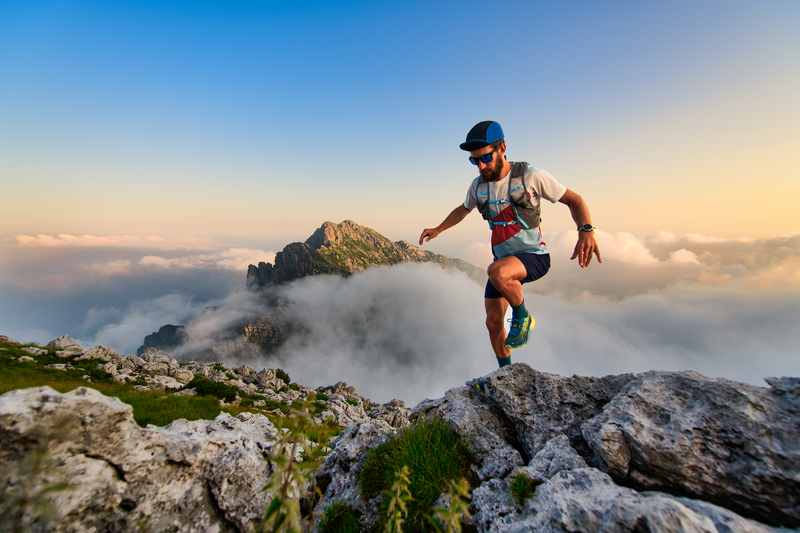
95% of researchers rate our articles as excellent or good
Learn more about the work of our research integrity team to safeguard the quality of each article we publish.
Find out more
ORIGINAL RESEARCH article
Front. Plant Sci. , 22 July 2016
Sec. Plant Biophysics and Modeling
Volume 7 - 2016 | https://doi.org/10.3389/fpls.2016.01092
Local stimulation induces generation and propagation of electrical signals, including the variation potential (VP) and action potential, in plants. Burning-induced VP changes the physiological state of plants; specifically, it inactivates photosynthesis. However, the mechanisms that decrease photosynthesis are poorly understood. We investigated these mechanisms by measuring VP-connected systemic changes in CO2 assimilation, parameters of light reactions of photosynthesis, electrochromic pigment absorbance shifts, and light scattering. We reveal that inactivation of photosynthesis in the pea, including inactivation of dark and light reactions, was connected with the VP. Inactivation of dark reactions decreased the rate constant of the fast relaxation of the electrochromic pigment absorbance shift, which reflected a decrease in the H+-ATP synthase activity. This decrease likely contributed to the acidification of the chloroplast lumen, which developed after VP induction. However, VP-connected decrease of the proton motive force across the thylakoid membrane, possibly, reflected a decreased pH in the stroma. This decrease may be another mechanism of chloroplast lumen acidification. Overall, stroma acidification can decrease electron flow through photosystem I, and lumen acidification induces growth of fluorescence non-photochemical quenching and decreases electron flow through photosystem II, i.e., pH decreases in the stroma and lumen, possibly, contribute to the VP-induced inactivation of light reactions of photosynthesis.
Local stimulation rapidly elicits systemic responses in plants (Gallé et al., 2015), including changes in gene expression (Stanković and Davies, 1996; Fisahn et al., 2004) and phytohormone production (Dziubinska et al., 2003; Hlaváčková et al., 2006; Hlavinka et al., 2012), increases in plant resistance to stressors (Retivin et al., 1997, 1999; Sukhov et al., 2014b, 2015a; Surova et al., 2016), the activation of respiration (Dziubinska et al., 1989; Filek and Kościelniak, 1997), etc. Numerous works have described the influence of local stimuli on photosynthetic processes (Hlaváčková et al., 2006; Krupenina and Bulychev, 2007; Grams et al., 2009; Pavlovič et al., 2011; Hlavinka et al., 2012; Sukhov et al., 2012, 2014a,b, 2015a,b; Vredenberg and Pavlovič, 2013; Bulychev and Komarova, 2014; Sherstneva et al., 2015, 2016; Surova et al., 2016), including reduced CO2 assimilation, decreases in the photosystem I (PSI) and photosystem II (PSII) quantum yields, the growth of fluorescence non-photochemical quenching (NPQ), and the activation of cyclic electron flow. Electrical signals, namely the action potential (AP), which is mainly induced by non-damaging stimuli, and the variation potential (VP), which is mainly caused by damaging stimuli, are the most likely links between stimulated and non-stimulated zones during the systemic responses of plants (Sukhov, 2016).
The AP is a self-propagating electrical signal that is primarily related to passive ions fluxes, including Ca2+, Cl-, and K+ fluxes (Beilby, 1984, 2007; Dziubinska, 2003; Krol et al., 2004; Felle and Zimmermann, 2007; Sukhov et al., 2011). The VP is a local electrical reaction to hydraulic and/or chemical signal propagation (Malone, 1994; Stahlberg and Cosgrove, 1996; Mancuso, 1999; Vodeneev et al., 2012, 2015; Sukhov et al., 2013). Transient H+-ATPase inactivation is the main mechanism of VP generation (Julien et al., 1991; Stahlberg and Cosgrove, 1997; Vodeneev et al., 2015), but ion fluxes also participate in the reaction (Julien et al., 1991; Vodeneev et al., 2011, 2015; Katicheva et al., 2014). According to studies of Chara alga by Krupenina and Bulychev (2007), Bulychev and Komarova (2014), the influence of AP on photosynthesis is likely a function of Ca2+ flux into cell. However, the influence of VP on photosynthesis in higher plants likely involves another mechanism. Numerous works (Grams et al., 2009; Sukhov et al., 2014a; Sherstneva et al., 2015, 2016) have reported that the VP-connected H+ influx is a potential mechanism of photosynthetic inactivation.
Variation potentials appear to affect photosynthesis in different ways (Sukhov, 2016). Inactivation of dark reactions of photosynthesis is an important mechanism of the photosynthetic response (Sukhov et al., 2012, 2014a,b, 2015b; Sherstneva et al., 2015), and a decreased flow of CO2 into mesophyll cells is likely responsible for photosynthesis inactivation (Gallé et al., 2015; Sukhov, 2016). This decreased flux can result from an increase in the :CO2 ratio in the apoplast, the inactivation of aquaporins, or changes in carbonic anhydrase activity (Grams et al., 2009; Gallé et al., 2013; Sukhov et al., 2014a; Sherstneva et al., 2015). These decreases in the CO2 flow can all be associated with the changes in cytoplasmic acidification and apoplastic alkalization observed during VP generation (Grams et al., 2009; Sukhov et al., 2014a; Sherstneva et al., 2015, 2016).
