- 1Department of Plant Sciences, University of California, Davis, Davis, CA, USA
- 2Group of Genetics, Breeding and Biochemistry of Brassicas, Department of Plant Genetics, Misión Biológica de Galicia, Spanish Council for Scientific Research, Pontevedra, Spain
- 3DynaMo Center, University of Copenhagen, Copenhagen, Denmark
A key limitation in modern biology is the ability to rapidly identify genes underlying newly identified complex phenotypes. Genome wide association studies (GWAS) have become an increasingly important approach for dissecting natural variation by associating phenotypes with genotypes at a genome wide level. Recent work is showing that the Arabidopsis thaliana defense metabolite, allyl glucosinolate (GSL), may provide direct feedback regulation, linking defense metabolism outputs to the growth, and defense responses of the plant. However, there is still a need to identify genes that underlie this process. To start developing a deeper understanding of the mechanism(s) that modulate the ability of exogenous allyl GSL to alter growth and defense, we measured changes in plant biomass and defense metabolites in a collection of natural 96 A. thaliana accessions fed with 50 μM of allyl GSL. Exogenous allyl GSL was introduced exclusively to the roots and the compound transported to the leaf leading to a wide range of heritable effects upon plant biomass and endogenous GSL accumulation. Using natural variation we conducted GWAS to identify a number of new genes which potentially control allyl responses in various plant processes. This is one of the first instances in which this approach has been successfully utilized to begin dissecting a novel phenotype to the underlying molecular/polygenic basis.
Introduction
Exposure of plants to biotic and abiotic stress induces a disruption in plant metabolism implying physiological costs, and thus leading to a reduction in fitness and ultimately in productivity (Karban and Baldwin, 1997; Baldwin, 1998; Mauricio, 1998; Cipollini et al., 2003; Paul-Victor et al., 2010; Züst et al., 2011). In the course of evolution, plants have evolved a myriad of defense mechanisms, which include enhanced production of secondary metabolites such as phenolics, terpenoids, alkaloids, and glucosinolates (GSL) allowing them to adapt and survive stressful events (Ramakrishna and Ravishankar, 2011; War et al., 2012). GSLs are sulfur-containing secondary metabolites that are the most important groups of Brassicaceae metabolites derived from amino acid biosynthesis. Like other secondary metabolites, GSL are not directly involved in providing energy or structural components key for a plant's growth and development but they are essential for the plants survival through ecological interactions with the environment. GSLs are key factors controlling plant resistance against a broad suite of biotic attackers in Arabidopsis and other Brassicas (Lambrix et al., 2001; Kliebenstein et al., 2002; Beekwilder et al., 2008; Hansen et al., 2008; Fan et al., 2011; Bednarek, 2012).
Over the past decades, research has identified nearly the complete catalog of genes and enzymatic steps within the GSL biosynthetic pathways, including the identification of transcription factors (TFs) regulating the aliphatic GSL pathway, allowing for detailed studies on synthesis and regulation of these compounds (Wittstock and Halkier, 2002; Grubb and Abel, 2006; Halkier and Gershenzon, 2006; Gigolashvili et al., 2007, 2008; Hirai et al., 2007; Sønderby et al., 2007, 2010; Schweizer et al., 2013). From these studies, a model was developed that followed the standard hierarchical regulatory architecture for plant defenses, in which biotic attackers are perceived and signals transmitted via the JA-ILE controlled MYC2/3/4 TFs to modulate the expression of the aliphatic GSL pathway in conjunction with the MYB28/29/76 TFs (Gigolashvili et al., 2007, 2008; Hirai et al., 2007; Sønderby et al., 2007, 2010; Schweizer et al., 2013). Recently, this model has been expanded to include a wider array of TFs that interact with the pathway, showing that signal integration can occur at the promoter level of the pathway and not solely rely on integration prior to JA-ILE (Li et al., 2014). Thus, there is a complex suite of external stimuli that can modulate the expression of the pathway.
Challenging the hierarchical model where the defense is solely an output of a regulatory network is new evidence suggesting that GSL metabolites and genes can have a regulatory influence on itself and other pathways. The accumulation of specific GSL have been shown to impact plant growth in a manner that was occasionally considered to be solely caused by the metabolic cost of the GSLs production (Delarue et al., 1998; Barlier et al., 2000; Hansen et al., 2001; Chen et al., 2003; Mikkelsen et al., 2004). However, more recent work suggests that these changes in growth linked to GSL accumulation are more likely the consequence of regulatory cross-talk between the GSL pathway and hormone metabolism (Züst et al., 2011). The introduction of a functional AOP2, a biosynthetic enzyme in the aliphatic GSL pathway, into a naturally occurring knockout genotype leads to alterations in flowering, JA-ILE mediated defense signaling and oscillatory behavior of the circadian clock (Wentzell et al., 2007; Kerwin et al., 2011; Burow et al., 2015). While these papers suggested that at least some of these effects are caused by the AOP2 RNA, additional research showed that a GSL metabolite produced by AOP2 enzyme, allyl GSL (also known as 2-propenyl GSL or sinigrin), function as a signal that alters plant biomass and metabolism in Arabidopsis (Francisco et al., 2016). Similarly, studies have shown that a product resulting from the indole GSL pathway can regulate the production of callose in response to pathogen attack (Bednarek et al., 2009; Clay et al., 2009). These studies showed that a specific GSL metabolite may provide potential regulatory information to influence the general behavior of the plant. However, there is still a need to test how ally GSL modulates these processes and to identify the genes and mechanisms that may facilitate this.
Effective developing methodology to elucidate genes underlying complex traits is the use of natural variation through genome wide association mapping studies (GWAS). GWAS combines phenotype and single-nucleotide polymorphism (SNP) data from natural populations to study the genetic basis of heritable phenotypes, providing valuable information for gene hunting, understanding of biological processes, and plant breeding (Borevitz et al., 2007; Atwell et al., 2010; Chan et al., 2010a, 2011; Brachi et al., 2015; Corwin et al., 2016). The most extensive use of GWAS in Arabidopsis has been testing of well-studied traits such as flowering time variation or disease resistance (phenotypes controlled by single genes with very large effects) where strong phenotype-SNP associations have been found for candidate genes identified a priori from molecular genetic studies (Atwell et al., 2010). The use of GWAS with previously studied polygenic traits allowed the identification of both previously known genes and numerable validatable candidate genes (Borevitz et al., 2007; Chan et al., 2011; Filiault and Maloof, 2012; Corwin et al., 2016). While this suggests that GWAS may be an efficient way to uncover candidate genes for novel phenotypes that have no previous mechanistic information, there is relatively little precedent for this use of GWAS.
