- 1Jiangsu Key Laboratory for Bioresources of Saline Soils, Provincial Key Laboratory of Agrobiology, Institute of Agro-biotechnology, Jiangsu Academy of Agricultural Sciences, Nanjing, China
- 2Department of Plant Physiology, Faculty of Agrobiology and Food Resources, Slovak University of Agriculture in Nitra, Nitra, Slovakia
- 3Department of Agrochemistry and Plant Nutrition, Faculty of Agrobiology and Food Resources, Slovak University of Agriculture in Nitra, Nitra, Slovakia
- 4Yantai Institute of Coastal Zone Research, Chinese Academy of Sciences, Yantai, China
Photosynthesis limitation by CO2 flow constraints from sub-stomatal cavities to carboxylation sites in chloroplasts under drought stress conditions is, at least in some plant species or crops not fully understood, yet. Leaf mesophyll conductance for CO2 (gm) may considerably affect both photosynthesis and water use efficiency (WUE) in plants under drought conditions. The aim of our study was to detect the responses of gm in leaves of four winter wheat (Triticum aestivum L.) genotypes from different origins under long-term progressive drought. Based on the measurement of gas-exchange parameters the variability of genotypic responses was analyzed at stomatal (stomata closure) and non-stomatal (diffusional and biochemical) limits of net CO2 assimilation rate (AN). In general, progressive drought caused an increasing leaf diffusion resistance against CO2 flow leading to the decrease of AN, gm and stomatal conductance (gs), respectively. Reduction of gm also led to inhibition of carboxylation efficiency (Vcmax). On the basis of achieved results a strong positive relationship between gm and gs was found out indicating a co-regulation and mutual independence of the relationship under the drought conditions. In severely stressed plants, the stomatal limitation of the CO2 assimilation rate was progressively increased, but to a less extent in comparison to gm, while a non-stomatal limitation became more dominant due to the prolonged drought. Mesophyll conductance (gm) seems to be a suitable mechanism and parameter for selection of improved diffusional properties and photosynthetic carbon assimilation in C3 plants, thus explaining their better photosynthetic performance at a whole plant level during periods of drought.
Introduction
At the global level, drought accompanied by low water availability in soils is considered the main environmental factor that limits plant growth and yield (Chaves et al., 2003; Nemani et al., 2003; Zhao et al., 2011). This combination may negatively affect the productivity of agricultural crops as well as natural ecosystems and the diversity of plant species (Zivcak et al., 2013). There are some strategies aimed at maintaining water resources in soils and plants, e.g., improvement of crop water use efficiency (WUE; Wang et al., 2002; Condon et al., 2004) and photosynthesis itself, which may increase crop yields in the near future (Parry et al., 2002; Flexas et al., 2013).
A water deficit develops in plants when water losses by evapotranspiration are inadequately replaced by the water flow from soil. In a natural environment, a water deficit occurs progressively from a week to months, depending upon the characteristics of the soil where the plants are grown (Cano et al., 2014). Water deficiency triggers many responses at different levels (molecular to whole plant) of plants in conditions of water scarcity (Shao et al., 2009; Zivcak et al., 2014) that involve different survival strategies (such as stress escape, avoidance or tolerance), adaptive changes and deleterious effects which can all develop even in parallel (Barnabás et al., 2008). They also include the production of many biological macro- and micro-molecules, such as nucleic acids (DNA, RNA, microRNA), proteins, carbohydrates, lipids, hormones, ions, or mineral elements (Shao et al., 2006). These responses to external limiting factors can vary and are genotype- and species-related (Rampino et al., 2006), including the length, intensity and duration of water stress (Araus et al., 2002), plant age and ontogeny (Zhu et al., 2005), light and temperature (Gallé et al., 2009), intensity of previous stresses (Flexas et al., 2009), as well the application of successive drought and recovery cycles (Gallé et al., 2011). Moreover, under natural conditions, plants are often exposed to multiple stress factors that influence photosynthesis and growth (Lu et al., 2003). The combination of drought with other abiotic stress factors, such as intense light, salinity or heat, considerably increases the photoinhibition of photosynthesis (Shao et al., 2006; Yan et al., 2013).
The impact of drought on photosynthesis can basically be divided into two groups: (i) a direct effect, which increases the restriction of the CO2 diffusion pathway via stomata, as intercellular airspaces leading to the mesophyll cells that cause a decline in CO2 availability for Rubisco (Cornic et al., 1989; Chaves, 1991; Flexas et al., 2004a,b, 2007; McDowell, 2011), (ii) an indirect effect, such as alterations in the biochemistry and metabolism of the photosynthetic apparatus, membrane permeability (aquaporins) (Lawlor and Cornic, 2002; Chaves et al., 2009) and the promotion of oxidative stress (Aranda et al., 2012).
Indeed, restricted CO2 diffusion from the atmosphere to the site of carboxylation is the main reason for decreased photosynthesis under water stress conditions caused by both the stomatal and mesophyll limitations (Centritto et al., 2003; Flexas et al., 2004a,b; Grassi and Magnani, 2005; Zivcak et al., 2014). Stomata are the primary component of the CO2 diffusional pathway, which limits water loss. Under prolonged drought, they also limit the CO2 supply inside the leaves (Martorell et al., 2014). In C3 plants, low gs reduces water loss from drying plants to save water via a rapid and effective survival strategy. The stomata response could vary in degree, becoming more pronounced with the increasing severity of a stress (Zivcak et al., 2013). The net CO2 assimilation rate (AN) is usually reduced by water deficit due to not only stomatal closure but also non-stomatal processes (Medrano et al., 2002) such as decreased gm (Flexas et al., 2008). According to Fick's first law of diffusion, AN is determined as follows: AN = gs·(Ca − Ci) = gm·(Ci−Cc), where Ca, Ci, and Cc are the CO2 concentrations in the atmosphere, sub-stomatal cavities and carboxylation site of Rubisco, respectively (Long and Bernacchi, 2003). Previous works usually stated that gm is large and constant (therefore, Ci = Cc). However, at present, there are many lines of evidence suggesting that the CO2 concentration in chloroplasts is significantly lower than in sub-stomatal cavities because of the finite value of gm (von Caemmerer and Evans, 1991; Niinemets et al., 2009). Although gm is rather small, it markedly regulates Cc and hence limits leaf photosynthesis (Di Marco et al., 1990; Harley et al., 1992; Loreto et al., 1992; Warren and Adams, 2006).
The mesophyll conductance indicates the conductance for CO2 flowing from the intercellular air spaces to the site of carboxylation in the chloroplasts of mesophyll cells and includes the quite complicated pathways of the cell wall, plasma membrane, chloroplast envelope, and stromal thylakoids. It involves gas phase resistance among intercellular air spaces and liquid phase resistance from the cell wall to stroma (Evans et al., 2009). Recent studies show a crucial role for gm in the regulation of photosynthesis, and it has already been assumed that gm represents up to 40% of the CO2 diffusional limitations to whole photosynthesis (Warren, 2008).
Currently, there are many studies showing decreased gm during a progressive leaf water deficit. Recent studies (Roupsard et al., 1996; Flexas et al., 2004a, 2006; Delfine et al., 2005; Galmés et al., 2007, 2011; Tomás et al., 2013; Niinemets and Keenan, 2014) clearly confirm that drought in plants may significantly limit gm. Nevertheless, it remains unknown which mechanisms are responsible for the reduction of gm. Any changes in gm during low soil water availability may potentially play an important role in the regulation and control of photosynthesis (Flexas et al., 2014). It is hypothesized that a crop under drought stress should reach low stomatal conductance (gs), which can reduce water loss but consequently maintains a high intensity of carbon fixation. This is only possible when the CO2 concentration in chloroplasts (Cc) remains high as a result of improved gm (Flexas et al., 2012).
The high sensitivity of gm to different environmental factors has already been shown with the reactions occurring in a wide time range, from minutes to hours (Pons and Welchen, 2003; Flexas et al., 2012). Recent reviews have already highlighted the effects of environmental conditions, such as increased and decreased CO2 concentration around leaves (Harley et al., 1992; Centritto et al., 2003), exogenous application of ABA and polyethyleneglycol (Flexas et al., 2006), high altitude (Vitousek et al., 1990), low light (Laisk et al., 2005), low nitrogen availability (Warren and Adams, 2006), low and high temperatures (Bernacchi et al., 2002; Pons and Welchen, 2003; Yamori et al., 2006), or viral infections (Sampol et al., 2003). There is also increasing evidence to suggest a significant role for aquaporins in the control of membrane permeability to CO2, which are also limiting factors of gm in C3 plants (Heckwolf et al., 2011; Sade et al., 2014). In particular, gm is also determined by the variability of leaf structural traits, such as leaf thickness, cell packing, shape, and wall thickness (Tosens et al., 2012; Tomás et al., 2013; Muir et al., 2014).
The decrease in AN as a consequence of water stress is also commonly analyzed in terms of the stomatal and non-stomatal limitations (Grassi and Magnani, 2005). However, the dynamics between the stomatal and non-stomatal limitations during drought remain unclear (Lawlor and Cornic, 2002; Loreto and Centritto, 2008). In previous decades, valuable studies of sufficient quantity accumulated on the effect of drought on gm. Indeed, inter-specific genotypic differences in gm have already been found for several species, e.g., Vitis vinifera (Tomás et al., 2013), Hordeum vulgare (Barbour et al., 2010), Castanea sativa, Solanum lycopersicum (Galmés et al., 2011), Oryza sativa (Gu et al., 2012), and Triticum aestivum (Jahan et al., 2014).
The aim of this work was to perform an eco-physiological analysis of the main diffusional limits to leaf photosynthesis in wheat under a long-term progressive drought by determination of the dynamics and proportion of mesophyll vs. stomatal limitation changes and their sensitivity to water scarcity in four winter wheat genotypes of different geographical proveniences.