Changes in parameters of light reactions of photosynthesis can be also observed after propagation of electrical signals (Krupenina and Bulychev, 2007; Grams et al., 2009; Pavlovič et al., 2011; Sukhov et al., 2012, 2014a,b, 2015a,b; Vredenberg and Pavlovič, 2013; Sherstneva et al., 2015, 2016; Surova et al., 2016). These changes demonstrate that electrical signals influence the thylakoid membrane (Sukhov, 2016), and the influence may be connected with inactivation of dark reactions of photosynthesis, an increase in the ATP:ADP ratio in the chloroplast stroma, and the inactivation of H+-ATP synthase (Pavlovič et al., 2011; Sukhov et al., 2012, 2014a; Sukhov, 2016). Furthermore, the VP can decrease electron flow through the acceptor side of PSI (Sukhov et al., 2012) and increase NPQ (Sukhov et al., 2014a) independently of inactivation of dark reactions of photosynthesis. The last responses may be related to pH changes in the chloroplast stroma and lumen (Sukhov et al., 2014b, 2015a; Sukhov, 2016), but this relationship has not yet been experimentally investigated. Thus, an experimental investigation of the influence of the VP on the H+-ATP synthase activity, proton gradient across the thylakoid membrane, and pH in the chloroplast is important to understand the mechanism underlying the photosynthetic response.
The registration of changes in green light absorption by photosynthetic pigments, including the ‘electrochromic pigment absorbance shift’ (ECS) and ‘light scattering’ (LS), are classical, widely used, non-invasive methods used to investigate electrical and proton gradients across thylakoid membranes (Deamer et al., 1967; Murakami and Packer, 1970; Ivanov et al., 2001; Avenson et al., 2004; Schreiber and Klughammer, 2008; Bailleul et al., 2010; Klughammer et al., 2013; Wang et al., 2015). It should be noted that ECS and LS are often measured in intact leaves or in segments of leaves (Kramer and Crofts, 1989; Sacksteder et al., 2000; Ruban et al., 2002; Schreiber and Klughammer, 2008; Klughammer et al., 2013; Wang et al., 2015).
The ECS is a change in the leaf absorbance between 515 and 525 nm, considered to be proportional to the electrical potential across the thylakoid membrane, and associated with carotenoids and Chl b (Schreiber and Klughammer, 2008; Klughammer et al., 2013). The proton electrochemical gradient (proton motive force, pmf), transmembrane electrical potential (ΔΨ), and proton gradient (ΔpH) can be estimated using the ECS relaxation after the ‘light-dark’ transition (Avenson et al., 2004; Schreiber and Klughammer, 2008; Bailleul et al., 2010; Klughammer et al., 2013). This relaxation can also be used to calculate the H+-ATP synthase activity (Morita et al., 1982; Kramer and Crofts, 1989; Sacksteder et al., 2000; Klughammer et al., 2013; Wang et al., 2015).
Light scattering is a change in the leaf absorbance at approximately 535 nm that is characterized by slow relaxation kinetics (minutes), independently from the ECS (Schreiber and Klughammer, 2008). LS is caused by the internal acidification of thylakoids upon light-induced ΔpH formation (Deamer et al., 1967; Schreiber and Klughammer, 2008); this relationship is supported by the monotonous growth of LS in response to pH decreases in the physiological range (Deamer et al., 1967; Murakami and Packer, 1970). Aggregation of light harvesting complexes in thylakoids, which is connected with protonation of these complexes, is a probable mechanism for the shift in LS (Horton et al., 1991, 2005; Ruban et al., 2002). Therefore, LS reflects the luminal pH in the chloroplast and can be used as a semi-quantitative indicator of membrane energization (Schreiber and Klughammer, 2008).
The aims of this study were to investigate the influence of the VP on the electrical and proton gradients across thylakoid membranes, the H+-ATP synthase activity, and the pH of pea leaves (Pisum sativum L.).
Pea seedlings (14–21 days old) were used in this investigation. Seedlings were cultivated hydroponically in a Binder KBW 240 plant growth chamber (Binder GmbH, Tuttlingen, Germany) at 24°C, with a 16/8-h (light/dark) photoperiod. White light was used (∼100 μmol m-2 s-1).
Local burning is widely used to stimulate the VP in plants (Stanković and Davies, 1996; Hlaváčková et al., 2006; Sukhov et al., 2012, 2014b; Vodeneev et al., 2015); in particular, flames are most commonly used to investigate the influence of electrical signals on photosynthesis (Hlaváčková et al., 2006; Grams et al., 2009; Sukhov et al., 2012, 2014b; Sherstneva et al., 2015, 2016; Surova et al., 2016). Therefore, the VP was induced by burning the tip of the first mature leaf (flame, 3–4 s, ∼1 cm2), as shown in Figure 1A. This burning was localized and did not change the temperature of the adjacent leaves and stem.
FIGURE 1. Positions of burning (flame, 3–4 s, ∼1 cm2), electrical potential monitoring, and photosynthetic and light absorption parameter measurements in plants. (A) EL and ES, Ag+/AgCl electrodes connected to the leaf and stem, ER, reference electrode; distance between EL and ES, 3–4 cm. ElowCO2 and Econtrol, electrodes from silver wire. (B) Kinetics of leaf light absorption at 530 nm, A530. (C) Kinetics of differences between light absorption at 515 nm and absorption at 550 nm, A515–A550. LS, light scattering; ECSpmf, ECSΔΨ, and ECSΔpH represent electrochromic pigment absorbance shifts proportional to the proton motive force, transmembrane electrical potential, and proton gradient on the thylakoid membrane, respectively.
The extracellular measurement of electrical activity was primarily conducted using Ag+/AgCl electrodes (RUE “Gomel Measuring Equipment Plant,” Gomel, Belarus), a high-impedance (1012 Ω) amplifier IPL-113 (Semico, Novosibirsk, Russia), and a personal computer. First, an electrode was placed on the stem close to the second mature leaf (ES), and a second electrode (EL) was then placed at the center of the leaflet of this leaf; the distance between ES and EL was 3–4 cm. The electrodes contacted the seedling via ‘Uniagel’ conductive gel (Geltek-Medica, Moscow, Russia). The reference electrode (ER) was placed in standard solution (1 mM KCl. 0.5 mM CaCl2, 0.1 mM NaCl) surrounding the root.
In a separate experimental series, the influence of a low CO2 concentration on the VP parameters was investigated using electrodes consisting of silver wire (0.5-mm diameter) and a pointed tip. The first silver electrode (ElowCO2) was placed at the center of a leaflet in the photosynthesis-measuring head (see below). The second silver electrode (Econtrol) was placed at the center of the second leaflet on the same leaf. The reference electrode (ER) was placed in standard solution surrounding the root. The CO2 concentration was controlled using a photosynthesis measuring system (see below).
A standard system (Heinz Walz GmbH, Effeltrich, Germany) consisting of a portable gas exchange measuring system (GFS-3000), a measuring system for the simultaneous assessment of P700 oxidation and chlorophyll fluorescence (Dual-PAM-100), and a measuring head (Cuvette 3010-Dual) were used to measure photosynthetic parameters.
The photosynthetic parameters were measured under red actinic light (630 nm, 278 μmol m-2 s-1), a controlled CO2 concentration (360 ppm in the most of experiments or approximately 10 ppm (from 7 to 12 ppm) in experiment with low CO2 concentration), 67–72% relative humidity, and a temperature of 23°C. The standard functions of the Dual-PAM-100 (light conditions), GFS-3000 (CO2 concentration and humidity conditions), and 3010-Dual cuvette (temperature conditions) were used to control the conditions.