To start dissecting the mechanisms behind exogenous allyl GSL induced responses we measured changes in plant biomass and defense metabolites in a collection of natural 96 Arabidopsis thaliana accessions fed with 50 μM of allyl GSL. Exogenous GSL was introduced exclusively to the roots and the compound was transported up to the leaf and caused a wide range of heritable effects upon plant biomass and endogenous GSL accumulation. Using natural variation we conducted GWAS to identify and validate a number of new genes which potentially control allyl signaling feedback inhibition. This identified eight genes that can influence the link between allyl GSL and either biomass accumulation or defense chemistry. These genes include known genes in different pathways and completely unstudied genes. This is one of the first instances in which this approach has been successfully utilized to dissect a novel phenotype to the underlying molecular/polygenic basis.
Material and Methods
Plant Material and Exogenous Allyl GSL Feeding Experiment
A set of 96 natural A. thaliana accessions was analyzed (Nordborg et al., 2002, 2005; Borevitz et al., 2007; Atwell et al., 2010; Chan et al., 2010a,b, 2011; Francisco et al., 2016). Seeds were surface-sterilized (1-min, 70% ethanol soaking followed by a 20-min, 50% sodium hypochlorite), rinsed (five times) in sterile, distilled water. They were then placed on petri dishes containing half-strength Murashige and Skoog (MS) salt medium (CAISSON, MSP01-1LT) adjusted to pH 5.8, containing 0.8% agar, and 1% sucrose concentration (control). To study the effect of exogenous allyl GSL on plant biomass and metabolite content, 0.22 μm filter sterilized allyl GSL 100 mM stock solution (Sigma S1647-1G) was added to the autoclaved MS (at 55⋅C) to a final concentration of 50 μM (treatment). Seeds were placed in 36 grid square 100 × 15 mm plates with 50 mL of medium. Five plants per accession were grown in a randomized partial block design (one seed per grid square). Seeds were planted on control (MS) and allyl-containing MS (MS + Allyl) to provide five measurements per accession per treatment. After planting on media, plates were stratified for 3 days in the dark at 4⋅C to break dormancy. Plates were then transferred to a growth chamber under long-day conditions (16 h light at 100–120 μEi, 20⋅C). Any seedlings with leaf contact to the agar were removed from the analysis to ensure that root-to-shoot transport had occurred. At 15 days post germination, the rosette of each seedling was harvested from the plates, weighed to record the plant fresh weight (fw), then placed into a 96-deep well tube containing 90% methanol for GSL extraction and analyzed for GSL content as described below.
Analysis of GSL Content
GSL of excised shoots were measured using a previously described high-throughput analytical system (Kliebenstein et al., 2001a, b, c). Briefly, rosettes of all seedlings were individually removed from plates with forceps, weighed and placed in a single well of 96-well microliter plate containing 400 μL of 90% methanol and one 3.8 mm stainless steel ball-bearing. Tissues were homogenized for 3 min in a paint shaker, centrifuged, and the supernatants transferred to a 96-well filter plate with 50 μL of DEAE sephadex and washed once with water. The sephadex-bound GSL were eluted by overnight, room temperature incubation with 110 μL of sulfatase. Individual desulfo-GSL within each sample was separated and detected by HPLC-DAD, identified, and quantified by comparison to purified standards. The GSL traits are reported as μmol g of fw of each plant. All seedlings were measured individually and GSL abundance was normalized to the fresh weight. In addition to the content of individual GSL, we developed a series of summation and ratio traits based on prior knowledge of the GSL pathways (Table S1; Kliebenstein, 2007; Wentzell et al., 2007).
Genome Wide Association Mapping
We obtained the least-square means for plant biomass and all GSL traits in all the accessions for both the treated and untreated seedlings. We used these values for GWA using a ridge regression approach that models all polymorphisms in a single model as random effects to predict the H2 model-corrected genotypic accessions means for each phenotype (Shen et al., 2013). From this model, the heteroscedastic effects (HEM) were extracted for each polymorphism. Since determining the degrees of freedom for random variables is difficult, a significant effect threshold was estimated by permuting the phenotypic means across the accession backgrounds 1000 times and taking the 99th quantile. Individual genes were considered associated with the phenotype if they had at least 2 significant SNPs in their coding region, similar to the method used in Chan et al. (2011). For the GSL traits, we focused only on the traits that were measurable in all accessions to maximize the information from the accessions and to minimize the signal from the natural variation controlling the GSL profile.
Single Gene Validation
To validate the ability of specific genes to influence the response to exogenous allyl application, we measured biomass and GSL content of 17 T-DNA insertion lines from 13 candidate genes (Table 1; Haughn et al., 1991; Kliebenstein et al., 2001a; Hansen et al., 2007; Sønderby et al., 2007, 2010; Li et al., 2008). For these analyses, 10 plants per T-DNA line were grown in a randomized partial block design and the entire experiment was performed four times using the same design providing 40 measurements per genotype per treatment.
Statistical Analyses
All the relative differences for each trait were calculated as: [MS stands for MS media with (MS + allyl) or without (MS) exogenous allyl]. To test how the plant biomass and GSL responses to allyl treatment interact with natural variation, the ANOVA utilized accession and treatment (MS and MS + Allyl) as factors and experiment as a random variable. Plate was tested for significance as a random effect in a mixed model but not found to significantly alter the results and hence dropped from the model. The least-square means of each plant biomass and GSL phenotype per each accession within each treatment were obtained using this model. Multiple comparisons were made post-hoc using Tukey's t-test with P ≤ 0.05 within the model. Nested ANOVA was also utilized to test for the effect of exogenous allyl GSL on plant biomass and GSL content of different T-DNA insertion lines. Each mutant was tested in an individual ANOVA against the Col-0 (WT) genotype. We calculated estimates of broad-sense heritability (H) for plant biomass and allyl accumulation as H2 = σ/σ, where σ was the estimated trait genetic variance among different genotypes in this mapping population of 96 Arabidopsis accessions and σ was the total phenotypic variance for a trait. All statistical analyses were conducted using SAS.
Results
Natural Variation in Arabidopsis Biomass Responses to Allyl GSL
To begin identifying genes and the potential mechanism(s) by which allyl GSL can affect biomass changes in Arabidopsis, we measured the response of a population of 96 natural Arabidopsis accessions to external allyl GSL application. All accessions were planted in quintuplicate using a random split-block design. This population was previously analyzed to assess how endogenous GSL genetic variation influences the link between allyl GSL and biomass accumulation but the genetic architecture of these traits have not yet been described (Francisco et al., 2016). ANOVA showed that plant biomass was highly heritable (H2 = 0.88) and that natural Arabidopsis accessions have significant variation for the effect of allyl GSL upon seedling plant biomass (Table 2). The distribution of plant biomass across the accessions showed that, in general, exogenous allyl GSL application decreased plant biomass across the population but individual accessions showed positive responses (Figure 1). Thus, there is genetic variation for the plant biomass response to exogenous allyl GSL application in A. thaliana. Further, the presence of accessions with positive and negative responses to allyl GSL suggests that there is likely more than one mechanism controlling the response.