Materials and Methods
Biological Material and Cultivation
The outdoor pot experiment was conducted in the experimental cage of the Department of Plant Physiology, Slovak University of Agriculture in Nitra. Seeds of four winter wheat (T. aestivum L.) genotypes (Šamorínska from Slovakia, GK Forrás from Hungary, Pehlivan from Turkey and Piopio-4 from Mexico) were selected on the basis of their (i) geographical origin (European genotypes–Middle to South Europe vs. Latin America), (ii) historical view of wheat breeding (Šamorínska as a landrace vs. GK Forrás, Pehlivan, and Piopio-4 as modern genotypes) and (iii) different mechanism of WUE regulation under drought conditions. They were obtained from the Gene bank in Plant Production Research Institute in Piestany (Slovakia). The seeds were sown in plastic pots (15 l volume) filled with a mixture of horticultural substrate and clay soil in 1:1 ratio. The substrate of pH 7.3 contained 40.08 mg kg−1 Nan, 206.5 mg kg−1 P, 590 mg kg−1 K, and 3.73% of humus. Plants were grown in a natural environmental conditions and were regularly irrigated to maintain the optimum field water capacity during whole experiment. The foliar application of liquid fertilizers with macro- and micro-nutrients was carried out in the early spring time. At the growth stage of inflorescence emergence (BBCH-51, Zadoks et al., 1974), the progressive dehydration of soil and plants in pots was induced by a withholding watering for 21 days. The responses of photosynthesis and water status to the induced water stress were measured simultaneously from gas exchange and leaf RWC data. The leaf hydration range was used for differentiation of the water stress level, and the data were clustered into three groups, e.g., well-watered plants (WW; RWC = 80–100%), mild water stress (MS; RWC = 60–80%) and severe (SS; RWC = 40–60%) water stress. After the dehydration period watering of plants continued optimally. Climatic data (average daily temperature and daily total precipitation; Figure 1) were obtained from the meteorological station of Horticulture and Landscape Engineering Faculty in SUA Nitra, localized in neighborhood of the experimental site.
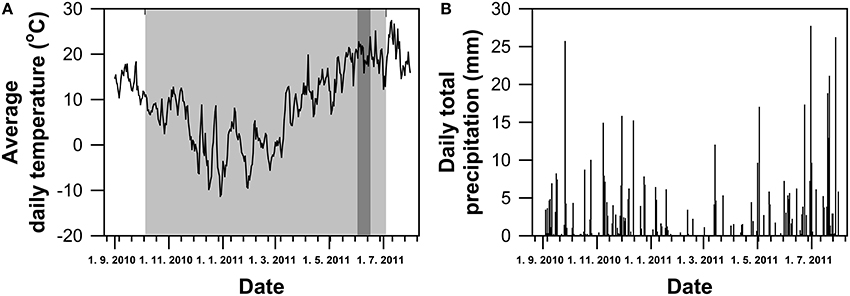
Figure 1. Climatic variables (average daily temperature—(A) and daily total precipitation—(B)) during the grown period of winter wheat. Gray and dark-gray areas represents time of cultivation period and dehydration, respectively.
Gas Exchange and Chlorophyll a Fluorescence Measurements
Gas exchange measurements were made daily on fully expanded flag leaves of control and stressed plants from the beginning of the dehydration process to its terminal phase when the stomata were fully closed.
The AN/Ci response curves of plants from each genotype were measured on a daily basis using the open gas-exchange system Li-6400XT (Li-Cor Inc., Lincoln, Nebraska, USA) with an integrated fluorescence chamber head Li-6400-40 (Li-Cor Inc.). Gas-exchange and chlorophyll a fluorescence parameters were measured in light-adapted leaves at saturation PPFD set up at 1500 μmol m−2 s−1 with 10% blue light to maximize stomatal aperture. Leaf temperature was kept at 21°C and relative air humidity was maintained between 60 and 70% during all measurements. Gas exchange and chlorophyll a fluorescence were first measured after reaching steady-state at 380 μmol CO2 mol−1 air surrounding the leaf (Ca). Subsequently, Ca was decreased stepwise until 50 μmol mol−1 and then increased stepwise until 1500 μmol CO2 mol−1. The number of different Ca values used for the AN/Ci response curves was 12, and the time between the two consecutive measurements at different Ca values was maximal 4 min.
The actual photochemical efficiency of photosystem II (ΦPSII) was assessed following the procedures of Genty et al. (1989) based on the measurements of actual (Fs) and maximal (F) fluorescence during pulse light saturation (intensity 8000 μmol m−2 s−1) and calculated as follows:
The electron transport rate (Jf) was calculated as:
where PPFD is the photosynthetically active photon flux density, α is leaf absorbance (0.85), and β is the partitioning of absorbed quanta between the PSII and PSI. The method of Valentini et al. (1995) was used to determine the product of α.β from the relationship between ΦPSII and ΦCO2 (ΦCO2 = (AN + Rd)/PPFD), where Rd is the daytime respiration rate determined by the Laisk method (Laisk, 1977) (see next section) obtained by varying Ca (11 different values) under non-photorespiratory conditions in an atmosphere containing less than 1% O2, a leaf temperature of 21°C, saturation PPFD (1500 μmol m−2 s−1) and a relative humidity of 75%.
Flow of CO2 out and into the leaf cuvette was determined for the range of Ca values used with photosynthetically inactive leaves (obtained by heating) of each genotype enclosed in the chamber; the correction was used for the calculation of CO2 fluxes (Flexas et al., 2007).
Leaf-intrinsic WUEi was calculated as AN to gs ratio from gas-exchange measurements of Ca at 380 μmol CO2 mol−1 air and saturating light.
Calculation of gm
The mesophyll conductance for CO2 (gm) was estimated from simultaneously measured gas-exchange and chlorophyll a fluorescence parameters of varying Ca according to Harley et al. (1992):
where AN, Jf and Ci were obtained during the dehydration from gas-exchange measurements of Ca at 380 μmol CO2 mol−1 air and saturating light. The chloroplastic CO2 compensation point (Γ*) and daytime respiration rate (Rd) were estimated using the method of Laisk (1977). Several AN/Ci response curves were measured at three different PPFDs (50, 150, and 300 μmol m−2 s−1) and six different Ca levels (from 250 to 50 μmol mol−1) for each genotype in well-watered plants. The intersection point of the linear regression of AN/Ci response curves was used to determine the apparent CO2 compensation point, C (x-axis) and Rd (y-axis). C was used as a proxy for Γ* (Warren and Adams, 2006). The measured data of Rd and Γ* which were used for the calculation of gm are shown in Table 1.
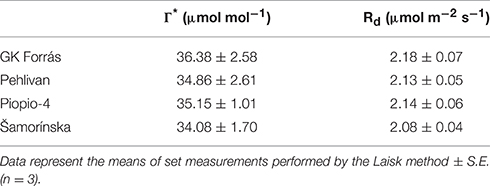
Table 1. The CO2 compensation point in the absence of respiration (Γ*; μmol mol−1) and the mitochondrial respiration rate under light (Rd; μmol m−2 s−1) as measured in four wheat genotypes under well-watered conditions.
Calculation of Vcmax
The maximal in vivo carboxylation activity of Rubisco (Vcmax) was calculated from the gas exchange measurement by the data fitting procedure of the initial slope of the AN/Ci curve (Ci < 300 μmol mol−1):
where A is the net assimilation rate limited by Rubisco activity, and Kc and Ko are the Michaelis-Menten constants of Rubisco activity for RuBP carboxylation and oxygenation, respectively. Kc and Ko are assumed to be 404.9 μmol mol−1 and 278.4 mmol mol−1 at 25°C, respectively, according to Bernacchi et al. (2001). Oxygen concentration in chloroplasts (O) was assumed to be 210 mmol mol−1.
Estimation of Relative Limitation to Photosynthesis
The limitation of photosynthesis based on gs and gm was estimated as potential rate of photosynthesis assuming these conductance values were infinite or measured, respectively (Farquhar and Sharkey, 1982). AN/Ci curves were used to separate and estimate the stomatal and non-stomatal limitations to photosynthesis. To assess an effect of dehydration on CO2 assimilation, the photosynthetic limitations were partitioned into the components related to stomatal and mesophyll conductance according to Warren et al. (2003) and calculated as follows:
where LS and LM are the relative stomatal and mesophyll limitation of AN, respectively, ACa is the light-saturated rate of photosynthesis at Ca = 380 μmol mol−1 (gs and gm as measured), ACi is the light-saturated rate of photosynthesis at Ci = 380 μmol mol−1 (assuming gs was infinite and gm was measured), and ACc is the light-saturated rate of photosynthesis at Cc = Ci (assuming gm was infinite and gs was measured).
Relative Water Content
The leaf relative water content (RWC) was determined as:
The leaf disc was cut out from the central part of a measured leaf. Fresh weight (FW) was determined immediately after the gas exchange measurement. Turgid weight (TW) was obtained after 12 h of hydration, when a leaf disc was kept in distilled water at 4°C in the dark. Dry weight (DW) was measured after drying the leaf disc at 80°C for 24 h.
Statistical Analyses
The experiment with wheat plants in pots was established by block method with a completely randomized design of experimental plots. All analyses were performed using the Statistica v. 10 software (StatSoft Inc., Tulsa, Oklahoma, USA) and the graphics software SigmaPlot version 11.0 (Systat Software Inc., San Jose, California, USA). Analysis of variance was performed between the different levels of drought (well-watered, mild and severe water stress) at a significance level of 0.05, and Duncan's post hoc test was used. The variability between investigated genotypes was tested by the HSD test.
Results
Climatic conditions at the experimental site are shown in Figure 1. Average daily temperature during the growing season (October 5, 2010 to July 4, 2011) was 7.4°C with the sum of precipitation of 373.9 mm. The sum of active daily temperatures (above 10°C) per growing season was 1658°C. The average daily temperature during the drought treatment was 20.03°C.
Significant differences among the investigated wheat genotypes grown in WW conditions were found for AN, gs, gm, and Vcmax. The AN and gs varied from 26.39 to 28.64 μmol m−2 s−1 and 0.50 to 0.43 mol m−2 s−1, respectively. Differences among wheat genotypes for WUEi were non-significant (p > 0.05) and varied from 56.87 to 64.52 μmol CO2 mol−1 H2O. Genotype Pehlivan reached the highest value for these parameters (Table 2). Genotypic variation in gs (c.v. 12%) explained 7% of the observed variability in AN under WW conditions (Table 3). Mesophyll conductance (gm) in WW plants varied nearly 3-fold among all genotypes, from 0.24 to 0.73 mol m−2 s−1 (p < 0.001). The highest value for gm was observed in the genotype Pehlivan.