The photosynthetic parameters were measured as previously described (Sukhov et al., 2014a, 2015b). The dark (F0) and maximal (Fm) fluorescence yields (Maxwell and Johnson, 2000; Kalaji et al., 2012, 2014) were measured after dark adaptation for 20 min. The maximal change in the P700 signal (Pm) of PSI, reflecting maximal P700 oxidation (Klughammer and Schreiber, 2008), was measured after preliminary illumination by far red light for 10 s. Later the steady-state (F) and maximal (F′m) fluorescence yields in light (Maxwell and Johnson, 2000) and steady-state (P) and maximal (P′m) P700 signals in light (Klughammer and Schreiber, 2008) were measured using saturation pulses generated every 10 s. Quantum yield of PSI (ϕPSI) was calculated using the equation ϕPSI = (Pm′ - P)/Pm (Klughammer and Schreiber, 2008); quantum yield of PSII (ϕPSII) was calculated using the equation ϕPSII = (Fm′ - F)/Fm′ (Maxwell and Johnson, 2000); fluorescence non-photochemical quenching (NPQ) was calculated using the equation NPQ = (Fm - Fm′)/Fm′ (Maxwell and Johnson, 2000; Kalaji et al., 2012). The CO2 assimilation rate (ACO2, μmol CO2⋅m-2⋅s-1) was measured using the GFS-3000 system and its software, and the parameter programmatically calculated according to von Caemmerer and Farquhar (1981).
A Dual-PAM-100 with P515/535 emitter–detector modules, GFS-3000, and 3010-Dual cuvette (Heinz Walz GmbH, Effeltrich, Germany) were used to measure LS and the ECS.
Light scattering at 530 nm was used to qualitatively estimate the pH in the lumen because it reflected the internal acidification of thylakoids upon light-induced ΔpH formation (Deamer et al., 1967; Murakami and Packer, 1970; Schreiber and Klughammer, 2008). Periodic ‘light-dark’ transitions were used to analyze LS. For each cycle, the duration of illumination by red actinic light was 450 s, and the duration of darkening was 150 s. The magnitude of LS was assessed by measuring the change in absorption with slow relaxation kinetics (∼90–120 s) according to Schreiber and Klughammer (2008). Figure 1B shows the methodology used to measure LS. The mean LS magnitude, before VP or a CO2 decrease was assumed to be 100%; relative LS were used in the analysis.
Differences between light absorption at 515 and 550 nm were used to analyze the ECS (Schreiber and Klughammer, 2008). Changes in this difference under the light-dark transition with different relaxation kinetics were used to estimate the pmf (ECSpmf), ΔΨ (ECSΔΨ), and proton gradient (ECSΔpH). The methods used to estimate the ECSpmf, ECSΔΨ, and ECSΔpH (Avenson et al., 2004; Schreiber and Klughammer, 2008; Bailleul et al., 2010; Klughammer et al., 2013) are shown in Figure 1C. The mean electrochromic shift before VP or a CO2 decrease was assumed to be 100%; relative ECSpmf, ECSΔΨ, and ECSΔpH values were used for the analysis.
According to several studies (Morita et al., 1982; Sacksteder et al., 2000; Wang et al., 2015) the rapid relaxation of ECS under dark conditions reflects the H+-ATP synthase activity because the proton flux through H+-ATP synthase is likely the main mechanism underlying proton motive force changes after the onset of darkness. In contrast to most methods of chemical or molecular analysis, measuring rapid ECS relaxation can be used to investigate the dynamics of rapid (seconds and minutes) changes in the H+-ATP synthase activity in intact leaves; furthermore, this method is relatively simple. Therefore, we used ECS relaxation measurements to analyze changes in the H+-ATP synthase activity after VP or a CO2 decrease. The rate constant of rapid ECS relaxation (kECS) was estimated by fitting the first 80 ms of the decay curve with a first-order exponential decay kinetic as the inverse of the decay time constant. Based on the approach of Wang et al. (2015), the kECS reflected the proton conductivity of H+-ATP synthase.
Two variants of periodic ‘light-dark’ transitions were used to analyze the ECS. The ECSΔΨ and ECSΔpH were estimated under light:dark conditions of 450 s:150 s; this condition was similar to that used to investigate LS. The kECS was calculated under light:dark conditions of 50 s:10 s. The ECSpmf was estimated for both light:dark regimens.
The conditions of these measurements were similar to those used for photosynthetic investigations. A photosynthesis measuring system was used to control conditions.
Each measurement was performed on a separate plant. Representative records, mean values, and standard errors were determined and are presented in the figures. Numbers of replicates are shown in the figures. Significant differences were determined according to the Student’s t-test.
The local burning of the first leaf induced a propagating electrical signal observable in the stems and second leaves of pea plants (Figure 2). The mean VP amplitudes were 64 ± 3 mV in the stem and 52 ± 6 mV in the leaf. The average time between the appearance of the VP in the stem and a leaflet at the center of the second leaf was approximately 100 s. The duration of the VP was at least 20–60 min, and the electrical reaction shape was variable.
FIGURE 2. Changes in the surface electrical potential of the stem near the second leaf (ES) and at the center of its leaflet (EL), as induced by the burning of the first leaf (n = 15).
Upon propagating into the leaf, the VP decreased the CO2 assimilation, ϕPSI and ϕPSII and increased NPQ (Figure 3A). The characteristics of these changes are shown in Table 1. Photosynthetic parameters began to change 1-2 min after the start of VP in the leaf. The VP amplitude in the leaf significantly correlated with the magnitudes of changes in the ACO2 and NPQ (Table 1). Time of beginning of VP in the leaf was significantly correlated with time of beginning of changes in the ACO2 and NPQ (Table 1). A connection between changes in the ACO2 and parameters of light reactions of photosynthesis was also observed (Table 1).
FIGURE 3. Changes in the photosynthetic parameters induced by VP at 360 ppm and approximately 10 ppm CO2 (n = 5–10). (A) Changes in the ACO2 induced by VP at 360 ppm CO2. (B) Changes in ACO2 induced by VP at approximately 10 ppm CO2. (C) Changes in parameters of light reactions of photosynthesis induced by VP at 360 ppm CO2. (D) Changes in parameters of light reactions of photosynthesis induced by VP at approximately 10 ppm CO2. VP was induced by burning the first mature leaf (arrow).
TABLE 1. Characteristics of changes in photosynthetic parameters after VP induction and CO2 concentration lowering.
A decrease in the CO2 concentration decreased the CO2 assimilation, ϕPSI and ϕPSII and increased NPQ (Figure 3B, Table 1), and these changes were similar to the VP-induced photosynthetic response. The VP-induced photosynthetic response was weak at low CO2 concentration (∼10 ppm). All changes, excluding ϕPSI changes, were significantly lower than those observed at the atmospheric CO2 concentration (Table 1).