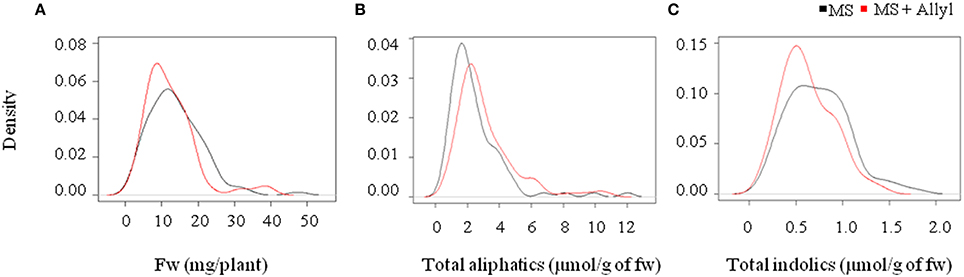
Figure 1. Natural variation in Arabidopsis biomass and GSL accumulation in response to exogenous allyl GSL. (A) Kernel density plots showing the distribution of fw (mg/plant), (B) total aliphatic GSLs, and (C) total indolic GSLs (μmol/g of fw) from 96 natural Arabidopsis accessions grown in MS (black line) and MS + Allyl (red line).
To measure the genetic variation in how endogenous GSL accumulation responds to exogenous allyl GSL application, we measured GSL from the 96 Arabidopsis accessions seedlings fed with allyl GSL and from the control samples (Francisco et al., 2016). These were from the same individual seedlings measured for biomass and all values are adjusted to the seedlings' biomass. This analysis detected 14 aliphatic GSL compounds and three indolic GSL compounds from which we could define an additional 15 descriptive variables to isolate specific biosynthetic processes and generate a total of 32 traits (Wentzell et al., 2007; Chan et al., 2010a). The GSL traits significantly varied between the accessions with 29 of the 32 aliphatic and indolic GSL traits showing a significant interaction of accession by allyl GSL treatment, suggesting that the accessions have differential responses to the treatment as measured by GSL accumulation (Table 3; Figure 2). In general, aliphatic GSL accumulation across the accessions tended to increase after the application of allyl GSL (Figure 1). However, like biomass accumulation, these changes in endogenous aliphatic GSL accumulation showed a wide range of both positive and negative responses across the accessions (Figure 2). Further, the positive effects were larger than could be accounted for by the additive effect of exogenous allyl GSL application. Moreover, other GSL that cannot be synthesized from the allyl GSL, such as but-3-enyl GSL were also affected by the allyl treatment showing that this is not caused by the uptake and conversion of allyl GSL to other structures (Figure 2). In contrast to the aliphatic GSL, total indolic GSL content and specific indolic GSL compounds, tended to be reduced in most accessions while a few specific accessions showed an increase (Figures 1, 2). Again, the presence of accessions showing both positive and negative responses suggests that the response to allyl GSL likely involves a number of pathways.
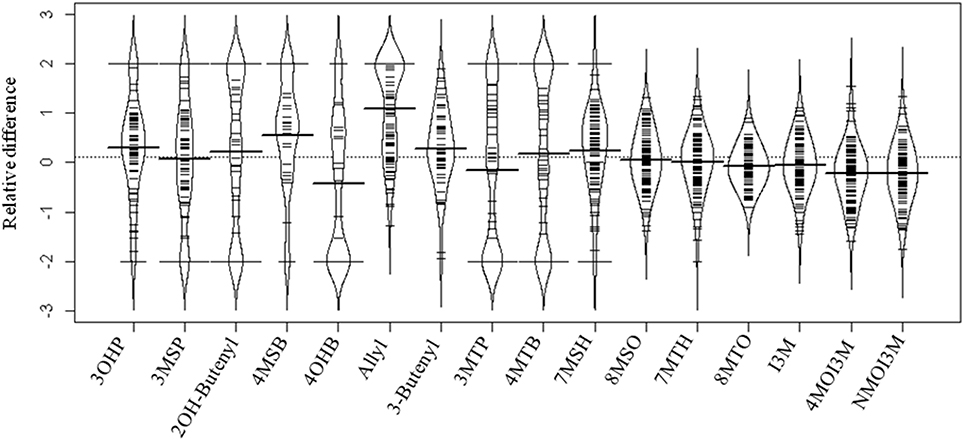
Figure 2. Distribution of the relative difference of individual GSL accumulation in response to allyl GSL treatment across the Arabidopsis accessions. A beanplot is used to show the distribution of the change in GSL accumulation between the treated and untreated samples across the accessions using the abbreviations in Table S1. Relative difference was determined as (GSL treatment − GSL control)/(0.5 × [GSL treatment + GSL control]). The dashed line in the middle of the plot is the overall average of the relative GSL difference between control and allyl treatment across all GSL. The thick black line in the middle of each bean for each compound is the mean response for that specific GSL trait across all the accessions. The black colored curved bean pod surrounding the observations is the theoretical probability density distribution of these observations. The small lines represent individual data points, with the length of the line proportional to the number of observations with that specific value. The relative difference between treatment and control varied across the 96 accessions from −2 to 2 for each GSL compound, depending on whether that GSL was present in the treatment compared with control.
Natural Variation in Arabidopsis Accumulation of Exogenous Allyl GSL
Approximately half of the studied accessions grown do not produce endogenous allyl GSL because they do not contain a functional copy of the required single-copy gene for the AOP2 enzyme (Kliebenstein et al., 2001a). Thus, any accumulated allyl GSL that we measure within these accessions is solely due to the uptake and transport of exogenous allyl allowing us to test if there are differences in the ability to take up and store this GSL from the growth media. The mean accumulation of allyl GSL was 0.34 μmol/g of fw and ranged from 0.11 to 0.86 μmol/g of fw. A three-way ANOVA identified highly significant differences between accessions for the accumulation of exogenous allyl GSL within the seedling leaves (Table 4). Broad-sense heritability of exogenous allyl GSL accumulation was 70% for the accessions that do not produce endogenous allyl GSL. Thus, there is genetic variation for the ability to import, transport and accumulate the exogenous allyl GSL in the leaves of Arabidopsis seedlings.