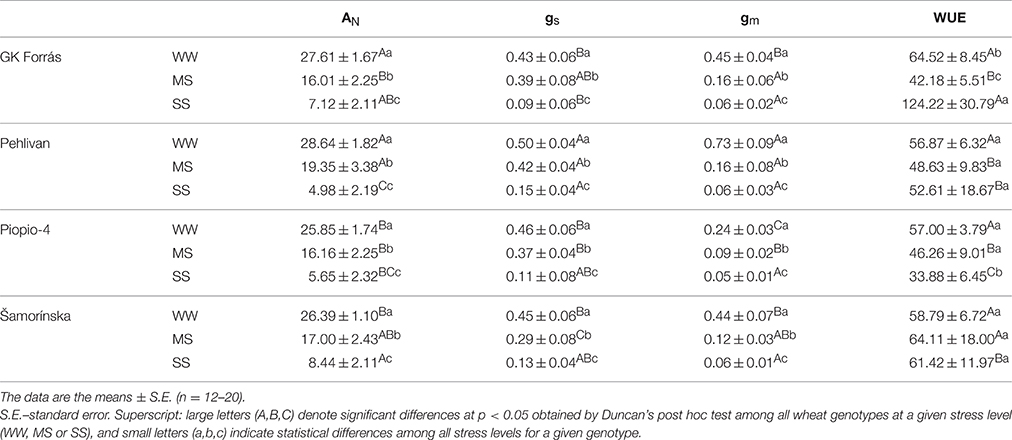
Table 2. The net CO2 assimilation rate (AN; μmol m−2 s−1), stomatal conductance to H2O (gs; mol H2O m−2 s−1), mesophyll conductance to CO2 (gm; mol CO2 m−2 s−1) and leaf-intrinsic water use efficiency (WUEi calculated as AN/gs ratio; μmol CO2 mol−1 H2O) in flag leaves of four wheat genotypes under well-watered (WW; RWC = 80–100%), mild stressed (MS; RWC = 60–80%) and severe stressed (SS; RWC = 40–60%) conditions.
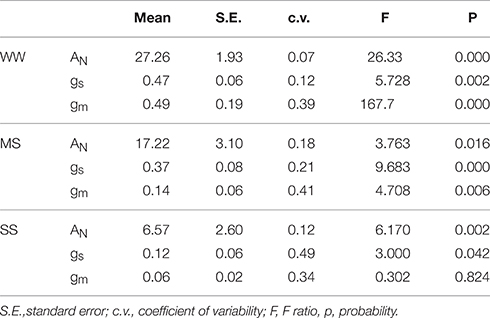
Table 3. Genotypic variability of the net CO2 assimilation rate (AN; μmol m−2 s−1), stomatal conductance to H2O (gs; mol H2O m−2 s−1), and mesophyll conductance to CO2 (gm; mol CO2 m−2 s−1) in four wheat genotypes under well-watered (RWC = 80–100%), mild stress (RWC = 60–80%), and severe stress (RWC = 40–60%) conditions.
Significant reductions in AN, gs, and gm were observed under progressive dehydration from WW conditions (Figure 2, Tables 2, 3). Under the MS conditions, significant genotypic differences were found in AN and gs, which varied from 16.01 to 19.35 μmol m−2 s−1 and 0.29 to 0.42 mol m−2 s−1, respectively. Thus, the average 1.5-fold reduction of AN was accompanied by an almost 20% reduction of gs and a 3.5-fold reduction of gm. The highest stomatal sensitivity to the decline in RWC was observed in genotype Šamorínska, while the highest sensitivity of gm to RWC was found in genotype Pehlivan. Under severe water stress (SS) conditions, gs declined below 0.15 mol m−2 s−1 in all genotypes, with the most pronounced reduction in GK Forrás. However, we should be noted that in genotypes Piopio-4 and Šamorínska originating from Mexico and Slovakia (Šamorínska is a Slovakian landrace), respectively, the dehydration cycle was faster (11 days), causing the gs to drop below 0.08 mol m−2 s−1, while in genotypes Pehlivan and GK Forrás from Turkey and Hungary, similar gs values (0.09 and 0.15 mol m−2 s−1) were reached after 15 and 16 days of dehydration, respectively. The reduction of leaf RWC resulted in the decline of gm (0.05–0.06 mol m−2 s−1) with non-significant (p > 0.05) genotypic differences. The gs and gm reductions resulted in the reduction of AN (Table 2). Then, the reduction of gs relative to AN in genotype GK Forrás under drought condition significantly (p < 0.001) increased WUEi. Finally, under SS conditions, the genotypic variation in gs (c.v. 49%) explained 12% of the observed variability in AN (Table 3).
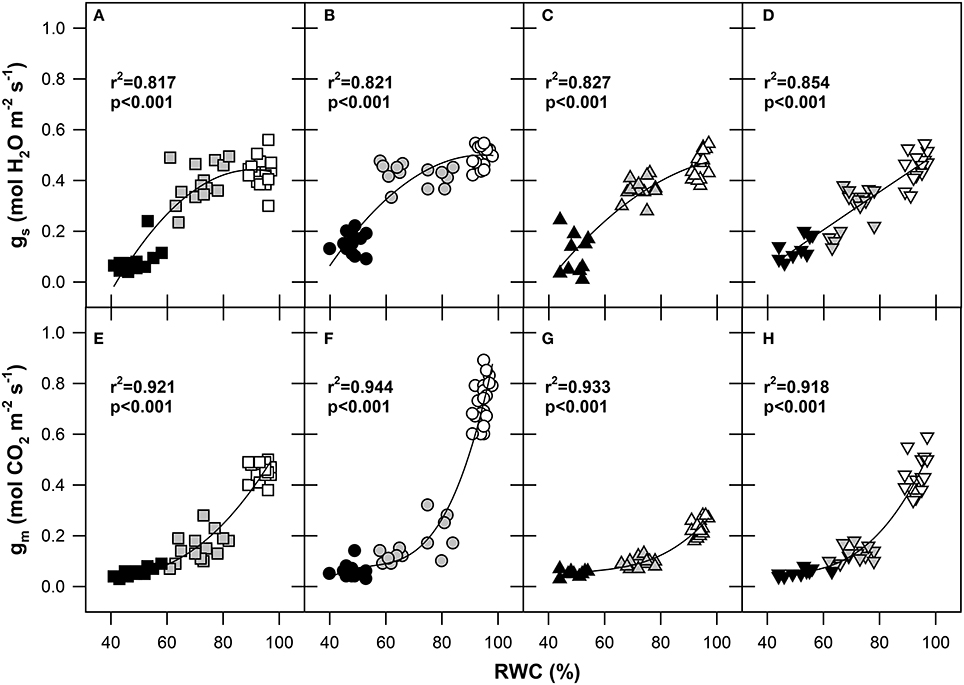
Figure 2. Responses of stomatal conductance to H2O (gs; mol H2O m−2 s−1) and mesophyll conductance to CO2 (gm; mol CO2 m−2 s−1) to the relative water content (RWC; %) of flag leaves in four wheat genotypes (A, E) GK Forrás, (B, F) Pehlivan, (C, G) Piopio-4 and (D, H) Šamorínska. The points represent individual measurements of leaves. Symbols: square-GK Forrás, circle-Pehlivan, up-triangle-Pio, down-triangle–Šamorínska; empty symbols–well-watered (RWC 80–100%) plants, gray symbols-mild stress (RWC 60–80%) and black symbols-severe stress (RWC 40–60%). The coefficients of determination (r2) and significance level (p) as well as the lines of polynomial quadratic (A–D) and polynomial cubic (E–H) regressions are shown.
There was a clear polynomial decline in gs induced by stomatal closure in the plant response to progressive drought, showing the same trends for genotypes GK Forrás, Pehlivan, and Piopio-4 (Figures 2A–C), with the exception of genotype Šamorínska (Figure 2D), which showed almost linear decline of gs. This result indicates a high stomatal sensitivity of landrace genotype to water stress, confirming that stomata were completely closed after 11 days of dehydration.
As shown in Figure 3, the AN was positively correlated with gm under progressive dehydration in all genotypes (r2 from 0.890 for Pehlivan to 0.924 for Šamorínska; p < 0.001). A significant decline in AN in response to reduced gm was observed under the transition from WW to MS conditions. Under SS conditions, a strong reduction of gm (below 0.15 mol m−2 s−1) resulted in a progressive decline of AN; however, this was still above the CO2 compensation point in all genotypes. The largest slope of the AN/gm relationship was observed in the Piopio-4 genotype, where we conclude that the drought stress had a greater impact on gm compared to AN.
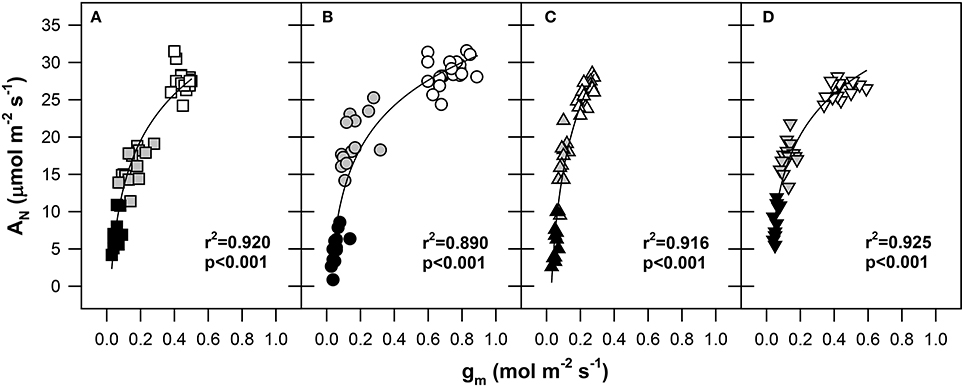
Figure 3. The net CO2 assimilation rate (AN, μmol CO2 m−2 s−1) response to mesophyll conductance (gm, mol CO2 m−2 s−1) in (A) GK Forrás, (B) Pehlivan, (C) Piopio-4 and (D) Šamorínska genotypes under progressive drought conditions. The points represent individual measurements of leaves. The symbols are the same as in Figure 2. The coefficients of determination (r2) and significance level (p) as well as the line of logarithmic regressions are shown in the plot.
Analysis of the in vivo maximal carboxylation activity of Rubisco (Vcmax) revealed the genotypic variability (p < 0.05) only under well-watered conditions (Figure 4), with the changes ranging from 88.14 ± 6.3 to 108.44 ± 8.2 μmol m−2 s−1 for genotypes Šamorínska and Pehlivan, respectively. Water stress (MS and SS) significantly (p < 0.01) reduced Vcmax, but without any genotypic difference. The mean level of Vcmax was 74.8 ± 5.4 and 39.12 ± 1.2 μmol m−2 s−1 both in MS and SS, which constituted ~0.5-fold and 2.5-fold decline for MS and SS, respectively.