Figure 4 shows the influence of a decreased CO2 concentration on the surface membrane potential and VP parameters. Decreasing the CO2 concentration decreased the surface potential (Figure 4A) by approximately 15 mV (Figure 4B) but did not influence the VP amplitude (Figures 4A,B). Moreover, the VP amplitudes under low CO2 conditions and control conditions strongly correlated (correlation coefficient was 0.77, p < 0.05), whereas the change in the surface potential after decreasing the CO2 concentration and VP amplitude did not correlate (data not shown). Notably, the VP measured by silver electrodes (Figure 4A) did not significantly differ from the VP measured by Ag+/AgCl electrodes in leaves (Figure 2). Differences in amplitudes were also insignificant.
FIGURE 4. Influence of decreasing the CO2 concentration on the surface membrane potential and VP in leaves (n = 7). (A) Changes in the surface electrical potential in leaflets at approximately 10 ppm CO2 concentration (ElowCO2) and in control leaflets (Econtrol). (B) Mean VP and CO2 decrease-induced changes in the surface potential. Significant differences between variants in Figure 4B were absent (Student’s t-test).
Local burning and, probably, propagation of burning-induced VP decreased the rate constant of rapid ECS relaxation (kECS, Figure 5A, Table 2), reflecting the proton conductivity of the H+-ATP synthase decrease (Wang et al., 2015). The minimum of rate constant (∼70% of the initial rate) was observed 2–7 min after the induction of the VP. Decreasing the CO2 concentration (Figure 5B, Table 2) also decreased the kECS, and the minimal value was approximately 40% of the initial rate. However, the VP did not decrease the kECS under low CO2 concentration.
FIGURE 5. Changes in the rate constant of the rapid relaxation of the electrochromic pigment absorbance shift (kECS), reflecting the proton conductivity of H+-ATP synthase, and the relative electrochromic shift, reflecting the proton motive force (relative ECSpmf) induced by VP at 360 ppm (A) and approximately 10 ppm (B) CO2 (n = 5–6). The mean ECSpmf magnitudes prior to VP or a decrease in the CO2 level were assumed to be 100%. Points were measured every 60 s (50 s light, 10 s dark). The VP was induced by burning the first mature leaf (arrow).
TABLE 2. Relative changes in the ECS and LS parameters after VP induction and CO2 concentration decrease.
The VP induced a weak decrease in the relative ECSpmf, reflecting the VP-induced changes in the proton motive force. Figure 5B shows that decreasing the CO2 level increased the relative ECSpmf, whereas the VP decreased the proton motive force at low CO2 concentration. The magnitude of the VP-induced ECSpmf decrease at a low CO2 concentration was greater than that observed at atmospheric CO2 concentration.
Local burning and, probably, propagation of burning-induced VP changed the relative ECSpmf, ECSΔΨ, and ECSΔpH reflecting proton motive force, ΔΨ, and ΔpH across the thylakoid membrane (Figure 6A; Table 2). Under a light:dark regimen of 450-s light:150-s dark, the VP decreased the ECSpmf, similar to the proton motive force decreases observed under a 50-s light:10-s dark regimen. The ECSΔΨ and ECSΔpH also decreased after VP induction, which reflected a reduction in the ΔΨ and ΔpH. Decrease of the CO2 concentration (Figure 6B, Table 2) increased the ECSpmf and ECSΔpH but only weakly influenced the ECSΔΨ. The VP significantly decreased all investigated parameters at low CO2 concentration. The magnitudes of the VP-induced ECSpmf, ECSΔΨ, and ECSΔpH decreases at low CO2 concentration were larger than those under control conditions.
FIGURE 6. Changes in the relative ECSpmf, ECSΔΨ, and ECSΔpH induced by VP at 360 ppm (A) and approximately 10 ppm (B) CO2 (n = 6–8). The mean ECSpmf magnitudes prior to VP or a decrease in the CO2 were assumed to be 100%. Points were measured every 600 s (450 s light, 150 s dark). The VP was induced by burning the first mature leaf (arrow).
Figure 7A and Table 2 show that the VP transiently increased LS, which likely reflects a pH decrease in the thylakoid. Maximum LS growth occurred approximately 14 min after VP induction. Decreasing the CO2 concentration also increased LS (Figure 7B, Table 2). The VP also increased LS at low CO2 concentration, but this LS growth was less pronounced than that observed under control conditions.
FIGURE 7. Changes in the relative LS induced by VP at 360 ppm (A) and approximately 10 ppm (B) CO2 (n = 6–8). The mean LS magnitudes prior to VP or a decrease in the CO2 were assumed to be 100%. Points were measured every 600 s (450 s light, 150 s dark). The VP was induced by burning the first mature leaf (arrow).
Local burning induced VP propagation (Figures 2 and 4) and elicited a photosynthetic response in undamaged pea leaves under red actinic light (Figure 3A, Table 1). VP propagation is based on hydraulic and/or chemical signal propagation (Malone, 1994; Stahlberg and Cosgrove, 1996; Mancuso, 1999; Vodeneev et al., 2012, 2015; Sukhov et al., 2013); therefore, the first question is ‘does the VP induce a photosynthetic response or can hydraulic and/or chemical signals influence photosynthesis without a VP?’ Literature data (Grams et al., 2007) show that electrical and hydraulic signals can have distinct effects on leaf gas exchange. Our results showed that the VP amplitude was strongly correlated with the magnitudes of local burning-induced changes in CO2 assimilation and NPQ, and the initiation time of the VP in the leaf was strongly correlated with the initiation time of changes in these photosynthetic parameters (Table 1). These correlations can be explained by (i) hydraulic and/or chemical signals having very similar effects on electrical activity and photosynthesis, (ii) the effect of the photosynthetic response on electrical activity and (iii) the effect of the VP on photosynthesis. There are a number of arguments supporting the last supposition. First, the VP was propagated into undamaged leaves 1–2 min before the initiation of the photosynthetic response. Second, our previous results (Sukhov et al., 2014a; Sukhov, 2016) showed that imitation of a VP-connected proton influx (treatment of protonophores) caused a photosynthetic response in pea leaves, and the response was similar to the response induced by a VP. Third, the VP-induced photosynthetic response was very similar to the AP-induced response (Krupenina and Bulychev, 2007; Pavlovič et al., 2011), but AP is not connected with hydraulic or chemical signals (Fromm and Lautner, 2007). Thus, we suppose that VP induces a photosynthetic response in peas under red light.
According to previous studies (Krupenina and Bulychev, 2007; Pavlovič et al., 2011; Sukhov et al., 2012, 2014a,b, 2015b; Sukhov, 2016), electrical signal-induced inactivation of the dark reactions of photosynthesis is the main initial mechanism of the photosynthetic response. The following hypothetical chain of events was previously proposed to explain the photosynthetic response (Pavlovič et al., 2011; Sukhov et al., 2012, 2014a): electrical signals (AP or VP) → inactivation of the dark reactions of photosynthesis → an increase in the ATP:ADP ratio → inactivation of H+-ATP synthase → a decrease in H+ flux from the lumen to the stroma → alkalization of the chloroplast stroma and acidification of its lumen → an increase in the proton electrochemical gradient across thylakoid membranes → inactivation of the light reactions of photosynthesis.