Genome Wide Association Mapping of Biomass and GSL Responses to Exogenous Allyl GSL within Arabidopsis Accessions
To identify genes within Arabidopsis that may control the biomass or GSL accumulation responses to exogenous allyl GSL treatment, we utilized the mean biomass and GSL accumulation in each accession grown with or without allyl GSL to conduct GWA mapping (Figure 3). For these analysis we employed a ridge-regression model that treats all SNPs as random effects (Shen et al., 2013). Using this ridge-regression model we tested all traits for significance associations across 115,301 SNPs with a MAF > 0.2 that covered 19,352 unique genes. Significance thresholds were determined by measuring the 95th percentile of the randomly generated effects of 1000 permutations of the means among the accessions (Chan et al., 2011; Corwin et al., 2016). This permutation threshold, while conservative, allows us to utilize an empirically derived threshold for significance based on the specific phenotypes distribution. We then applied a filter to these SNP lists to find candidate genes by requiring a gene to be considered as a potential GWA candidate only if it has two or more significant SNPs. This approach has previously been shown to identify genes with a high validation success rate for an array of traits (Chan et al., 2011; Corwin et al., 2016). Using this approach we identified 671 genes significantly associated with biomass accumulation with the majority found uniquely in either the control (203) or allyl treated accessions (435) (Tables S2, S3). Only 33 genes were significant GWA candidates using biomass in the presence and absence of allyl GSL. A survey of these genes by either GO analysis or by co-expression network clustering using ATTED-II (Obayashi et al., 2009), did not identify any obvious enrichment patterns.
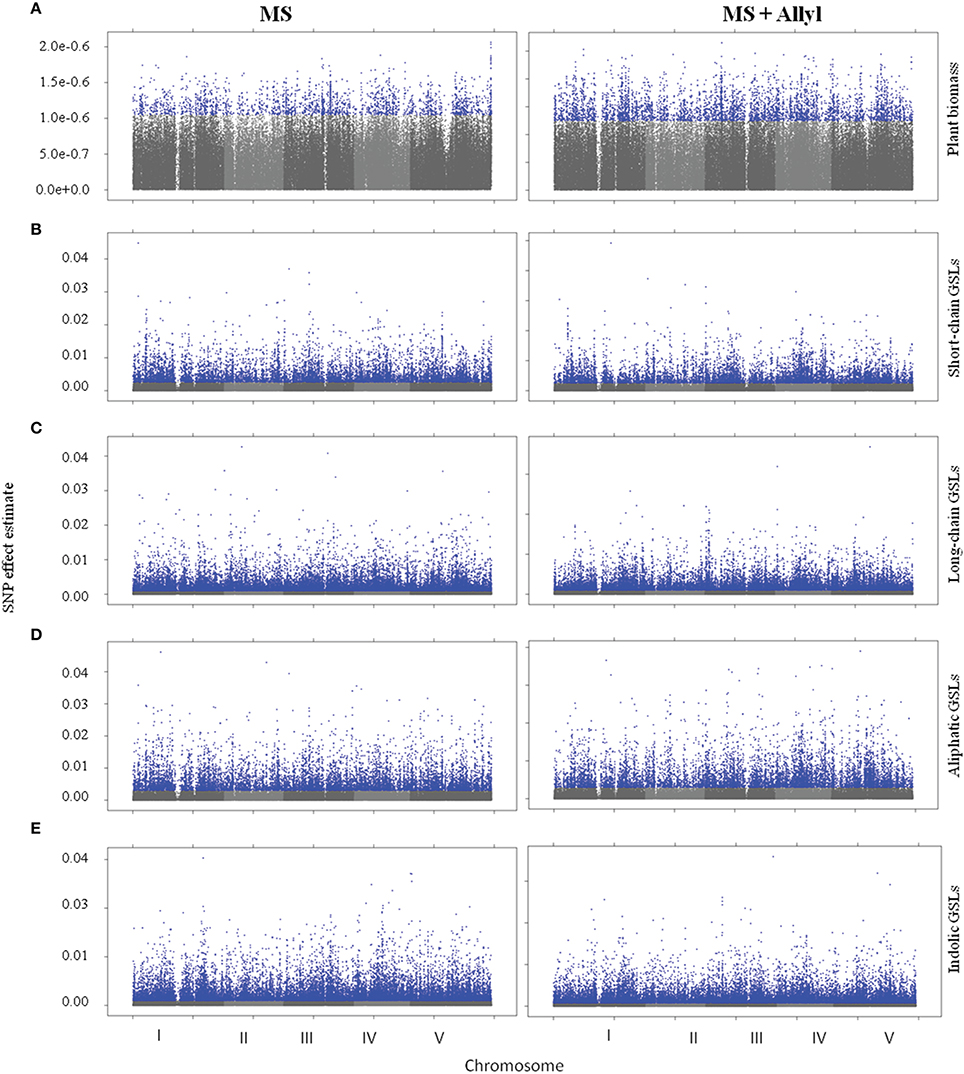
Figure 3. Manhattan plots of GWAS results. Genome wide distribution of the absolute value of the heteroscedastic SNP effects. Shades of gray represent nonsignificant SNP effects. Blue points represent significant SNP effects under control (MS) and allyl treatment (MS + Allyl). (A) Plant Biomass, (B) Short-Chain GSLs, (C) Long-Chain GSLs, (D) Aliphatic GSLs, (E) Indolic GSLs.
To map genes that influence the variation in endogenous GSL responses to exogenous allyl GSL, we focused on five GSL phenotypes that are present in all accessions, the total amount of long-chain GSLs, total short-chain GSLs, total aliphatic GSLs, total indolic GSLs and the sum of all GSLs (Kliebenstein et al., 2001b,c, 2002; Wentzell et al., 2007; Chan et al., 2010a, 2011). The accumulation of individual GSLs is heavily dependent on presence/absence variation of known GSL enzyme loci leading to presence/absence variation in these compounds and confounds the GWAS mapping. In contrast, these summation based traits are measurable in all accessions and are largely independent of the known major effect GSL polymorphisms as shown by previous GWAS analysis (Kliebenstein et al., 2001b,c, 2002; Wentzell et al., 2007; Chan et al., 2010a, 2011). This increases our power to identify causal genes both by having data for all accessions and by eliminating major effect polymorphisms that can otherwise hinder the power to identify smaller effect loci (Nordborg and Weigel, 2008). Given the quantitative distribution of GSL responses to exogenous allyl treatment, we expected mainly small to moderate effect loci (Figure 3). GWAS with these traits identified on average 2750 genes significantly associated with any given trait (Tables S2, S3). Of these candidate genes, 36% were typically found with the treated samples, 43% with the control treatment and 21% under both conditions (Figure 4). Interestingly, this contrasts with biomass accumulation where only 5% of the genes were found under both conditions. A survey of these genes by either GO analysis or by co-expression network clustering did not identify any obvious mechanistic patterns.