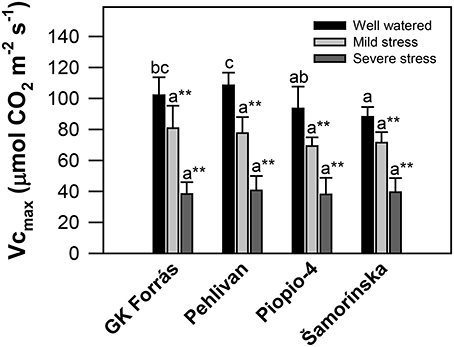
Figure 4. The maximum rate of carboxylation of Rubisco (Vcmax; μmol CO2 m−2 s−1) calculated from the gas-exchange data measured in the leaves of four wheat genotypes at different levels of drought stress. Vertical bars are the means of 9–20 individual measurements per treatment ± S.E. Different letters indicate significant differences among genotypes (p < 0.05) based on Duncan's post hoc test at one stress level; **Indicates significant differences among stress treatments (p < 0.01) based on the HSD test in one genotype.
Analysis of the fast AN–Ci response curve showed that the gm calculated via the method of Harley et al. (1992) was not constant along the range of Ci values employed in this study (Figure 5). We observed the obviously known three-phase course of gm changes to varied Ci values. A strong sensitivity of gm at low Ci concentrations was observed in the first part of the response curve (Ci from ~80 to 200 μmol mol−1 air). After reaching an inflection peak of gm at Ci concentrations from 200 to 400 μmol mol−1 air, the gm values declined exponentially under the value of 0.1 mol m−2 s−1 at high Ci. The maximal sensitivity of gm to increased Ci was observed in Pehlivan (Figure 5B) with a 16-fold reduction of gm observed until the steady-state level was reached. The weak sensitivity of gm to increased Ci (only ~4-fold decline) was observed in the Piopio-4 genotype (Figure 5C). The highest genotypic differences in the sensitivity of gm to Ci variations were observed at low Ci concentrations (GK Forrás and Pehlivan with relatively lower gm and Piopio-4 and Šamorínska with relatively higher gm). Water stress reduced the sensitivity of gm to Ci changes in all of the investigated genotypes. During the transition from the mild to severe water stress, the mechanism responsible for the gm reaction was clearly inhibited, and gm did not react as fast as in the case of well-watered plants. The gm was negatively affected under SS conditions in all genotypes when the response to altered Ci was inhibited. Our results support the suggestions of others that mild to severe drought strongly influences the mechanism of gm regulation (Figure 5).
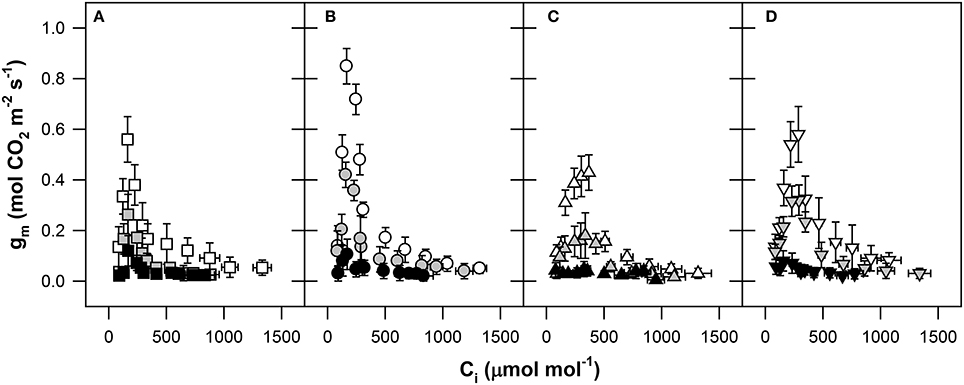
Figure 5. The response of mesophyll conductance (gm, mol CO2 m−2 s−1) to rapid changes in the CO2 concentration within intercellular spaces (Ci, μmol CO2 mol−1 air) in (A) GK Forrás, (B) Pehlivan, (C) Piopio-4, and (D) Šamorínska. The points represent means from 9 to 20 individual measurements per treatment ± S.E. The symbols correspond to those presented in Figure 2.
As shown in Figure 6, a close relationship between gm and gs was observed in all genotypes and stress levels (r2 = 0.77; p < 0.001). During the transition state from WW to MS conditions, the 1.5-fold reduction of gs was accompanied by a 3-fold decline of gm. A further increase in water stress up to SS conditions resulted in progressive stomatal closure and a reduction of gs accompanied by only small changes in gm. However, the transition from WW to MS affected both gs and gm in approximately the same measure. Thus, the final gm/gs relationship was linear. The highest slope of gm/gs was identified for genotype Pehlivan, while the lowest was identified for Piopio-4.
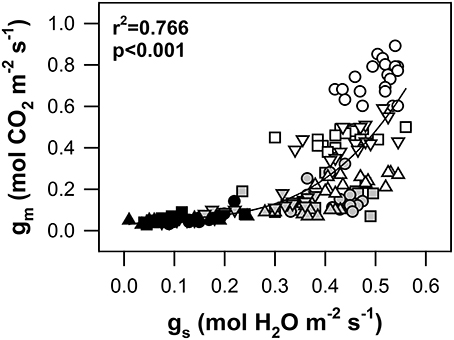
Figure 6. The relationship between the stomatal conductance to H2O (gs, mol H2O m−2 s−1) and mesophyll conductance to CO2 (gm, mol CO2 m−2 s−1) in the leaves of four wheat genotypes under progressive drought. The symbols are the same as in Figure 2. Data were fitted by a non-linear regression (full line). The equation is gm = 0.426 gs − 2.129 g 6.189 g, and the coefficient of determination (r2) and significance (p) are shown (n = 161) in the plot.
Based on the analyses of the AN/Ci response curves measured on a daily basis during the experiment, the stomatal and mesophyll limitation ratio was calculated (Figure 7). After the determination of both limitations in all genotypes, genotypic differences in the limitations were evaluated. The observed differences could tell us more about drought response reactions and could also help determine which limitation is more crucial for the regulation of photosynthesis during drought.
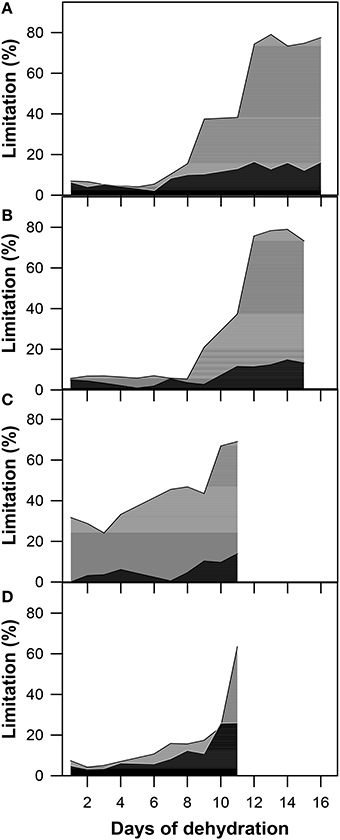
Figure 7. The stomatal (LS; black) and mesophyll (LM; gray) limitations to the AN calculated as the % of the maximum (well-watered) values in (A) GK Forrás, (B) Pehlivan, (C) Piopio-4, and (D) Šamorínska genotypes under progressive drought. Each value represents the mean of four replicates.
From the first day of the experiment, we assessed the initial values of stomatal (LS) and mesophyll (LM) limitations as a percentage (Figure 7). As drought progressed and leaf water deficit increased, both LS and LM increased simultaneously, but the dynamics of the increase became uneven. LS began to increase to a less extent than LM. The maximal value of LS (22.53%) was reached in stressed plants of the old Slovak genotype Šamorínska. However, this is not a crucial value that limits leaf photosynthesis. Therefore, we suggest that LS did not play as important a role in comparison with LM in dehydrated plants of all selected genotypes. LM predominated in three genotypes (Šamorínska, GK Forrás and Piopio-4). Although the LS of Pehlivan was higher than LM in the first period of dehydration, it changed after LM dominated over LS. In genotype Piopio-4, LM was mostly disabled by drought in comparison with other genotypes. It obtained very high initial values (31.91%) and increased even further with a culmination at 69.2% as the drought progressed. Additionally, a great impact of water deficit caused a significant increase in LM and was found in dehydrated plants of Pehlivan (76.2%) and GK Forrás (77.6%).
Discussion
Soil water scarcity is the main limiting factor for crop growth and yield worldwide. Despite the increased knowledge over the past decade on the effects of water stress on photosynthesis, there is still a controversial debate whether water stress limits AN primarily by stomata closure (stomata limitation) or mesophyll limitation (diffusional and metabolic). A general response of plant tissues to soil water deficit is the decline of relative water content (RWC). This depends on the strength and duration of drought stress (Chaves et al., 2009). The withholding of water resulted in the reduction of stomatal conductance (gs) as a consequence of stomatal closure (Table 2; Figure 2) with significant genotypic differences (Table 3). The higher stomata sensitivity to RWC decline found in the genotype Šamorínska is the result of rapid water loss from leaf tissues (Figure 2D). As observed from our experimental data, modern genotypes reacted to drought by a slow reduction of gs at the initial phase of dehydration, probably due to better osmotic adjustment and/or a deeper and more efficient root system (Wasson et al., 2012).
The reduction in AN resulting from decreased RWC was significantly correlated with a decline in gs. This response is similar to those observed in many studies, and it is thought to be the general acclimation response of plants to drought (Cornic et al., 1989; Chaves, 1991; Cornic, 2000; Flexas et al., 2006). Under the gradual dehydration induced by withholding watering in plants, a highly significant relationship (r2 = 0.93; data not shown) between the RWC decline and the reduction in AN was observed. Flexas et al. (2006) summarized their own results and compared them with others to reach a compromise in order to determine what limits AN more, stomata closure or metabolic impairments in the mesophyll. They noted that the reduction of CO2 supply from the atmosphere to chloroplasts was the main factor that decreased AN under drought conditions. However, metabolic impairments occurred as well, but only during stronger water stress when gs dropped below 0.10 mol H2O m−2 s−1.
