda, R., Soria, T., and Cuartero, J. (2001). Tomato plant-water uptake and plant-water relationships under saline growth conditions. Plant Sci. 160, 265–272. doi: 10.1016/S0168-9452(00)00388-5
Sekiya, J., Schmidt, A., Wilson, L. G., and Filner, P. (1982). Emission of hydrogen sulfide by leaf tissue in response to L-cysteine. Plant Physiol. 70, 430–436. doi: 10.1104/pp.70.2.430
Shan, C. J., Zhang, S. L., Li, D. F., Zhao, Y. Z., Tian, X. L., Zhao, X. L., et al. (2011). Effects of exogenous hydrogen sulfide on the ascorbate and glutathione metabolism in wheat seedlings leaves under water stress. Acta Physiol. Plant 33, 2533–2540. doi: 10.1007/s11738-011-0746-4
Shi, J., Fu, X. Z., Peng, T., Huang, X. S., Fan, Q. J., and Liu, J. H. (2010). Spermine pretreatment confers dehydration tolerance of citrus in vitro plants via modulation of antioxidative capacity and stomatal response. Tree Physiol. 30, 914–922. doi: 10.1093/treephys/tpq030
Takahashi, T., and Kakehi, J. I. (2010). Polyamines: ubiquitous polycations with unique roles in growth and stress responses. Ann. Bot. 105, 1–6. doi: 10.1093/aob/mcp259
Tiburcio, A., Altabella, T., Bitrián, M., and Alcázar, R. (2014). The roles of polyamines during the lifespan of plants: from development to stress. Planta 240, 1–18. doi: 10.1007/s00425-014-2055-9
Toumi, I., Moschou, P. N., Paschalidis, K. A., Bouamama, B., Ben Salem-fnayou, A., Ghorbel, A. W., et al. (2010). Abscisic acid signals reorientation of polyamine metabolism to orchestrate stress responses via the polyamine exodus pathway in grapevine. J. Plant Physiol. 167, 519–525. doi: 10.1016/j.jplph.2009.10.022
Tun, N. N., Santa-Catarina, C., Begum, T., Silveira, V., Handro, W., Floh, E. I. S., et al. (2006). Polyamines induce rapid biosynthesis of nitric oxide (NO) in Arabidopsis thaliana seedlings. Plant Cell Physiol. 47, 346–354. doi: 10.1093/pcp/pci252
Wang, A. G., and Luo, G. H. (1990). Quantitative relation between the reaction of hydroxylamine and superoxide anion radicals in plants. Plant Physiol. Commun. 84, 2895–2898.
Wang, B. L., Shi, L., Li, Y. X., and Zhang, W. H. (2010). Boron toxicity is alleviated by hydrogen sulfide in cucumber (Cucumis sativus L.) seedlings. Planta 231, 1301–1309. doi: 10.1007/s00425-010-1134-9
Wang, R. (2002). Two’s company, three’s a crowd: can H2S be the third endogenous gaseous transmitter? FASEB J. 16, 1792–1798. doi: 10.1096/fj.02-0211hyp
Wang, Y., Li, L., Cui, W., Xu, S., Shen, W., and Wang, R. (2012). Hydrogen sulfide enhances alfalfa (Medicago sativa) tolerance against salinity during seed germination by nitric oxide pathway. Plant Soil 351, 107–119. doi: 10.1007/s11104-011-0936-2
Wen, X. P., Pang, X. M., Matsuda, N., Kita, M., Inoue, H., Hao, Y. J., et al. (2008). Over-expression of the apple spermidine synthase gene in pear confers multiple abiotic stress tolerance by altering polyamine titers. Transgenic Res. 17, 251–263. doi: 10.1007/s11248-007-9098-7
Whittaker, J. B. (1994). Physiological responses of leaves of Rumex obtusifolius to damage by a leaf miner. Funct. Ecol. 8, 627–630. doi: 10.2307/2389925
Wilkinson, S., and Davies, W. J. (2010). Drought, ozone, ABA and ethylene: new insights from cell to plant to community. Plant Cell Environ. 33, 510–525. doi: 10.1111/j.1365-3040.2009.02052.x
Yamasaki, H., and Cohen, M. F. (2006). NO signal at the crossroads: polyamine-induced nitric oxide synthesis in plants? Trends Plant Sci. 11, 522–524. doi: 10.1016/j.tplants.2006.09.009
Yang, G., Wu, L., Jiang, B., Yang, W., Qi, J., Cao, K., et al. (2008). H2S as a physiologic vasorelaxant: hypertension in mice with deletion of cystathionine γ –Lyase. Science 322, 587–590. doi: 10.1126/science.1162667
Yin, L. N., Wang, S. W., Li, J. Y., Tanaka, K., and Oka, M. (2013). Application of silicon improves salt tolerance through ameliorating osmotic and ionic stresses in the seedling of Sorghum bicolor. Acta Physiol. Plant 35, 3099–3107. doi: 10.1007/s11738-013-1343-5