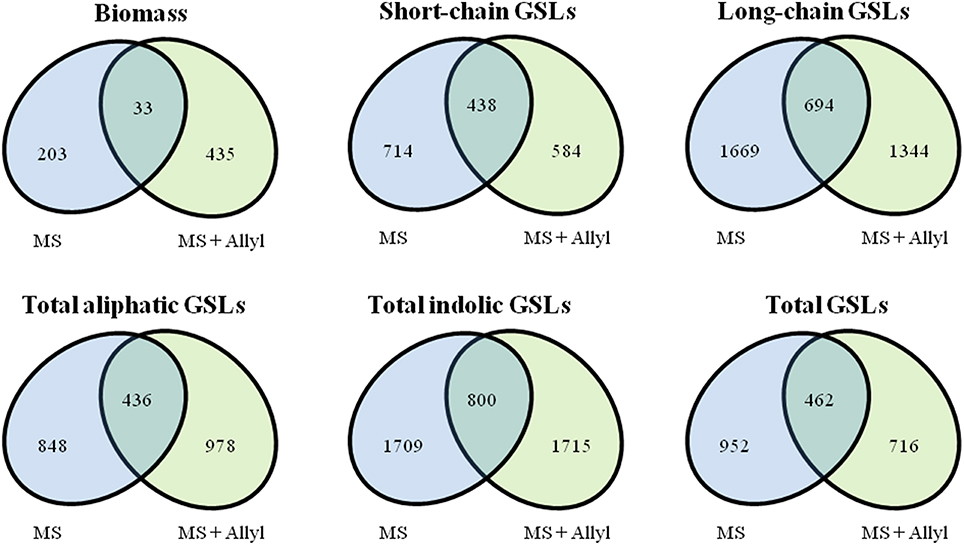
Figure 4. Overlap of significant GWA candidate genes between control (MS) and treated samples with 50 μM of allyl GSL (MS + Allyl GSL). VENN diagram showing common candidate genes identified among the plant biomass, short-chain GSLs, long-chain GSLs, total aliphatic GSLs, total incolic GSLs, and total GSLs traits.
To check if the GWA mapping identified candidate genes were similar for the biomass and GSL traits, we investigated the overlap of GWA candidate genes identified across plant biomass and three GSL traits that summarize the majority of GSL variation (short-chain GSLs, long-chain GSLs and total indolic GSLs) from control and treated samples (Figure 5). This showed that 133 of the identified GWA candidate gene sets from plant biomass overlap with the identified GWA candidate genes from GSL phenotypes in the control samples. The number of overlap candidate genes between plant biomass and GSL traits was 232 for the exogenous allyl treated samples. Only 27 genes overlapped between biomass and GSL accumulation in both the presence and absence of allyl GSL. This suggests that the effect of the majority of the candidate genes we identified for biomass and GSL phenotypes are conditioned by the exogenous allyl treatment.
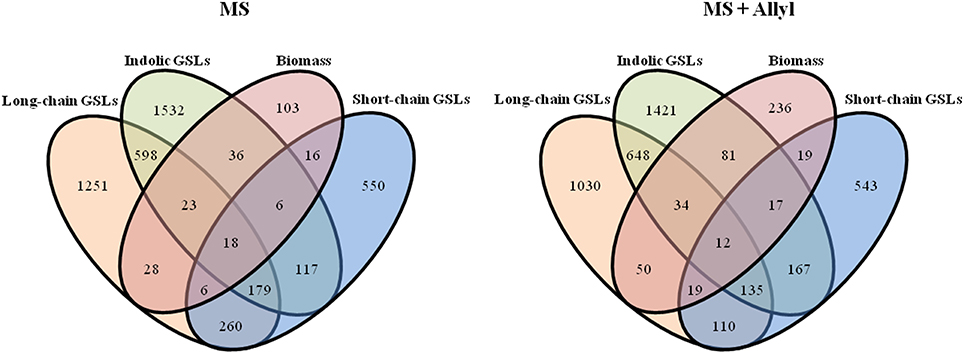
Figure 5. Overlap of significant GWA candidate genes between plant biomass and GSL phenotypes. VENN diagram showing common candidate genes identified among the short-chain GSLs, long-chain GSLs, total incolic GSLs and plant biomass traits studied from control (MS) and treated samples with 50 μM of allyl GSL (MS + Allyl).
Validation of Candidate Genes via T-DNA Insertion Lines
To test if any of the genes identified through GWA mapping may influence the response to exogenous allyl GSL, we filtered the candidate genes that affect plant biomass by removing the genes that were candidates in both conditions. We then further queried the remaining genes to find those whose transcript accumulation is responsive to allyl GSL (Burow et al., 2015). We ranked genes from this list based on fold-change response of their transcripts to allyl GSL. We chose the top 13 most responsive candidate genes and obtained 17 homozygous T-DNA insertion lines (Table 1). This included obtaining two alleles in as many genes as we could given public databases. All of these lines were validated as homozygous and grown concurrently with the wild type (WT) Col-0 in the same growth chamber to obtain seeds to control for maternal environmental effects as much as possible. We then grew all the genotypes in the presence and absence of the exogenous allyl treatment and measured the plant biomass and GSL responses to exogenous allyl GSL. Each genotype within each treatment has a minimum of 40 independent measurements conducted across four experiments using a randomized block design (Table S4).
Using per seedling biomass, we utilized ANOVA based-tests to compare the exogenous allyl GSL response of the different T-DNA lines to WT Col-0 and showed that insertions in a number of genes abolished the Col-0 biomass and/or GSL response to exogenous allyl GSL treatment (Figure 6 and Table S5). For plant biomass, seven of the 13 candidate genes showed a significant interaction with exogenous allyl GSL treatment (P < 0.05) and three were suggestive of an interaction (P < 0.10). In addition, the T-DNA lines for eight of the genes also showed altered responses of endogenous GSL accumulation to exogenous allyl GSL in comparison to the WT Col-0. Interestingly, all of the T-DNA insertions that abolished the biomass response to exogenous allyl GSL also displayed an altered oxidation status of the 4C GSL away from the 4MTB and toward the 4MSB following exogenous GSL application (Figure 6 and Table S5). Thus, we can validate our ability to utilize the GWA mapping approach to identify genes that modulate the response to exogenous allyl GSL.