In our study with well-watered wheat plants, the observed gm corresponded to the gm level for wheat as found in many published works (Tazoe et al., 2009, 2011; Jahan et al., 2014; Sun et al., 2015). Interestingly, a wide interval and significant genotypic differences in gm (from 0.24 to 0.73 mol m−2 s−1) (Tables 2, 3) may be the result of both the differences in Rubisco activity and the anatomical properties of leaves, respectively (Evans et al., 1994, 2009; Medrano et al., 2002; Parry et al., 2002; Flexas et al., 2006; Niinemets et al., 2009; Tomás et al., 2013; Muir et al., 2014). The role of aquaporins in the transport of CO2 and thus the regulation of gm are also essential (Hanba et al., 2004). Inter-specific variations in gm were also previously reported in a number of publications (Ethier and Livingston, 2004; Niinemets et al., 2009; Tomás et al., 2013; Niinemets and Keenan, 2014).
Based on the data analyses, a strong relationship was observed in our measurements between AN and gm (Figure 3). The gm decreased simultaneously as AN declined, which was caused by enhanced water scarcity. This trend was found for each of the studied wheat genotypes. This observed strong correlation demonstrates a well-known fact about the substantial regulation of gm that is directly connected to AN and thus represents the main factor underlying diffusive limitation for CO2 from the internal sub-stomatal cavities to the site of carboxylation (Tezara et al., 1999). Ultimately, due to this significant relationship, we could also consider gm as the main factor that limits photosynthesis (Lawlor and Cornic, 2002) and plays a crucial role in the entire metabolism within the leaf mesophyll (Flexas et al., 2012).
Previously, one group of researchers argued that the decline in AN occurs as a direct consequence of stomata closure, which restricts further CO2 diffusion from the intercellular spaces to the sites of carboxylation (Sharkey, 1990; Chaves, 1991; Cornic, 2000). On the other hand, Tezara et al. (1999) suggested that the decline of AN is due to the impairment of ATP and RuBP synthesis and low ATP content, rather than stomata limitation. Another factor could be any of the processes of the Calvin cycle, although it is still not clear which of these might be involved. Moreover, drought is able to damage and influence processes involved in RuBP regeneration, e.g., activities of key enzymes of the Calvin cycle, such as fructose-1,6-bisphosphate phosphatase, NADP:glyceraldehyde-3-phosphate dehydrogenase, ribulose-5-phospho kinase, or 3-phosphoglycerate kinase (Flexas et al., 2004a).
It has been established that gm is a finite variable (Niinemets et al., 2009). By simultaneously measuring gas exchange and chlorophyll a fluorescence, we exposed a substantial inhibition of gm during the development of water stress. It has been shown that gm is extremely sensitive to drought; photosynthesis in water-stressed conditions is considerably reduced (Grassi and Magnani, 2005; Flexas et al., 2006, 2007). In accordance with this, our results confirmed the differences in the kinetics of mesophyll limitation during photosynthesis (Figure 3). The genotypes Pehlivan and Piopio-4 differed the most in this regard (Figures 3B,C).
It is also well-known that gm controls the metabolic and anatomical properties of leaves during photosynthesis. Both the amount and activity of Rubisco are crucial in the control of gm (Niinemets et al., 2009). Therefore, we would expect a large inhibition of the maximal in vivo carboxylation activity of Rubisco (Vcmax) due to prolonged dehydration, which has already been established. During mild and severe stress conditions, drought induced a significant (2.5-fold) decline in Vcmax in all genotypes (Figure 4). However, the Vcmax decline should be more pronounced than was found in our experiment. Flexas et al. (2006) achieved 94% decrease in the Vcmax of Nicotiana tabacum plants resulting from inhibition of Rubisco activity as was also confirmed by other works (Medrano et al., 1997; Parry et al., 2002). Lawlor and Tezara (2009) studied the problem of Rubisco inhibition under drought in more detail and concluded that a key for the response was a decline in the Rubisco activase enzyme activity. Similarly, Lawlor and Cornic (2002) also reported that decreased Rubisco activase activity resulted from progressive water stress.
During our experiment, the dependence between gm and Ci was clearly demonstrated (Figure 5). Plants also differed in their gm sensitivity to changing Ci. Previously, a rapid response of gm as found in our study has also been reported by Centritto et al. (2003), Flexas et al. (2007, 2014), Bunce et al. (Bunce, 2009) and Tazoe et al. (2011). However, such a deep analysis has not been presented for wheat. But, as seen from the work of Flexas et al. (2007), the relationship was established for many different plant species, such as Arabidopsis thaliana, Limonium gibertii, N. tabacum, Vitis berlandieri × Vitis rupestris, Cucumis sativus, and Olea europaea var. europaea. These studies show that gm rapidly responds to changing Ci ranging from 50 to 1200 μmol mol−1 air. At high CO2 concentrations in sub-stomatal cavities where CO2 is limited by insufficient available energy, gm sharply decreases.
Irrespectively to current knowledge about the function and regulation of gm, the mechanism leading to the photosynthetic response to varying Ci remains unclear. Even less is known about the intra-species variations in gm at changing Ci. It has been assumed that the genotypic divergence could be the result of different structural characteristics and features of leaves as well as the activity of membrane aquaporins (von Caemmerer and Evans, 1991; Kjellbom et al., 1999). Another possible mechanism clearly affecting gm, but not linked to the function of aquaporins, is chloroplast swelling and movement (Flexas et al., 2007).
Co-regulation between gm and gs is currently debated by many scientists. This is still a complicated question because CO2 diffusion from the ambient air directly into the chloroplasts is defined by gs and gm together, which could vary either over the long-term periods of leaf morphological changes or over short-term changes in chloroplast membrane permeability (Evans et al., 2009; Tosens et al., 2012). However, a verdict on the co-regulation of both remains to be presented. Centritto et al. (2003) and Warren (2008) argued that a linear relationship between gm and gs is not ubiquitous but rather differs among species and levels of water stress. On the other hand, studies by Loreto et al. (1992), Flexas et al. (2002, 2008), Ethier et al. (2006), and Perez-Martin et al. (2009) show a strong co-regulation between gm and gs. The results from our experiment confirmed a co-regulation of both limiting components of the CO2 diffusion pathway (Figure 6). An interesting finding was additionally observed if plant sensitivity studied under drought. The current works highlighted that both gm and gs operate sequentially rather than in parallel, and that the mechanisms of their co-regulation in wheat are still not fully clear. However, the responses of gm and gs to environmental stimuli have recently been studied intensively (Barbour et al., 2010; Easlon et al., 2014).
The gm in our experiment responded more rapidly than gs, as also suggested by Flexas et al. (2008), Bunce et al. (Bunce, 2009), and Keenan et al. (2010), and their mutual dependence was found to be statistically significant (r2 = 0.77). Based on our results, we support the suggestions of Flexas et al. (2006, 2007) and Warren et al. (Warren, 2008) in that these two parameters of the CO2 diffusion pathway in photosynthesizing leaves are dependent on each other. This work has also shown that the relationship is highly variable in many species and could be affected by a variety of environmental factors.
Although the increase in stomatal (LS) and mesophyll (LM) limitations to photosynthesis as a result of water scarcity is quite well-documented, processes linked to these phenomena are still a matter of debate (Flexas and Medrano, 2002). Restricted CO2 diffusion from the surrounding atmosphere to chloroplasts is a common response to water deficit and is caused by limiting factors to photosynthesis even under mild stress conditions (Roupsard et al., 1996; Grassi and Magnani, 2005; Chaves et al., 2009). To study the impact of drought and to demonstrate which limits of photosynthesis dominate, AN–Ci response curve analyses are often used (Ni and Pallardy, 2009).
In our analysis of LS and LM under progressive drought stress (Figure 7), genotype differences in these parameters were observed. A variety of differences could be dependent on both the intensity and duration of stress, as well as different abilities to respond to water shortage (Grassi and Magnani, 2005). Under the initial water stress, LS dominated over LM in the Pehlivan genotype (Figure 6). Furthermore, as the water stress developed, LM increased and became crucial. The reason was simply the decline of gm, which was caused by the reduced CO2 concentration within chloroplasts. However, LS has not yet been distinguished at this point in comparison with LM. This was caused by only a slight change in the intercellular CO2 concentration (Ci), as also found by Lawlor and Cornic (2002). Of course, LS increased as well. However, its development was less sufficient compared to LM. The same result for the function of LM was reported in the studies by Galmés et al. (2007) and Tosens et al. (2012).
Our photosynthesis limitation analysis showed that the dynamics of the changes in LS and LM were different in genotypes GK Forrás, Piopi-4 and Šamorínska (Figures 7A,C,D). Since the beginning of dehydration, LM and LS have increased concurrently, as was also observed by Martin-Ruiz and Torres (1992). However, LM began to dominate immediately from the first day of dehydration, as was also observed in the work of Delfine et al. (2001). They argued that the high values of LM indicate the reduction of gm and that the increase in LM is responsible for the impairment of plant metabolism. LM values above 80% were also demonstrated by Gallé et al. (2009) in tobacco plants. Other studies (Escalona et al., 1999) observed significant increases in LS and LM at the same time of a stress. Finally, we obtained similar results as documented in the studies of Flexas et al. (2014), Limousin et al. (2010), Misson et al. (2010), and StPaul et al. (2012), which stated that LS is a more important factor during early drought events; however, under severe water stress, LM dominates over LS and primarily limits wheat photosynthesis.
Conclusions
The present results show a significant inter-genotypic variability in wheat photosynthetic responses to a long-term progressive drought, as studied in four selected wheat genotypes of different geographical origins and breeding chronology. Our study demonstrated the effect of low water availability in plants on gm inhibition. Drought clearly reduced gm during long-term progressive dehydration in all wheat genotypes. The results show that gm is co-regulated with gs with their strong effect on AN regulation. Interestingly, gm is a genotypic variable not only for the conditions of drought but also for well-watered plant conditions. Therefore, we offer reliable evidence of a crucial role for gm in the regulation of CO2 assimilation under both well-watered and drought conditions. We also demonstrated a rapid response of gm to short-term Ci changes with significant genotypic variability under WW conditions. However, this response is significantly reduced without any genotypic effect during prolonged drought. For future research, we suggest the study of leaf anatomical traits linked to the limitations of photosynthesis together with an evaluation of plant photosynthetic parameters. It has been hypothetized, and in some individual works already demonstrated, that the differences in leaf anatomy may have a rather significant influence on the CO2 diffusion within the leaf mesophyll and on the whole leaf photosynthetic performance. In summary, the present results with wheat are statistically remarkable, and they contribute to the general knowledge of the regulation of leaf photosynthesis under periods of water scarcity by the mesophyll and stomata.