Yin, L. N., Wang, S. W., Liu, P., Wang, W. H., Cao, D., Deng, X. P., et al. (2014). Silicon-mediated changes in polyamine and 1-aminocyclopropane-1-carboxylic acid are involved in silicon-induced drought resistance in Sorghum bicolor L. Plant Physiol.Biochem. 80, 268–277. doi: 10.1016/j.plaphy.2014.04.014
Yin, L. N., Wang, S. W., Tanaka, K., Fujihara, S., Itai, A., Deng, X. P., et al. (2015). Silicon-mediated changes in polyamines participate in silicon-induced salt tolerance in Sorghum bicolor L. Plant Cell Environ. 39, 245–258. doi: 10.1111/pce.12521
Yoo, C. Y., Pence, H. E., Jin, J. B., Miura, K., Gosney, M. J., Hasegawa, P. M., et al. (2010). The Arabidopsis GTL1 transcription factor regulates water use efficiency and drought tolerance by modulating stomatal density via transrepression of SDD1. Plant Cell 22, 4128–4141. doi: 10.1105/tpc.110.078691
Zhang, H., Hu, L. Y., Hu, K. D., He, Y. D., Wang, S. H., and Luo, J. P. (2008). Hydrogen sulfide promotes wheat seed germination and alleviates oxidative damage against copper stress. J. Integr. Plant Biol. 50, 1518–1529. doi: 10.1111/j.1744-7909.2008.00769.x
Zhang, H., Hu, S. L., Zhang, Z. J., Hu, L. Y., Jiang, C. X., Wei, Z. J., et al. (2011). Hydrogen sulfide acts as a regulator of flower senescence in plants. Postharvest Biol. Technol. 60, 251–257. doi: 10.1016/j.postharvbio.2011.01.006
Zhang, H., Jiao, H., Jiang, C. X., Wang, S. H., Wei, Z. J., Luo, J. P., et al. (2010a). Hydrogen sulfide protects soybean seedlings against drought-induced oxidative stress. Acta Physiol. Plant 32, 849–857. doi: 10.1007/s11738-010-0469-y
Zhang, H., Tan, Z. Q., Hu, L. Y., Wang, S. H., Luo, J. P., and Jones, R. L. (2010b). Hydrogen sulfide alleviates aluminum toxicity in germinating wheat seedlings. J. Integr. Plant Biol. 52, 556–567. doi: 10.1111/j.1744-7909.2010.00946.x
Zhang, H., Ye, Y. K., Wang, S. H., Luo, J. P., Tang, J., and Ma, D. F. (2009). Hydrogen sulfide counteracts chlorophyll loss in sweetpotato seedling leaves and alleviates oxidative damage against osmotic stress. Plant Growth Regul. 58, 243–250. doi: 10.1007/s10725-009-9372-1
Zhao, T. Y., Thacker, R., Corum, J. W., Snyder, J. C., Meeley, R. B., Obendorf, R. L., et al. (2004). Expression of the maize GALACTINOL SYNTHASE gene family: (I) Expression of two different genes during seed development and germination. Physiol. Plant 121, 634–646. doi: 10.1111/j.1399-3054.2004.00367.x
Zhao, W., Zhang, J., Lu, Y., and Wang, R. (2001). The vasorelaxant effect of H2S as a novel endogenous gaseous KATP channel opener. EMBO J. 20, 6008–6016. doi: 10.1093/emboj/20.21.6008
Keywords: hydrogen sulfide, drought, soluble sugar, polyamines, water relations, Spinacia oleracea
Citation: Chen J, Shang Y-T, Wang W-H, Chen X-Y, He E-M, Zheng H-L and Shangguan Z (2016) Hydrogen Sulfide-Mediated Polyamines and Sugar Changes Are Involved in Hydrogen Sulfide-Induced Drought Tolerance in Spinacia oleracea Seedlings. Front. Plant Sci. 7:1173. doi: 10.3389/fpls.2016.01173
Received: 26 April 2016; Accepted: 21 July 2016;
Published: 04 August 2016.
Edited by:
Maren Müller, University of Barcelona, SpainReviewed by:
Vasileios Fotopoulos, Cyprus University of Technology, CyprusRavi Valluru, International Maize and Wheat Improvement Center, Mexico
Copyright © 2016 Chen, Shang, Wang, Chen, He, Zheng and Shangguan. This is an open-access article distributed under the terms of the Creative Commons Attribution License (CC BY). The use, distribution or reproduction in other forums is permitted, provided the original author(s) or licensor are credited and that the original publication in this journal is cited, in accordance with accepted academic practice. No use, distribution or reproduction is permitted which does not comply with these terms.
*Correspondence: Zhouping Shangguan, c2hhbmdndWFuQG1zLmlzd2MuYWMuY24=
†These authors have contributed equally to this work.