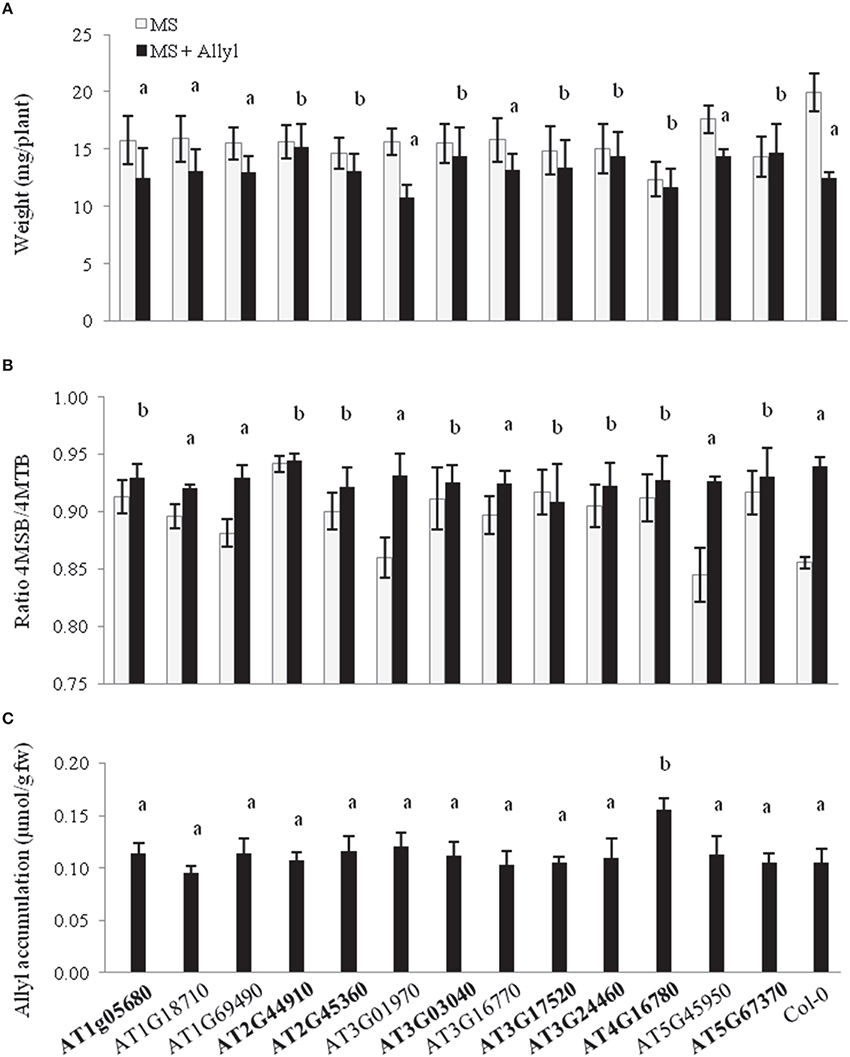
Figure 6. Plant biomass responses and GSL content variation among T-DNA insertion lines of 13 candidate genes treated with allyl GSL. (A) Quantification of 15-day-old fw (mg/plant) seedlings from T-DNA insertion lines of 13 candidate genes and wild-type (Col-0) fed with 50 μM of allyl glucosinolate. (B) Ratio of 4-methylsulfinylbutyl (4MSB)/4-methylthiobutyl (4MTB) calculated as 4MSB/(4MSB + 4MTB). (C) Average allyl GSL accumulation of the evaluated genotypes. The bar chart represents the mean and the standard deviation. Each genotype within each treatment has a minimum of 40 independent measurements conducted across four experiments using a randomized block design. Means with the same letter show if the genotype's response to the treatment was statistically similar to Col-0 (a) or different from Col-0 (b) at P ≤ 0.05 from the two-way ANOVA analysis (Table S5). Gene's in bold have one or more phenotypes with a statistically different response to exogenous allyl treatment in comparison to Col-0. See Table 1 for T-DNA insertion lines details.
We also tested the ability of the T-DNA lines to alter the uptake and accumulation of the exogenous allyl GSL to test if the mutant effects were due to alterations in the metabolism of the exogenous allyl GSL rather than potential signaling effects. This is facilitated by the fact that the Col-0 background for the mutants has no functional AOP2 gene and as such cannot make nor convert the allyl GSL. As such, all measured allyl GSL in these samples had to come from the exogenous application. The level of accumulation of exogenous allyl GSL among the T-DNA lines was statistically identical to WT Col-0, except for AT4G16780 (ATHB2), which accumulated approximately 50% more allyl GSL in the leaves. Interestingly, this line was non-responsive to the exogenous GSL for both plant biomass and other aliphatic GSL traits (Figures 6A,B). This suggests that this increased uptake of allyl GSL was not causing hyper-responsiveness affecting plant biomass and endogenous GSL accumulation within this genotype. Thus, ATHB2 appears to modulate both the accumulation of exogenous GSL and the plant biomass to this exogenous GSL (Figure 6C). Further, these results show that the other genotypes all accumulated the exogenous allyl GSL to a level identical to WT Col-0 and any differences are likely from variation in other mechanisms. Thus, we can use GWA to find genes affecting the accumulation of exogenous allyl GSL and the response to this GSL.
Discussion
A key limitation in modern biology is the ability to rapidly identify the genes underlying newly identified complex phenotypes/traits. GWAS are a promising route for dissecting natural variation by associating phenotypes with genotypes at a genome wide level. These studies exploit the nonrandom coinheritance of genetic variants (linkage disequilibrium) to simultaneously assay hundreds of thousands of markers for an association with any given trait. In contrast to the traditional use of structured mapping populations derived from two parent genomes, GWAS allow a wide sampling of the genotypes present within a species, increasing allelic variants per locus which allow potentially identify a greater proportion of the variable loci contributing to polygenic traits. In the present study, we used GWA mapping to begin to identify genes underlying the newly described trait wherein Arabidopsis modulates its plant biomass in response to a plant secondary metabolite. For that, we fed with exogenous allyl GSL a natural population of 96 Arabidopsis accessions. This population was chosen to minimize population structure and maximize statistical power for GWAS mapping with a moderate population size (Nordborg et al., 2002, 2005; Kim et al., 2007; Zhao et al., 2007; Atwell et al., 2010; Chan et al., 2010a,b, 2011). The application of exogenous allyl GSL caused changes in plant biomass and accumulation of defense metabolites identifying a wide range of genetic diversity across accessions varying between strong positive responses to strong negative responses. Utilizing natural variation of plant biomass, total amount of long-chain GSLs, total short-chain GSLs, total aliphatic GSLs, total indolic GSLs, and the sum of all GSL, we were able to identify genes within Arabidopsis that may control the biomass or GSL accumulation responses to exogenous allyl GSL. From all of the significantly associated genes with each trait, a small percentage was coincident between both conditions (control and allyl treatment; Tables S2, S3). Thus, the majority of identified GWA candidate genes are conditional upon the presence or absence of exogenous allyl GSL application.