Author Contributions
HS, KO, MB designed the experiment and revised the paper; MK, MZ performed the experiment; KO, PS, MZ, MK analyzed the data and finished the original paper.
Conflict of Interest Statement
The authors declare that the research was conducted in the absence of any commercial or financial relationships that could be construed as a potential conflict of interest.
The reviewer JL declared a shared affiliation, though no other collaboration, with several of the authors HS, MB to the handling Editor, who ensured that the process nevertheless met the standards of a fair and objective review.
Acknowledgments
The authors are thankful to Mgr. Marian Gabris, Ph.D. for his great technical support during the experimental work. The work was financially supported by the National Project of the Agency for Research and Development under the Ministry of Education in Slovakia (project No. APVV-15-0721) and the One Hundred Talent Plan of Foreign Experts of Jiangsu Province (JSB2015005).
Abbreviations
Γ*, chloroplastic CO2 compensation point; ΦPSII, actual photochemical efficiency of photosystem II; AN, net CO2 assimilation rate; Ca, ambient CO2 concentration; Cc, CO2 concentration at the carboxylation site of Rubisco; Ci, CO2 concentration in sub-stomatal cavities; gm, mesophyll conductance; gs, stomatal conductance; Jf, electron transport rate; MS, mild water stress; PPFD, photosynthetically active photon flux density; Rd, day respiration rate; RWC, relative water content; SS, severe water stress; Vcmax, maximal in vivo carboxylation activity of Rubisco; WUEi, intrinsic water use efficiency; WW, well-watered conditions; c.v., coefficient of variability.
References
Aranda, I., Gil-Pelegrín, E., Gascó, A., Guevara, M. A., Cano, J. F., Miguel, M., et al. (2012). “Drought response in forest trees: from the species to the gene,” in Plant Responses to Drought Stress, ed R. Aroca (Heidelberg: Springer Berlin Heidelberg), 293–333.
Araus, J. L., Slafer, G. A., and Reynolds, M. P. (2002). Plant breeding and drought in C3 cereals: what should we breed for? Ann. Bot. 89, 925–940. doi: 10.1093/aob/mcf049
Barbour, M. M., Warren, C. R., Farquhar, G. D., Forrester, G., and Brown, H. (2010). Variability in mesophyll conductance between barley genotypes, and effects on transpiration efficiency and carbon isotope discrimination. Plant Cell Environ. 33, 1176–1185. doi: 10.1111/j.1365-3040.2010.02138.x
Barnabás, B., Jäger, K., and Feher, A. (2008). The effect of drought and heat stress on reproductive processes in cereals. Plant Cell Environ. 31, 11–38. doi: 10.1111/j.1365-3040.2007.01727.x
Bernacchi, C. J., Portis, A. R., von Caemmerer, S., and Long, S. P. (2002). Temperature response of mesophyll conductance. Implications for the determination of Rubisco enzyme kinetics and for limitation to photosynthesis in vivo. Plant Physiol. 130, 1992–1998. doi: 10.1104/pp.008250
Bernacchi, C. J., Singsaas, E. L., Pimentel, C., Portis, A. R. Jr., and Long, S. P. (2001). Improved temperature response functions for models of Rubisco-limited photosynthesis. Plant Cell Environ. 24, 253–259. doi: 10.1111/j.1365-3040.2001.00668.x
Bunce, J. (2009). Use of the response of photosynthesis to oxygen to estimate mesophyll conductance to carbon dioxide in water-stressed soybean leaves. Plant Cell Environ. 32, 875–881. doi: 10.1111/j.1365-3040.2009.01966.x
Cano, F. J., López, R., and Warren, C. R. (2014). Implications of the mesophyll conductance to CO2 for photosynthesis and water-use efficiency during long-term water stress and recovery in two contrasting Eucalyptus species. Plant Cell Environ. 37, 2470–2490. doi: 10.1111/pce.12325
Centritto, M., Loreto, F., and Chartzoulakis, K. (2003). The use of low CO2 to estimate diffusional and non-diffusional limitations of photosynthetic capacity of salt-stressed olive saplings. Plant Cell Environ. 26, 585–594. doi: 10.1046/j.1365-3040.2003.00993.x
Chaves, M. M. (1991). Effects of water deficit on carbon assimilation. J. Exp. Bot. 42, 1–16. doi: 10.1093/jxb/42.1.1
Chaves, M. M., Flexas, J., and Pinheiro, C. (2009). Photosynthesis under drought and salt stress: regulation mechanism from whole plant to cell. Ann. Bot. 103, 551–560. doi: 10.1093/aob/mcn125
Chaves, M. M., Maroco, J. P., and Pereira, J. S. (2003). Understanding plant response to drought – from genes to whole plant. Funct. Plant Biol. 143, 122–133. doi: 10.1071/fp02076
Condon, A. G., Richards, R. A., Rebetzke, G. J., and Farquhar, G. D. (2004). Breeding for high water-use efficiency. J. Exp. Bot. 55, 2447–2460. doi: 10.1093/jxb/erh277
Cornic, G. (2000). Drought stress inhibits photosynthesis by decreasing stomatal aperture – not by affecting ATP synthesis. Trends Plant Sci. 5, 187–188. doi: 10.1016/S1360-1385(00)01625-3
Cornic, G., Le Gouallec, J. L., Briantais, J. M., and Hodges, M. (1989). Effect of dehydration and high light on photosynthesis of two C3 plants (Phaseolus vulgaris L. and Elastotema repens (Lour.) (Hall f.). Planta 177, 84–90. doi: 10.1007/BF00392157
Delfine, S., Loreto, F., and Alvino, A. (2001). Drought-stress effects on physiology, growth and biomass production of rained and irrigated bell pepper plants in the Mediterranean region. J. Am. Soc. Hort. Sci. 126, 297–304.
Delfine, S., Loreto, F., Pinelli, P., Tognetti, R., and Alvino, A. (2005). Isoprenoids content and photosynthetic limitations in rosemary and spearmint plants under drought water stress. Agric. Ecosystem Environ. 106, 243–252. doi: 10.1016/j.agee.2004.10.012
Di Marco, G., Manes, F., Tricoli, D., and Vitale, E. (1990). Fluorescence parameters measured concurrently with net photosynthesis to investigate chloroplastic CO2 concentration in leaves of Quercus ilex L. J. Plant Physiol. 136, 538–543. doi: 10.1016/S0176-1617(11)80210-5
Easlon, H. M., Nemali, K. S., Richards, J. H., Hanson, D. T., Juenger, T. E., and McKay, J. K. (2014). The physiological basis for genetic variation in water use efficiency and carbon isotope composition in Arabidopsis thaliana. Photosyn. Res. 119, 119–129. doi: 10.1007/s11120-013-9891-5
Escalona, J. M., Flexas, J., and Medrano, H. (1999). Stomatal and nonstomatal limitations of photosynthesis under water stress in field-grown grapevines. Aust. J. Plant Physiol. 26, 421–433. doi: 10.1071/PP99019
Ethier, G. J., and Livingston, N. J. (2004). On the need to incorporate sensitivity to CO2 transfer conductance into Farquhar – von Caemmerer – Berry leaf photosynthesis model. Plant Cell Environ. 27, 137–153. doi: 10.1111/j.1365-3040.2004.01140.x
Ethier, G. J., Livingston, N. J., Harrison, D. L., Black, T. A., and Moran, J. A. (2006). Low stomatal and internal conductance to CO2 versus Rubisco deactivation as determinants of the photosynthetic decline of ageing evergreen leaves. Plant Cell Environ. 29, 2168–2184. doi: 10.1111/j.1365-3040.2006.01590.x
Evans, J. R., Kaldenhoff, R., Genty, B., and Terashima, I. (2009). Resistances along the CO2 diffusion pathway inside leaves. J. Exp. Bot. 60, 2235–2248. doi: 10.1093/jxb/erp117
Evans, J. R., von Caemmerer, S., Setchell, B. A., and Hudson, G. S. (1994). The relationship between CO2 transfer conductance and leaf anatomy in transgenic tobacco with a reduced content of Rubisco. Aust. J. Plant Physiol. 21, 475–495. doi: 10.1071/PP9940475
Farquhar, G. D., and Sharkey, T. D. (1982). Stomatal conductance and photosynthesis. Ann. Rev. Plant Physiol. 33, 317–345. doi: 10.1146/annurev.pp.33.060182.001533
Flexas, J., Barbour, M. M., Brendel, O., Cabrera, H. M., Carriquí, M., Díaz-Espejo, A., et al. (2012). Mesophyll diffusion conductance to CO2: An unappreciated central player in photosynthesis. Plant Sci. 193, 70–84. doi: 10.1016/j.plantsci.2012.05.009
Flexas, J., Barón, M., Bota, J., Ducruet, J. M., Gallé, A., Galmés, J., et al. (2009). Photosynthesis limitations during water stress acclimation and recovery in the drought-adapted Vitis hybrid Richter-110 (V. berlandieri x V. rupestris). J. Exp. Bot. 60, 2361–2377. doi: 10.1093/jxb/erp069
Flexas, J., Bota, J., Cifre, J., Escalona, J. M., Galmés, J., Gulías, J., et al. (2004b). Understanding down-regulation of photosynthesis under water stress: future prospects and searching for physiological tools for irrigation management. Ann. Bot. 144, 273–283. doi: 10.1111/j.1744-7348.2004.tb00343.x
Flexas, J., Bota, J., Escalona, J. M., Sampol, B., and Medrano, H. (2002). Effects of drought on photosynthesis in grapevines under field conditions: an evaluation of stomatal and mesophyll limitations. Funct. Plant Biol. 29, 461–471. doi: 10.1071/PP01119
Flexas, J., Bota, J., Loreto, F., Cornic, G., and Sharkey, T. D. (2004a). Diffusive and metabolic limitations of photosynthesis under drought and salinity in C3 plants. Plant Biol 6, 269–279. doi: 10.1055/s-2004-820867
Flexas, J., Diaz-Espejo, A., Gago, J., Gallé, A., Galmés, J., Gulíasa, J., et al. (2014). Photosynthetic limitations in Mediterranean plants: a review. Environ. Exp. Bot. 103, 12–23. doi: 10.1016/j.envexpbot.2013.09.002