The results of the current study show that inactivation of the dark reactions is involved in the initiation of the photosynthetic response. There are three groups of arguments to support this, as follows. (i) The magnitude of VP-induced ACO2 inactivation was significantly correlated with the magnitudes of changes in the parameters of the light reactions of photosynthesis. (ii) Artificial suppression of the dark reactions of photosynthesis, caused by lowering the CO2 concentration, decreased the quantum yields of PSI and PSII and increased NPQ (Figure 3D, Table 1); these changes were similar to VP-induced changes (Figure 3C, Table 1). (iii) The magnitudes of VP-induced changes in the parameters of photosynthetic light reactions were decreased at a low CO2 concentration (Figure 3D, Table 1). This effect was not connected with changes in VP parameters under a low CO2 concentration because the amplitudes and shapes of the VPs were similar at low and atmospheric CO2 concentrations (Figure 4). Thus, the photosynthetic response in peas under red light is primarily initiated by inactivation of the dark reactions of photosynthesis; i.e., the mechanisms of the VP-induced photosynthetic responses under blue light (Sukhov et al., 2014a,b, 2015b) and red light (current study) are similar.
However, the strong suppression of dark reaction inactivation under low CO2 concentrations did not strongly inactivate the responses of the photosynthetic light reactions, especially those of ϕPSI and ϕPSII (the magnitudes of the changes were 79% and 66% from their magnitudes under the atmospheric CO2 concentration). This result shows that the influence of VP on the light reactions of photosynthesis can be observed without inactivating the dark reactions under red light; i.e., additional pathways are involved. It is also in good agreement with our previous results in peas under blue light (Sukhov et al., 2014a, 2015b). According to our previous hypothesis (Sukhov et al., 2012, 2014a; Sukhov, 2016), proton flux from the chloroplast to the stroma and lumen, and their acidification, may be an additional mechanism by which VP affects the light reactions; however, this supposition needs experimental analysis.
We also investigated the effect of VP on the activity of H+-ATP synthase using ECS relaxation (Morita et al., 1982; Sacksteder et al., 2000; Schreiber and Klughammer, 2008; Wang et al., 2015). Our results indicated that the VP decreased the rate constant of ECS relaxation (Figure 5A, Table 2), reflecting a decrease in the proton conductivity of H+-ATP synthase (Wang et al., 2015). Artificial suppression of the dark reactions of photosynthesis, by lowering the CO2 concentration, also decreased the rate constant of ECS relaxation, and the VP did not induce significant changes in kECS under a low CO2 concentration (Figure 5B, Table 2). Our results experimentally support the hypothesis that H+-ATP synthase activity is decreased after electrical signal-induced suppression of the dark reactions of photosynthesis (Pavlovič et al., 2011; Sukhov et al., 2012, 2014a).
The decrease in H+-ATP synthase activity after suppression of the dark reactions of photosynthesis probably results in an increase of the proton motive force (proton electrochemical gradient) across thylakoid membranes (Pavlovič et al., 2011; Sukhov et al., 2012, 2014a, 2015b). Here, artificial suppression of the dark reactions of photosynthesis increased the ECSpmf, ECSΔpH, and ECSΔΨ (Figure 6B, Table 2), which reflect the proton motive force, proton gradient, and ΔΨ across thylakoid membranes, respectively (Avenson et al., 2004; Schreiber and Klughammer, 2008; Bailleul et al., 2010). LS and NPQ, which are connected with lumen acidification (Deamer et al., 1967; Murakami and Packer, 1970; Ruban et al., 1993; Maxwell and Johnson, 2000; Müller et al., 2001; Schreiber and Klughammer, 2008; Goss and Lepetit, 2015), were also stimulated after lowering the CO2 concentration (Figures 3D and 7B, Tables 1 and 2).
However, our results showed (Figures 5A and 6A, Table 2) that VP moderately decreased the ECSpmf, ECSΔpH, and ECSΔΨ under an atmospheric CO2 concentration; i.e., VP probably reduce the proton motive force, proton gradient, and ΔΨ across thylakoid membranes.
Two a priori hypotheses can be proposed for the VP-induced ΔpH decrease: (i) an increase in the internal pH of the thylakoid lumen or (ii) a decrease in the pH of the chloroplast stroma. The first hypothesis is not supported by the experimental data. Firstly, we found that VP induction increased LS (Figure 7A, Table 2), reflecting the internal acidification of the thylakoids (Deamer et al., 1967; Murakami and Packer, 1970; Schreiber and Klughammer, 2008), i.e., the luminal pH is probably decreased after VP propagation. Secondly, the VP-induced increase in NPQ (Figure 3C, Table 1) supports a decrease of luminal pH because lumen acidification is known to increase NPQ (Ruban et al., 1993; Maxwell and Johnson, 2000; Müller et al., 2001; García-Plazaola et al., 2012; Goss and Lepetit, 2015). Thirdly, a decrease of H+-ATP synthase activity (a decrease of proton efflux from the lumen to the stroma) can also stimulate lumen acidification.
The second hypothesis implies that the ΔpH decrease is connected with a decrease in the pH of the chloroplast stroma. This hypothesis explains the simultaneous ΔpH decrease (reduction in ECSΔpH) and luminal pH decrease (LS and NPQ increases), i.e., it is very probable. Additionally, the second hypothesis is well supported by literature data, which demonstrate that VP generation is accompanied by H+-ATPase inactivation in the plasma membrane (Sukhov et al., 2013; Katicheva et al., 2014; Vodeneev et al., 2015) and a decrease in the pH of the cytoplasm (Grams et al., 2009; Sukhov et al., 2014a; Sherstneva et al., 2015, 2016); notably, this decrease was observed in pea seedlings (Sukhov et al., 2014a; Sherstneva et al., 2016). The acidification of the cytoplasm can contribute to proton flux into the stroma through different H+-transporting systems in the membrane envelope (Peters and Berkowitz, 1991; Wu and Berkowitz, 1992; Song et al., 2004). Moreover, a stromal pH decrease can contribute to a decrease of the luminal pH via proton transport through the photosynthetic electron-transport chain (Allen, 2003), i.e., this decrease may participate in VP-induced lumen acidification.
The VP-induced decrease in the proton motive force is likely connected to the ΔpH decrease (Figure 6A, Table 2). However, the tendency of ΔΨ to decrease after a VP, which also decreases the proton motive force, may be related to the acidification of the thylakoid lumen because a luminal pH decrease can suppress the photosynthetic electron-transport chain activity (Kramer et al., 1999; Tikhonov, 2013, 2014).
Variation potential-induced stroma and lumen acidification can inactivate the light reactions of photosynthesis. Decrease in the stromal pH is known to change ferredoxin-NADP+ reductase localization (Benz et al., 2010), which suppresses electron flow through PSI. Moreover, decrease in the lumen pH is well known to stimulate NPQ (Ruban et al., 1993; Maxwell and Johnson, 2000; Müller et al., 2001) and directly suppresses photosynthetic electron-transport chain activity (Kramer et al., 1999; Tikhonov, 2013, 2014). Both processes decrease the quantum yields of the photosystems, i.e., inactivate photosynthesis. It is possible that these mechanisms participate in additional pathways by which electrical signals affect the light reactions of photosynthesis because an electrical signal-induced photosynthetic response can develop without inactivation of the dark reactions of photosynthesis (Sukhov et al., 2012, 2014a, 2015b; Vredenberg and Pavlovič, 2013; Sukhov, 2016).