A limiting factor for the utility of GWAS has been the preponderance of false-positive and false-negative associations which makes the accurate prediction of biologically valid genotype-phenotype associations very difficult. Integrating GWAS mapping results with additional forms of genome-scale data, such as transcript profiling or proteomics datasets has also been proposed to strengthen support for detected gene-trait associations and reduce the incidence of false-positive associations (Hawkins et al., 2010). We used a set of filters to reduce the number of candidate genes influencing plant biomass under exogenous GSL. First we removed the genes that were candidates in both conditions (control and allyl treatment). Then, the remaining genes were filtered based on their fold-change transcript accumulation is responsive to exogenous allyl GSL application (Burow et al., 2015). We selected 13 genes to test if T-DNA mutants of these genes altered this response (Table 1). Mutants in eight of these genes lead to a diminished or abolished response to the exogenous allyl GSL treatment (Figure 6A). In addition to altering biomass, these T-DNA lines also displayed an altered oxidation status of the 4C GSL (4MSB/4MTB ratio) following exogenous GSL application in comparison to the WT Col-0 (Figure 6B). Negative correlation between plant biomass response to exogenous allyl GSL and the ratio of methylsulfinylalkyl/methylthioalkyl GSL was reported before in Arabidopsis (Francisco et al., 2016). Of these eight genes, three had at least two mutant T-DNA alleles giving them stronger support for being true causal loci. It should be noted however, that all genes have at least two significant SNPs linked to the traits in question showing that there are two or more alleles within the natural accessions also linked to causing the trait variation. As was observed across the 96 Arabidopsis accessions, the mutants typically affected both the biomass and GSL responses, with only one mutant affecting only one or the other.
Potential Mechanisms of Allyl Response
These newly identified genes began to develop a crude model of how allyl GSL may influence biomass. Two of the genes, HB4 (At2g44910) and HB2 (At4g16780), are both homeodomain-leucine zipper II transcription factors that are important for controlling Arabidopsis development (Bou-Torrent et al., 2012; Nomoto et al., 2012; Carabelli et al., 2013; Turchi et al., 2013). HB2 is also linked to altered auxin regulation suggesting that there may be a link between allyl GSL responses and auxin (Bou-Torrent et al., 2012; Nomoto et al., 2012; Carabelli et al., 2013; Turchi et al., 2013). Supporting this is the observation that another gene influencing the response to allyl GSL is At1g05680, UGT74E2, has been shown to alter in planta indole-3-acetic acid metabolism (Grubb et al., 2004, 2014). Further, auxin response networks are highly polymorphic within Arabidopsis (Delker et al., 2010). Interestingly, raphanusanin, a GSL specifically produced by Raphanus sativa, controls hypocotyl bending in response to light by affecting the TIR1 auxin receptor to modulate auxin signaling (Hasegawa et al., 2000; Yamada et al., 2003). Thus, it is possible that there are overlaps in how these two structurally unrelated GSL compounds, allyl, and raphanusanin, may affect plant growth in different lineages.
The remaining GWA candidate genes that we were able to validate as altering responses to allyl GSL are largely unstudied, including an F-box (At3g03040), a putative sphingolipid metabolism gene (At3g24460), a late embryogenesis abundant protein (At3g17520) and two proteins of unknown function At2g45360 (DUF1230) and At5g67370 (DUF1442). Thus, it is likely that these newly identified genes will allow us to identify new mechanisms controlling Arabidopsis plant biomass regulation. Extensive future studies will be required to map out how the defense metabolite allyl GSL can modulate various plant processes. Together, this shows that it is possible to use GWA mapping in plants to begin to identify genes controlling previously unknown traits, such as the ability to respond to endogenous secondary metabolites.
A key future step is to identify how Arabidopsis can detect allyl GSL and convey this information to the regulatory processes that control biomass and defense metabolism. Once these processes are understood, it will be possible to disconnect the potential feedback loop involving allyl GSL and formally test if the dynamic regulatory system becomes destabilized as predicted by theory.
Author Contributions
MF, MB, and DK conceived and designed the experiments. MF, HC, BL, CL, and RK conducted the plant work. MF, BJ, JC, and DK did the statistical analyses. MF and DK interpreted the data and wrote the paper.
Funding
This work was funded by a Marie Curie International Outgoing Fellowship within the 7th European Community Framework Programme (PIOF-GA-2010-275286) to MF, the Spanish Ministry of Economy and Competitiveness through a ‘Juan de la Cierva’ program (IJCI-2014-19653) to MF, the NSF DBI grant 0820580 to DK, the NSF MCB grant 1330337 to DK, the USDA National Institute of Food and Agriculture, Hatch project number CA-D-PLS-7033-H to DK and by the Danish National Research Foundation (DNRF99) grant to DK and MB.
Conflict of Interest Statement
The authors declare that the research was conducted in the absence of any commercial or financial relationships that could be construed as a potential conflict of interest.
Supplementary Material
The Supplementary Material for this article can be found online at: http://journal.frontiersin.org/article/10.3389/fpls.2016.01010
References
Atwell, S., Huang, Y., Vilhjalmsson, B. J., Willems, G., Horton, M., Li, Y., et al. (2010). Genome-wide association study of 107 phenotypes in a common set of Arabidopsis thaliana in-bred lines. Nature 465, 627–631. doi: 10.1038/nature08800
Baldwin, I. T. (1998). Jasmonate-induced responses are costly but benefit plants under attack in native populations. Proc. Natl. Acad. Sci. U.S.A. 95, 8113–8118. doi: 10.1073/pnas.95.14.8113
Barlier, I., Kowalczyk, M., Marchant, A., Ljung, K., Bhalerao, R., Bennett, M., et al. (2000). The SUR2 gene of Arabidopsis thaliana encodes the cytochrome P450CYP83B1, a modulator of auxin homeostasis. Proc. Natl. Acad. Sci. U.S.A. 97, 14819–14824. doi: 10.1073/pnas.260502697