Flexas, J., Diaz-Espejo, A., Galmés, J., Kaldenhoff, R., Medrano, H., and Ribas-Carbó, M. (2007). Rapid variations of mesophyll conductance in response to changes in CO2 concentration around leaves. Plant Cell Environ. 30, 1284–1298. doi: 10.1111/j.1365-3040.2007.01700.x
Flexas, J., and Medrano, H. (2002). Drought-inhibiton of photosynthesis in C3 plants: stomatal and non-stomatal limitations revisited. Ann. Bot. 89, 183–189. doi: 10.1093/aob/mcf027
Flexas, J., Niinemets, Ü., Gall, A., Barbour, M. M., Centritto, M., Diaz-Espejo, A., et al. (2013). Diffusional conductances to CO2 as a target for increasing photosynthesis and photosynthetic water-use efficiency. Photosyn. Res. 117, 45–59. doi: 10.1007/s11120-013-9844-z
Flexas, J., Ribas-Carbó, M., Bota, J., Galmés, J., Henkle, M., Martínez-Canellas, S., et al. (2006). Decreased Rubisco activity during water stress in not induced by decreased relative water content but related to conditions of low stomatal conductance and chloroplast CO2 concentration. New Phytol. 172, 73–82. doi: 10.1111/j.1469-8137.2006.01794.x
Flexas, J., Ribas-Carbó, M., Diaz-Espejo, A., Galmés, J., and Medrano, H. (2008). Mesophyll conductance to CO2: current knowledge and future prospects. Plant Cell Environ. 31, 602–612. doi: 10.1111/j.1365-3040.2007.01757.x
Gallé, A., Floréz-Sarasa, I. D., Tomás, M., Medrano, H., Ribas-Carbo, M., and Flexas, J. (2009). The role of mesophyll conductance during water stress and recovery in tobacco (Nicotiana sylvestris): acclimation or limitation? J. Exp. Bot. 60, 2379–2390. doi: 10.1111/gcb.13415
Gallé, A., Flórez-Sarasa, I., El Aouuad, H., and Flexas, J. (2011). The Mediterranean evergreen Quercus ilex the semi-deciduous Cistus albidus differ in their leaf gas exchange regulation and acclimation repeated drought and re-watering cycles. J. Exp. Bot. 62, 5207–5216. doi: 10.1093/jxb/err233
Galmés, J., Conesa, M. A., Ochogavía, J. M., Perdomo, J. A., Francis, D. M., Ribas-Carbó, M., et al. (2011). Physiological and morphological adaptations in relation to water use efficiency in Mediterranean accessions of Solanum lycopersicum. Plant Cell Environ. 2, 245–260. doi: 10.1111/j.1365-3040.2010.02239.x
Galmés, J., Medrano, H., and Flexas, J. (2007). Photosynthetic limitations in response to water stress and recovery in Mediterranean plants with different growth forms. New Phytol. 175, 81–93. doi: 10.1111/j.1469-8137.2007.02087.x
Genty, B., Briantais, J. M., and Baker, N. R. (1989). The relationship between the quantum yield of photosynthetic electron transport and quenching of chlorophyll fluorescence. Biochim. Biophys Acta 990, 87–92. doi: 10.1016/S0304-4165(89)80016-9
Grassi, G., and Magnani, F. (2005). Stomatal, mesophyll conductance and biochemical limitations to photosynthesis as affected by drought and leaf ontogeny in ash and oak trees. Plant Cell Environ. 28, 834–849. doi: 10.1111/j.1365-3040.2005.01333.x
Gu, J., Yin, X., Stomph, T. J., Wang, H., and Struik, P. C. (2012). Physiological basic of genetic variation in leaf photosyynthesis among rice (Oryza sativa L.) introgression lines under drought and well-watered conditions. J. Exp. Bot. 63, 5137–5153. doi: 10.1093/jxb/ers170
Hanba, Y. T., Shibasaka, M., Hayashi, Y., Hayakawa, T., Kasamo, K., Terahsima, I., et al. (2004). Overexpression of the barley aqauporin HvPIP2;1 increases internal CO2 conductance and CO2 assimilation in the leaves of transgenic rice plants. Plant Cell Biol. 45, 521–529.
Harley, P. C., Loreto, F., Di Marco, F., and Sharkey, T. D. (1992). Theoretical considerations when estimating the mesophyll conductance to CO2 flux by the analysis of the response of photosynthesis to CO2. Plant Physiol. 98, 1429–1436. doi: 10.1104/pp.98.4.1429
Heckwolf, M., Pater, D., Hanson, D. T., and Kaldenhoff, R. (2011). The Arabidopsis thaliana aquaporin AtPIP1;2 is a physiologically relevant CO2 transport facilitator. Plant J. 67, 795–804. doi: 10.1111/j.1365-313X.2011.04634.x
Jahan, E., Amthor, J. S., Farquhar, G. D., Trethowan, R., and Barbour, M. M. (2014). Variation in mesophyll conductance among Australian wheat genotypes. Funct. Plant Biol. 41, 568–580. doi: 10.1071/FP13254
Keenan, T., Sabate, S., and Gracia, C. (2010). The importance of mesophyll conductance in regulating forest ecosystem productivity during drought periods. Glob Change Biol. 16, 1019–1034. doi: 10.1111/j.1365-2486.2009.02017.x
Kjellbom, P., Larsson, C., Johanson, I., Karlsson, M., and Johanson, U. (1999). Aquaporins and water homeostasit in plants. Trends Plant Sci. 4, 308–314. doi: 10.1016/S1360-1385(99)01438-7
Laisk, A. (1977). Kinetics of Photosynthesis and Photorespiration in C3 Plants. Moscow: Nauka Moscow (in Russian).
Laisk, A., Eichelmann, H., Oja, V., Rasulov, B., Padu, E., Bichele, I., et al. (2005). Adjustment of leaf photosynthesis to shade in a natural canopy: rate parameters. Plant Cell Environ. 28, 375–388. doi: 10.1111/j.1365-3040.2004.01274.x
Lawlor, D. W., and Cornic, G. (2002). Photosynthetic carbon assimilation and associated metabolism in relation to water deficits in higher plants. Plant Cell Environ. 25, 275–294. doi: 10.1046/j.0016-8025.2001.00814.x
Lawlor, D. W., and Tezara, W. (2009). Causes of decreased photosynthetic rate and metabolic capacity in water deficient leaf cells: a critical evaluation of mechanisms and integration of processes. Ann. Bot. 103, 561–579. doi: 10.1093/aob/mcn244
Limousin, J. M., Misson, L., Lavoir, A. V., Martin, N. K., and Rambal, S. (2010). Do photosynthetic limitations of evergreen Quercus ilex leaves change with long-term increased drought severity? Plant Cell Environ. 33, 863–875. doi: 10.1111/j.1365-3040.2009.02112.x
Long, S. P., and Bernacchi, C. J. (2003). Gas exchange measurements, what can they tell us about the underlying limitations to photosynthesis? Procedures and sources of error. J. Exp. Bot. 54, 2393–2401. doi: 10.1093/jxb/erg262
Loreto, F., and Centritto, M. (2008). Leaf carbon assimilation in a water-limited world. Plant Biosyst. 142, 154–161. doi: 10.1080/11263500701872937
Loreto, F., Harley, P. C., Di Marco, G., and Sharkey, T. D. (1992). Estimation of mesophyll conductance to CO2 flux by three different methods. Plant Physiol. 98, 1437–1443. doi: 10.1104/pp.98.4.1437
Lu, C., Qiu, N., Wang, B., and Zhang, J. (2003). Salinity treatment shows no effects on photosystem II photochemistry, but increases the resistance of photosystem II to heat stress in halophyte Suaeda salsa. J. Exp. Bot. 383, 851–860. doi: 10.1093/jxb/erg080
Martin-Ruiz, B., and Torres, N. A. (1992). Effect of water-deficit stress on photosynthesis, its componentns and component limitations, and on water use efficiency in wheat (Triticum aestivum L.). Plant Physiol. 100, 733–739. doi: 10.1104/pp.100.2.733
Martorell, S., Diaz-Espejo, A., Medrano, H., Ball, M. C., and Choat, B. (2014). Rapid hydraulic recovery in Eucalyptus pauciflora after drought: linkages between stem hydraulics and leaf gas exchange. Plant Cell Environ. 37, 617–626. doi: 10.1111/pce.12182
McDowell, N. G. (2011). Mechanisms linking drought, hydraulics, carbon metabolism, and vegatation mortality. Plant Physiol. 155, 1051–1059. doi: 10.1104/pp.110.170704
Medrano, H., Escalona, J. M., Bota, J., Gulías, J., and Flexas, J. (2002). Regulation of photosynthesis of C3 plants in response to progressive drought: stomatal conductance as a reference parameter. Ann. Bot. 89, 895–905. doi: 10.1093/aob/mcf079
Medrano, H., Parry, M. A., Socias, X., and Lawlor, D. W. (1997). Long term water stress inactivates Rubisco in subterranean clover. Ann. App. Biol. 131, 491–501. doi: 10.1111/j.1744-7348.1997.tb05176.x
Misson, L., Limousin, J., Rodriguez, R., and Letts, M. G. (2010). Leaf physiological responses to extreme droughts in Mediterranean Quercus illex forest. Plant Cell Environ. 11, 1898–1910. doi: 10.1111/j.1365-3040.2010.02193.x
Muir, C. D., Hangarter, P., Moyle, L. C., and Davis, P. A. (2014). Morphological and anatomical determinats of mesophyll conductacne in wold relatives of tomato (Solanum sect. Lycopersicon, sect. Lycopersicoides; Solanaceae). Plant Cell Environ. 37, 1415–1426. doi: 10.1111/pce.12245
Nemani, R. R., Keeling, C. D., and Hashimito, H. (2003). Climate driven increases in global terrestri0061l net primary production from 1982 to 1999. Science 300, 1560–1563. doi: 10.1126/science.1082750
Ni, B. R., and Pallardy, S. G. (2009). Stomatal and non-stomatal limitations to net photosynthesis in seedlings of woody angiosperms. Plant Physiol. 99, 1502–1508. doi: 10.1104/pp.99.4.1502
Niinemets, Û., Díaz-Espejo, A., Flexas, J., Galmés, J., and Warren, C. R. (2009). Importance of mesophyll diffusion conductance in estimation of plant photosynthesis in the field. J. Exp. Bot. 60, 2271–2282. doi: 10.1093/jxb/erp063