VS conceived and supervised the project. VS and VV designed the experiments. LS, EM, and OS performed the experiments. VS, LS, and VV analyzed the data. VS and VV wrote the manuscript. All authors participated in the discussions of the results and the preparation of the manuscript.
This work was supported by the Russian Science Foundation: research project No. 14-26-00098.
The authors declare that the research was conducted in the absence of any commercial or financial relationships that could be construed as a potential conflict of interest.
Allen, J. F. (2003). Cyclic, pseudocyclic and noncyclic photophosphorylation: new links in the chain. Trends Plant Sci. 8, 15–19. doi: 10.1016/S1360-1385(02)00006-7
Avenson, T. J., Cruz, J. A., and Kramer, D. M. (2004). Modulation of energy-dependent quenching of excitons in antennae of higher plants. Proc. Natl. Acad. Sci. U.S.A. 101, 5530–5535. doi: 10.1073/pnas.0401269101
Bailleul, B., Cardol, P., Breyton, C., and Finazzi, G. (2010). Electrochromism: a useful probe to study algal photosynthesis. Photosynth. Res. 106, 179–189. doi: 10.1007/s11120-010-9579-z
Beilby, M. J. (1984). Calcium and plant action potentials. Plant Cell Environ. 7, 415–421. doi: 10.1111/j.1365-3040.1984.tb01431.x
Beilby, M. J. (2007). Action potential in charophytes. Int. Rev. Cytol. 257, 43–82. doi: 10.1016/S0074-7696(07)57002-6
Benz, J. P., Stengel, A., Lintala, M., Lee, Y. H., Weber, A., Philippar, K., et al. (2010). Arabidopsis Tic62 and ferredoxin-NADP(H) oxidoreductase form light-regulated complexes that are integrated into the chloroplast redox poise. Plant Cell 21, 3965–3983. doi: 10.1105/tpc.109.069815
Bulychev, A. A., and Komarova, A. V. (2014). Long-distance signal transmission and regulation of photosynthesis in characean cells. Biochemistry (Mosc). 79, 273–281. doi: 10.1134/S0006297914030134
Deamer, D. W., Crofts, A. R., and Packer, L. (1967). Mechanisms of light-induced structural changes in chloroplasts I. Light-scattering increments and ultrastructural changes mediated by proton transport. Biochim. Biophys. Acta 131, 81–96. doi: 10.1016/0005-2728(67)90032-1
Dziubinska, H. (2003). Ways of signal transmission and physiological role of electrical potential in plants. Acta Soc. Bot. Pol. 72, 309–318. doi: 10.5586/asbp.2003.040
Dziubinska, H., Filek, M., Koscielniak, J., and Trebacz, K. (2003). Variation and action potentials evoked by thermal stimuli accompany enhancement of ethylene emission in distant non-stimulated leaves of Vicia faba minor seedlings. J. Plant Physiol. 160, 1203–1210. doi: 10.1078/0176-1617-00914
Dziubinska, H., Trêbacz, K., and Zawadzki, T. (1989). The effect of excitation on the rate of respiration in the liverwort Conocephalum conicum. Physiol. Plant. 75, 417–423. doi: 10.1111/j.1399-3054.1989.tb04648.x
Felle, H. H., and Zimmermann, M. R. (2007). Systemic signaling in barley through action potentials. Planta 226, 203–214. doi: 10.1007/s00425-006-0458-y
Filek, M., and Kościelniak, J. (1997). The effect of wounding the roots by high temperature on the respiration rate of the shoot and propagation of electric signal in horse bean seedlings (Vicia faba L. minor). Plant Sci. 123, 39–46. doi: 10.1016/S0168-9452(96)04567-0
Fisahn, J., Herde, O., Willmitzer, L., and Peña-Cortés, H. (2004). Analysis of the transient increase in cytosolic Ca2+ during the action potential of higher plants with high temporal resolution: requirement of Ca2+ transients for induction of jasmonic acid biosynthesis and PINII gene expression. Plant Cell Physiol. 45, 456–459. doi: 10.1093/pcp/pch054
Fromm, J., and Lautner, S. (2007). Electrical signals and their physiological significance in plants. Plant Cell Environ. 30, 249–257. doi: 10.1111/j.1365-3040.2006.01614.x
Gallé, A., Lautner, S., Flexas, J., and Fromm, J. (2015). Environmental stimuli and physiological responses: the current view on electrical signaling. Environ. Exp. Bot. 114, 15–21. doi: 10.1016/j.envexpbot.2014.06.013
Gallé, A., Lautner, S., Flexas, J., Ribas-Carbo, M., Hanson, D., Roesgen, J., et al. (2013). Photosynthetic responses of soybean (Glycine max L.) to heat-induced electrical signalling are predominantly governed by modifications of mesophyll conductance for CO2. Plant Cell Environ. 36, 542–552. doi: 10.1111/j.1365-3040.2012.02594.x
García-Plazaola, J. I., Esteban, R., Fernández-Marín, B., Kranner, I., and Porcar-Castell, A. (2012). Thermal energy dissipation and xanthophyll cycles beyond the Arabidopsis model. Photosynth. Res. 113, 89–103. doi: 10.1007/s11120-012-9760-7
Goss, R., and Lepetit, B. (2015). Biodiversity of NPQ. J. Plant Physiol. 172, 13–32. doi: 10.1016/j.jplph.2014.03.004
Grams, T. E. E., Koziolek, C., Lautner, S., Matyssek, R., and Fromm, J. (2007). Distinct roles of electric and hydraulic signals on the reaction of leaf gas exchange upon re-irrigation in Zea mays L. Plant Cell Environ. 30, 79–84. doi: 10.1111/j.1365-3040.2006.01607.x
Grams, T. E. E., Lautner, S., Felle, H. H., Matyssek, R., and Fromm, J. (2009). Heat-induced electrical signals affect cytoplasmic and apoplastic pH as well as photosynthesis during propagation through the maize leaf. Plant Cell Environ. 32, 319–326. doi: 10.1111/j.1365-3040.2008.01922.x
Hlaváčková, V., Krchňák, P., Nauš, J., Novák, O., Špundová, M., and Strnad, M. (2006). Electrical and chemical signals involved in short-term systemic photosynthetic responses of tobacco plants to local burning. Planta 225, 235–244. doi: 10.1007/s00425-006-0325-x
Hlavinka, J., Nožková-Hlaváčková, V., Floková, K., Novák, O., and Nauš, J. (2012). Jasmonic acid accumulation and systemic photosynthetic and electrical changes in locally burned wild type tomato, ABA-deficient sitiens mutants and sitiens pre-treated by ABA. Plant Physiol. Biochem. 54, 89–96. doi: 10.1016/j.plaphy.2012.02.014
Horton, P., Ruban, A. V., Rees, D., Pascal, A. A., Noctor, G., and Young, A. J. (1991). Control of the light-harvesting function of chloroplast membranes by aggregation of the LHCII chlorophyll-protein complex. FEBS Lett. 292, 1–4. doi: 10.1016/0014-5793(91)80819-O
Horton, P., Wentworth, M., and Ruban, A. (2005). Control of the light harvesting function of chloroplast membranes: the LHCII-aggregation model for non-photochemical quenching. FEBS Lett. 579, 4201–4206. doi: 10.1016/j.febslet.2005.07.003
Ivanov, B. N., Sacksteder, C. A., Kramer, D. M., and Edwards, G. E. (2001). Light-induced ascorbate-dependent electron transport and membrane energization in chloroplasts of bundle sheath cells of the C4 plant maize. Arch. Biochem. Biophys. 385, 145–153. doi: 10.1006/abbi.2000.2156
Julien, J. L., Desbiez, M. O., de Jaegher, G., and Frachisse, J. M. (1991). Characteristics of the wave of depolarization induced by wounding in Bidens pilosa L. J. Exp. Bot. 42, 131–137. doi: 10.1093/jxb/42.1.131
Kalaji, H. M., Goltsev, V., Bosa, K., Allakhverdiev, S. I., Strasser, R. J., and Govindjee. (2012). Experimental in vivo measurements of light emission in plants: a perspective dedicated to David Walker. Photosynth. Res. 114, 69–96. doi: 10.1007/s11120-012-9780-3
Kalaji, H. M., Schansker, G., Ladle, R. J., Goltsev, V., Bosa, K., Allakhverdiev, S. I., et al. (2014). Frequently asked questions about in vivo chlorophyll fluorescence: practical issues. Photosynth. Res. 122, 121–158. doi: 10.1007/s11120-014-0024-6
Katicheva, L., Sukhov, V., Akinchits, E., and Vodeneev, V. (2014). Ionic nature of burn-induced variation potential in wheat leaves. Plant Cell Physiol. 55, 1511–1519. doi: 10.1093/pcp/pcu082
Klughammer, C., and Schreiber, U. (2008). Saturation pulse method for assessment of energy conversion in PS I. PAM Appl. Notes. 1, 11–14.
Klughammer, C., Siebke, K., and Schreiber, U. (2013). Continuous ECS-indicated recording of the proton-motive charge flux in leaves. Photosynth. Res. 117, 471–487. doi: 10.1007/s11120-013-9884-4
Kramer, D. M., and Crofts, A. R. (1989). Activation of the chloroplast ATPase measured by the electrochromic change in leaves of intact plants. Biochim. Biophys. Acta 976, 28–41. doi: 10.1016/S0005-2728(89)80186-0
Kramer, D. M., Sacksteder, C. A., and Cruz, J. A. (1999). How acidic is the lumen? Photosynth. Res. 60, 151–163. doi: 10.1023/A:1006212014787
Krol, E., Dziubińska, H., and Trebacz, K. (2004). Low-temperature-induced transmembrane potential changes in mesophyll cells of Arabidopsis thaliana, Helianthus annuus and Vicia faba. Physiol. Plant. 120, 265–270.
Krupenina, N. A., and Bulychev, A. A. (2007). Action potential in a plant cell lowers the light requirement for non-photochemical energy-dependent quenching of chlorophyll fluorescence. Biochim. Biophys. Acta. 1767, 781–788. doi: 10.1016/j.bbabio.2007.01.004
Malone, M. (1994). Wound-induced hydraulic signals and stimulus transmission in Mimosa pudica L. New Phytol. 128, 49–56. doi: 10.1111/j.1469-8137.1994.tb03985.x
Mancuso, S. (1999). Hydraulic and electrical transmission of wound-induced signals in Vitis vinifera. Aust. J. Plant Physiol. 26, 55–61. doi: 10.1071/PP98098
Maxwell, K., and Johnson, G. N. (2000). Chlorophyll fluorescence – a practical guide. J. Exp. Bot. 51, 659–668. doi: 10.1093/jexbot/51.345.659
Morita, S., Itoh, S., and Nishimura, M. (1982). Correlation between the activity of membrane-bound ATPase and the decay rate of flash-induced 515-nm absorbance change in chloroplasts in intact leaves, assayed by means of rapid isolation of chloroplasts. Biochim. Biophys. Acta 679, 125–130. doi: 10.1016/0005-2728(82)90263-8
Müller, P., Li, X.-P., and Niyogi, K. K. (2001). Non-photochemical quenching. A response to excess light energy. Plant Physiol. 125, 1558–1566.
Murakami, S., and Packer, L. (1970). Protonation and chloroplast membrane structure. J. Cell Biol. 47, 332–351. doi: 10.1083/jcb.47.2.332
Pavlovič, A., Slováková, L., Pandolfi, C., and Mancuso, S. (2011). On the mechanism underlying photosynthetic limitation upon trigger hair irritation in the carnivorous plant Venus flytrap (Dionaea muscipula Ellis). J. Exp. Bot. 62, 1991–2000. doi: 10.1093/jxb/erq404
Peters, J. S., and Berkowitz, G. A. (1991). Studies on the system regulating proton movement across the chloroplast envelope. Effects of ATPase inhibitors, Mg2+, and an amine anesthetic on stromal pH and photosynthesis. Plant Physiol. 95, 1229–1236.
Retivin, V. G., Opritov, V. A., and Fedulina, S. B. (1997). Generation of action potential induces preadaptation of Cucurbita pepo L. stem tissues to freezing injury. Russ. J. Plant Physiol. 44, 432–442.
Retivin, V. G., Opritov, V. A., Lobov, S. A., Tarakanov, S. A., and Khudyakov, V. A. (1999). Changes in the resistance of photosynthesizing cotyledon cells of pumpkin seedlings to cooling and heating, as induced by the stimulation of the root system with KCl solution. Russ. J. Plant Physiol. 46, 689–696.
Ruban, A. V., Pascal, A. A., Robert, B., and Horton, P. (2002). Activation of zeaxanthin is an obligatory event in the regulation of photosynthetic light harvesting. J. Biol. Chem. 277, 7785–7789. doi: 10.1074/jbc.M110693200
Ruban, A. V., Young, A. J., and Horton, P. (1993). Induction of nonphotochemical energy dissipation and absorbance changes in leaves. Evidence for changes in the state of the light-harvesting system of photosystem II in vivo. Plant Physiol. 102, 741–750.
Sacksteder, C. A., Kanazawa, A., Jacoby, M. E., and Kramer, D. M. (2000). The proton to electron stoichiometry of steady-state photosynthesis in living plants: a proton-pumping Q cycle is continuously engaged. Proc. Natl. Acad. Sci. U.S.A. 97, 14283–14288. doi: 10.1073/pnas.97.26.14283
Schreiber, U., and Klughammer, C. (2008). New accessory for the DUAL-PAM-100: The P515/535 module and examples of its application. PAM Appl. Notes. 1, 1–10.