Niinemets, Ü., and Keenan, T. (2014). Photosynthetic responses to stress in Mediterranean evergreens: mechanism and models. Environ. Exp. Bot. 103, 24–41. doi: 10.1016/j.envexpbot.2013.11.008
Parry, M. A. J., Adralojc, P. J., Lea, P. J., and Keys, A. J. (2002). Rubisco activity: effects of drought stress. Ann. Bot. 89, 833–839. doi: 10.1093/aob/mcf103
Perez-Martin, A., Flexas, J., Ribas-Carbó, M., Bota, J., Tomás, M., Infante, J. M., et al. (2009). Interactive effects of soil water deficit and air vapour pressure deficit on mesophyll conductance to CO2 in Vitis vinifera and Olea europaea. J. Exp. Bot. 60, 2391–2405. doi: 10.1093/jxb/erp145
Pons, T. L., and Welchen, R. A. M. (2003). Midday depression of net photosynthesis in the tropical rainforest tree Eperua grandiflora: contribution of stomatal and internal conductances, respiration and Rubisco functioning. Tree Physiol. 23, 937–947. doi: 10.1093/treephys/23.14.937
Rampino, P., Pataleo, S., Gerardi, C., Mita, G., and Perrotta, C. (2006). Drought stress response in wheat. Physiological and molecular analysis of resistant and sensitive genotypes. Plant Cell Environ. 29, 2143–2152. doi: 10.1111/j.1365-3040.2006.01588.x
Roupsard, O., Gross, P., and Dreyer, E. (1996). Limitation of photosynthetic activity by CO2 availability in the chloroplasts of oak leaves from different species and during drought. Ann. Sci. 30, 1559–1567. doi: 10.1051/forest:19960207
Sade, N., Gallé, A., Flexas, J., Lerner, S., Peleg, G., Yaaran, A., et al. (2014). Differential tissue-specific expression of NtAQP1 in Arabidopsis thaliana reveals a role for this protein in stomatal and mesophyll conductance of CO2 under standard and salt-stress conditions. Planta 239, 357–366. doi: 10.1007/s00425-013-1988-8
Sampol, B., Bota, J., Riera, D., Medrano, H., and Flexas, J. (2003). Analysis of the virus-induced inhibition of photosynthesis in malmsey grapevines. New Phytol. 160, 403–412. doi: 10.1046/j.1469-8137.2003.00882.x
Shao, H. B., Chu, L. Y., Jaleel, C. A., Manivannan, P., Panneerselvam, R., and Shao, M. A. (2009). Understanding water deficit stress-induced changes in the basic metabolism of higher plants – biotechnologically and sustainably improving agriculture and the eco-environment in arid regions of the globe. Cr. Rev. Biotechnol. 29, 131–151. doi: 10.1080/07388550902869792
Shao, H. B., Liang, Z. S., and Shao, M. A. (2006). Osmotic regulation of 10 wheat (Triticum aestivum L.) genotypes at soil water deficits. Colloid Surface B 47, 132–139. doi: 10.1016/j.colsurfb.2005.11.028
StPaul, N. K. M., Linousin, J. M., Rodriguez-Calcerrada, J., Ruffault, J., Rambal, S., Letts, M. G., et al. (2012). Photosynthetic sensitivity to drought varies among populations of Quercus ilex along a rainfall gradient. Funct. Plant Biol. 39, 25–37. doi: 10.1071/FP11090
Sun, J., Sun, J., and Feng, Z. (2015). Modelling photosynthesis in flag leaves of winter wheat (Triticum aestivum) considering the variation in photosynthesis parameters during development. Funct. Plant Biol. 42, 1036–1044. doi: 10.1071/FP15140
Tazoe, Y., von Caemmerer, S., Badger, M. R., and Evans, J. R. (2009). Light and CO2 do not affect the mesophyll conductance to CO2 diffusion in wheat leaves. J. Exp. Bot. 60, 2291–2301. doi: 10.1093/jxb/erp127
Tazoe, Y., von Caemmerer, S., Estavillo, G. M., and Evans, J. R. (2011). Using tunable diode laser spectroscopy to measure carbon isotope discrimination and mesophyll conductance to CO2 diffusion dynamically at different CO2 concentration. Plant Cell Environ. 34, 580–591. doi: 10.1111/j.1365-3040.2010.02264.x
Tezara, W., Mitchel, V. J., Driscoll, S. D., and Lawlor, D. W. (1999). Water stress inhibits plant photosynthesis by decreasing coupling factor and ATP. Nature 401, 914–917. doi: 10.1038/44842
Tomás, M., Flexas, J., Copolovici, L., Galmés, J., Hallik, L., Medrano, H., et al. (2013). Importance of leaf anatomy in determining mesophyll diffusion conductance to CO2 across species: quantitative limitations and scaling up by models. J. Exp. Bot. 64, 2269–2281. doi: 10.1093/jxb/ert086
Tosens, T., Niinements, Ü., Vislap, V., Eichelmann, H., and Castro Díez, P. (2012). Developmental changes in mesophyll diffusion conductance and photosynthetic capacity under different light and water availabilities in Populus tremula: how structure constrains function. Plant Cell Environ. 35, 839–856. doi: 10.1111/j.1365-3040.2011.02457.x
Valentini, R., Epron, D., De Angelis, P., Matteucci, G., and Dreyer, E. (1995). In situ estimation of net CO2 assimilation, photosynthetic electron flow and photorespiration in Turkey oak (Quercus cerris L.) leaves: diurnal cycles under different levels of water supply. Plant Cell Environ. 18, 631–640. doi: 10.1111/j.1365-3040.1995.tb00564.x
Vitousek, P. M., Field, C. B., and Matson, P. A. (1990). Variation in foliar Delta C13 in Hawaiian Metrosideros polymorpha – a case of internal resistance. Oecologia 84, 362–370. doi: 10.1007/BF00329760
von Caemmerer, S., and Evans, J. R. (1991). Determination of the average partial pressure of CO2 in chloroplast from leaves of several C3 plants. Aust. J. Plant Physiol. 18, 287–305. doi: 10.1071/PP9910287
Wang, H. X., Liu, C. M., and Zhang, L. (2002). Water-saving agriculture in China: an overview. Adv. Agron 75, 135–171. doi: 10.1016/S0065-2113(02)75004-9
Warren, C. R. (2008). Does growth temperature affect the temperature response of photosynthesis and internal conductance to CO2? A test with Eucalyptus regnas. Tree Physiol. 28, 11–19. doi: 10.1093/treephys/28.1.11
Warren, C. R., and Adams, M. A. (2006). Internal conductance does not scale with photosynthetic capacity: implications for carbon isotope discrimination and economics of water and nitrogen use in photosynthesis. Plant Cell Environ. 29, 192–201. doi: 10.1111/j.1365-3040.2005.01412.x
Warren, C. R., Ethier, G. J., Livingston, N. J., Grant, N. J., Turpin, D. H., Harrison, D. L., et al. (2003). Transfer conductance in second growth Douglas-fir (Pseudotsuga menziesii (Mirb.) Franco) canopies. Plant Cell Environ. 26, 1215–1227. doi: 10.1046/j.1365-3040.2003.01044.x
Wasson, A. P., Richards, R. A., Chatrath, R., Misra, S. C., Prasad, S. V., Rebetzke, G. J., et al. (2012). Traits and selection strategies to improve root systems and water uptake in water-limited wheat crops. J. Exp. Bot. 63, 3485–3498. doi: 10.1093/jxb/ers111
Yamori, W., Noguchi, K., Hanba, Y. T., and Terashima, I. (2006). Effects of internal conductance on temperature dependence of the photosynthetic rate in spinach leaves from contrasting growth temperatures. Plant Cell Physiol. 47, 1069–1080. doi: 10.1093/pcp/pcj077
Yan, K., Chen, P., Shao, H. B., Shao, C., Zhao, S., and Brestic, M. (2013). Dissection of photosynthetic electron transport process in sweet sorghum under heat stress. PLoS ONE 8:e62100. doi: 10.1371/journal.pone.0062100
Zadoks, J. C., Chang, T. T., and Konzak, C. F. (1974). A decimal code for the growth stages of cereals. Weed Res. 14, 415–421. doi: 10.1111/j.1365-3180.1974.tb01084.x
Zhao, H. Y., Gao, G., Yan, X. D., Zhang, Q., Hou, M. T., Zhu, Y. Y., et al. (2011). Risk assessment of agricultural drought using the CERES-wheat model: a case study of Henan plain, China. Climate Res. 50, 247–256. doi: 10.3354/cr01060
Zhu, J., Hasegawa, P. M., Chen, G., Wang, S., and Zhang, C. (2005). Different solute levels in two spring wheat cultivars induced by progressive field water stress at different development stages. J. Arid Environ. 62, 1–14. doi: 10.1016/j.jaridenv.2004.10.010
Zivcak, M., Brestic, M., Balatova, Z., Drevenakova, P., Olsovska, K., Kalaji, H. M., et al. (2013). Photosynthetic electron transport and specific photoprotective responses in wheat leaves under drought stress. Photosyn. Res. 117, 529–546. doi: 10.1007/s11120-013-9885-3
Keywords: photosynthesis, drought, mesophyll conductance, AN/Ci, carboxylation efficiency, wheat
Citation: Olsovska K, Kovar M, Brestic M, Zivcak M, Slamka P and Shao HB (2016) Genotypically Identifying Wheat Mesophyll Conductance Regulation under Progressive Drought Stress. Front. Plant Sci. 7:1111. doi: 10.3389/fpls.2016.01111
Received: 11 March 2016; Accepted: 12 July 2016;
Published: 08 August 2016.
Edited by:
Mohammad Anwar Hossain, Bangladesh Agricultural University, BangladeshReviewed by:
Weiqiang Li, RIKEN Center for Sustainable Resource Science, JapanJia Liu, Jiangsu Academy of Agricultural Sciences, China
Copyright © 2016 Olsovska, Kovar, Brestic, Zivcak, Slamka and Shao. This is an open-access article distributed under the terms of the Creative Commons Attribution License (CC BY). The use, distribution or reproduction in other forums is permitted, provided the original author(s) or licensor are credited and that the original publication in this journal is cited, in accordance with accepted academic practice. No use, distribution or reproduction is permitted which does not comply with these terms.
*Correspondence: Marian Brestic, bWFyaWFuLmJyZXN0aWNAZ21haWwuY29t
Hong Bo Shao, c2hhb2hvbmdib2NodUAxMjYuY29t