Sherstneva, O. N., Surova, L. M., Vodeneev, V. A., Plotnikova, Yu. I, Bushueva, A. V., and Sukhov, V. S. (2016). The role of the intra- and extracellular protons in the photosynthetic response induced by the variation potential in pea seedlings. Biochemistry (Mos). 10, 60–67. doi: 10.1134/S1990747815050116
Sherstneva, O. N., Vodeneev, V. A., Katicheva, L. A., Surova, L. M., and Sukhov, V. S. (2015). Participation of intracellular and extracellular pH changes in photosynthetic response development induced by variation potential in pumpkin seedlings. Biochemistry (Moscow). 80, 776–784. doi: 10.1134/S0006297915060139
Song, C.-P., Guo, Y., Qiu, Q., Lambert, G., Galbraith, D. W., Jagendorf, A., et al. (2004). A probable Na+ (K+) H+ exchanger on the chloroplast envelope functions in pH homeostasis and chloroplast development in Arabidopsis thaliana. Proc. Natl. Acad. Sci. U.S.A. 101, 10211–10216. doi: 10.1073/pnas.0403709101
Stahlberg, R., and Cosgrove, D. J. (1996). Induction and ionic basis of slow wave potentials in seedlings of Pisum sativum L. Planta 200, 416–425. doi: 10.1007/BF00231397
Stahlberg, R., and Cosgrove, D. J. (1997). The propagation of slow wave potentials in pea epicotyls. Plant Physiol. 113, 209–217.
Stanković, B., and Davies, E. (1996). Both action potentials and variation potentials induce proteinase inhibitor gene expression in tomato. FEBS Lett. 390, 275–279. doi: 10.1016/0014-5793(96)00672-2
Sukhov, V. (2016). Electrical signals as mechanism of photosynthesis regulation in plants. Photosynth. Res. doi: 10.1007/s11120-016-0270-x [Epub ahead of print].
Sukhov, V., Akinchits, E., Katicheva, L., and Vodeneev, V. (2013). Simulation of variation potential in higher plant cells. J. Membr. Biol. 246, 287–296. doi: 10.1007/s00232-013-9529-8
Sukhov, V., Nerush, V., Orlova, L., and Vodeneev, V. (2011). Simulation of action potential propagation in plants. J. Theor. Biol. 291, 47–55. doi: 10.1016/j.jtbi.2011.09.019
Sukhov, V., Orlova, L., Mysyagin, S., Sinitsina, J., and Vodeneev, V. (2012). Analysis of the photosynthetic response induced by variation potential in geranium. Planta 235, 703–712. doi: 10.1007/s00425-011-1529-2
Sukhov, V., Sherstneva, O., Surova, L., Katicheva, L., and Vodeneev, V. (2014a). Proton cellular influx as a probable mechanism of variation potential influence on photosynthesis in pea. Plant Cell Environ. 37, 2532–2541. doi: 10.1111/pce.12321
Sukhov, V., Surova, L., Sherstneva, O., Bushueva, A., and Vodeneev, V. (2015a). Variation potential induces decreased PSI damage and increased PSII damage under high external temperatures in pea. Funct. Plant Biol. 42, 727–736. doi: 10.1071/FP15052
Sukhov, V., Surova, L., Sherstneva, O., Katicheva, L., and Vodeneev, V. (2015b). Variation potential influence on photosynthetic cyclic electron flow in pea. Front. Plant Sci. 5:766. doi: 10.3389/fpls.2014.00766
Sukhov, V., Surova, L., Sherstneva, O., and Vodeneev, V. (2014b). Influence of variation potential on resistance of the photosynthetic machinery to heating in pea. Physiol. Plant. 152, 773–783. doi: 10.1111/ppl.12208
Surova, L., Sherstneva, O., Vodeneev, V., and Sukhov, V. (2016). Variation potential propagation decreases heat-related damage of pea photosystem I by 2 different pathways. Plant. Signal. Behav. 11, e1145334. doi: 10.1080/15592324.2016.1145334
Tikhonov, A. N. (2013). pH-dependent regulation of electron transport and ATP synthesis in chloroplasts. Photosynth. Res. 116, 511–534. doi: 10.1007/s11120-013-9845-y
Tikhonov, A. N. (2014). The cytochrome b6f complex at the crossroad of photosynthetic electron transport pathways. Plant Physiol. Biochem. 81, 163–183. doi: 10.1016/j.plaphy.2013.12.011
Vodeneev, V., Akinchits, E., and Sukhov, V. (2015). Variation potential in higher plants: mechanisms of generation and propagation. Plant. Signal. Behav. 10:e1057365. doi: 10.1080/15592324.2015.1057365
Vodeneev, V., Orlova, A., Morozova, E., Orlova, L., Akinchits, E., Orlova, O., et al. (2012). The mechanism of propagation of variation potentials in wheat leaves. J. Plant Physiol. 169, 949–954. doi: 10.1016/j.jplph.2012.02.013
Vodeneev, V. A., Akinchits, E. K., Orlova, L. A., and Sukhov, V. S. (2011). The role of Ca2+, H+, and Cl- ions in generation of variation potential in pumpkin plants. Russ. J. Plant Physiol. 58, 974–981.
von Caemmerer, S., and Farquhar, G. D. (1981). Some relationships between the biochemistry of photosynthesis and the gas exchange of leaves. Planta 153, 376–387. doi: 10.1007/BF00384257
Vredenberg, W., and Pavlovič, A. (2013). Chlorophyll a fluorescence induction (Kautsky curve) in a Venus flytrap (Dionaea muscipula) leaf after mechanical trigger hair irritation. J. Plant Physiol. 170, 242–250. doi: 10.1016/j.jplph.2012.09.009
Wang, C., Yamamoto, H., and Shikanai, T. (2015). Role of cyclic electron transport around photosystem I in regulating proton motive force. Biochim. Biophys. Acta 1847, 931–938. doi: 10.1016/j.bbabio.2014.11.013
Keywords: electrochromic pigment absorbance shifts, H+-ATP synthase, light scattering, photosynthesis, proton motive force, variation potential
Citation: Sukhov V, Surova L, Morozova E, Sherstneva O and Vodeneev V (2016) Changes in H+-ATP Synthase Activity, Proton Electrochemical Gradient, and pH in Pea Chloroplast Can Be Connected with Variation Potential. Front. Plant Sci. 7:1092. doi: 10.3389/fpls.2016.01092
Received: 17 May 2016; Accepted: 11 July 2016;
Published: 22 July 2016.
Edited by:
Sergey Shabala, University of Tasmania, AustraliaReviewed by:
Lars Hendrik Wegner, Karlsruhe Institute of Technology, GermanyCopyright © 2016 Sukhov, Surova, Morozova, Sherstneva and Vodeneev. This is an open-access article distributed under the terms of the Creative Commons Attribution License (CC BY). The use, distribution or reproduction in other forums is permitted, provided the original author(s) or licensor are credited and that the original publication in this journal is cited, in accordance with accepted academic practice. No use, distribution or reproduction is permitted which does not comply with these terms.
*Correspondence: Vladimir Sukhov, dnNzdWhAbWFpbC5ydQ==
Disclaimer: All claims expressed in this article are solely those of the authors and do not necessarily represent those of their affiliated organizations, or those of the publisher, the editors and the reviewers. Any product that may be evaluated in this article or claim that may be made by its manufacturer is not guaranteed or endorsed by the publisher.
Research integrity at Frontiers
Learn more about the work of our research integrity team to safeguard the quality of each article we publish.