Bednarek, P. (2012). Sulfur-containing secondary metabolites from Arabidopsis thaliana and other brassicaceae with function in plant immunity. Chembiochem 13, 1846–1859. doi: 10.1002/cbic.201200086
Bednarek, P., Piślewska-Bednarek, M., Svatoš, A., Schneider, B., Doubský, J., Mansurova, M., et al. (2009). A glucosinolate metabolism pathway in living plant cells mediates broad-spectrum antifungal defense. Science 323, 101–106. doi: 10.1126/science.1163732
Beekwilder, J., van Leeuwen, W., van Dam, N. M., Bertossi, M., Grandi, V., Mizzi, L., et al. (2008). The impact of the absence of aliphatic glucosinolates on insect herbivory in Arabidopsis. PLoS ONE 3:e2068. doi: 10.1371/journal.pone.0002068
Borevitz, J. O., Hazen, S. P., Michael, T. P., Morris, G. P., Baxter, I. R., Hu, T. T., et al. (2007). Genome-wide patterns of single-feature polymorphism in Arabidopsis thaliana. Proc. Natl. Acad. Sci. U.S.A. 104, 12057–12062. doi: 10.1073/pnas.0705323104
Bou-Torrent, J., Salla-Martret, M., Brandt, R., Musielak, T., Palauqui, J.-C., Martinez-Garcia, J. F., et al. (2012). ATHB4 and HAT3, two class II HD-ZIP transcription factors, control leaf development in Arabidopsis. Plant Signal. Behav. 7, 1382–1387. doi: 10.4161/psb.21824
Brachi, B., Meyer, C. G., Villoutreix, R., Platt, A., Morton, T. C., Roux, F., et al. (2015). Coselected genes determine adaptive variation in herbivore resistance throughout the native range of Arabidopsis thaliana. Proc. Natl. Acad. Sci. U.S.A. 112, 4032–4037. doi: 10.1073/pnas.1421416112
Burow, M., Atwell, S., Francisco, M., Kerwin, R. E., Halkier, B. A., and Kliebenstein, D. J. (2015). The glucosinolate biosynthetic gene AOP2 mediates feed-back regulation of jasmonic acid signaling in Arabidopsis. Mol. Plant 8, 1201–1212. doi: 10.1016/j.molp.2015.03.001
Carabelli, M., Turchi, L., Ruzza, V., Morelli, G., and Ruberti, I. (2013). Homeodomain-Leucine Zipper II family of transcription factors to the limelight: central regulators of plant development. Plant Signal. Behav. 8:e25447. doi: 10.4161/psb.25447
Chan, E. K. F., Rowe, H. C., and Kliebenstein, D. J. (2010a). Understanding the evolution of defense metabolites in Arabidopsis thaliana using genome-wide association mapping. Genetics 185, 991–1007. doi: 10.1534/genetics.109.108522
Chan, E. K., Rowe, H. C., Corwin, J. A., Joseph, B., and Kliebenstein, D. J. (2011). Combining genome-wide association mapping and transcriptional networks to identify novel genes controlling glucosinolates in Arabidopsis thaliana. PLoS Biol. 9:e1001125. doi: 10.1371/journal.pbio.1001125
Chan, E. K. F., Rowe, H. C., Hansen, B. G., and Kliebenstein, D. J. (2010b). The complex genetic architecture of the metabolome. PLoS Genet. 6:e1001198. doi: 10.1371/journal.pgen.1001198
Chen, S. X., Glawischnig, E., Jorgensen, K., Naur, P., Jorgensen, B., Olsen, C. E., et al. (2003). CYP79F1 and CYP79F2 have distinct functions in the biosynthesis of aliphatic glucosinolates in Arabidopsis. Plant J. 33, 923–937. doi: 10.1046/j.1365-313X.2003.01679.x
Cipollini, D., Purrington, C. B., and Bergelson, J. (2003). Costs of induced responses in plants. Basic Appl. Ecol. 4, 79–89. doi: 10.1078/1439-1791-00134
Clay, N. K., Adio, A. M., Denoux, C., Jander, G., and Ausubel, F. M. (2009). Glucosinolate metabolites required for an Arabidopsis innate immune response. Science 323, 95–101. doi: 10.1126/science.1164627
Corwin, J. A., Copeland, D., Feusier, J., Subedy, A., Eshbaugh, R., Palmer, C., et al. (2016). The quantitative basis of the Arabidopsis innate immune system to endemic pathogens depends on pathogen genetics. PLoS Genetics 12:e1005789. doi: 10.1371/journal.pgen.1005789
Delarue, M., Prinsen, E., Van Onckelen, H., Caboche, M., and Bellini, C. (1998). Sur2 mutations of Arabidopsis thaliana define a new locus involved in the control of auxin homeostasis. Plant J. 14, 603–611. doi: 10.1046/j.1365-313X.1998.00163.x
Delker, C., Pöschl, Y., Raschke, A., Ullrich, K., Ettingshausen, S., Hauptmann, V., et al. (2010). Natural variation of transcriptional auxin response networks in Arabidopsis thaliana. Plant Cell 22, 2184–2200. doi: 10.1105/tpc.110.073957
Fan, J., Crooks, C., Creissen, G., Hill, L., Fairhurst, S., Doerner, P., et al. (2011). Pseudomonas sax genes overcome aliphatic isothiocyanate–mediated non-host resistance in Arabidopsis. Science 331, 1185–1188. doi: 10.1126/science.1199707
Filiault, D. L., and Maloof, J. N. (2012). A genome-wide association study identifies variants underlying the Arabidopsis thaliana shade avoidance response. PLoS Genet. 8:e1002589. doi: 10.1371/journal.pgen.1002589
Francisco, M., Joseph, B., Caligagan, H., Li, B., Corwin, J. A., Lin, C., et al. (2016). The defense metabolite, Allyl glucosinolate, modulates Arabidopsis thaliana biomass dependent upon the endogenous glucosinolate pathway. Front. Plant Sci. 7:774. doi: 10.3389/fpls.2016.00774
Gigolashvili, T., Engqvist, M., Yatusevich, R., Muller, C., and Flugge, U. I. (2008). HAG2/MYB76 and HAG3/MYB29 exert a specific and coordinated control on the regulation of aliphatic glucosinolate biosynthesis in Arabidopsis thaliana. New Phytol. 177, 627–642. doi: 10.1111/j.1469-8137.2007.02295.x
Gigolashvili, T., Yatusevich, R., Berger, B., Müller, C., and Flügge, U. I. (2007). The R2R3-MYB transcription factor HAG1/MYB28 is a regulator of methionine-derived glucosinolate biosynthesis in Arabidopsis thaliana. Plant J. 51 247–261. doi: 10.1111/j.1365-313X.2007.03133.x
Grubb, C. D., and Abel, S. (2006). Glucosinolate metabolism and its control. Trends Plant Sci. 11, 89–100. doi: 10.1016/j.tplants.2005.12.006
Grubb, C. D., Zipp, B. J., Kopycki, J., Schubert, M., Quint, M., Lim, E. K., et al. (2014). Comparative analysis of Arabidopsis UGT74 glucosyltransferases reveals a special role of UGT74C1 in glucosinolate biosynthesis. Plant J. 79, 92–105. doi: 10.1111/tpj.12541
Grubb, C. D., Zipp, B. J., Ludwig-Müller, J., Masuno, M. N., Molinski, T. F., and Abel, S. (2004). Arabidopsis glucosyltransferase UGT74B1 functions in glucosinolate biosynthesis and auxin homeostasis. Plant J. 40, 893–908. doi: 10.1111/j.1365-313X.2004.02261.x
Halkier, B. A., and Gershenzon, J. (2006). Biology and biochemistry of glucosinolates. Annu. Rev. Plant Biol. 57, 303–333. doi: 10.1146/annurev.arplant.57.032905.105228
Hansen, B. G., Kerwin, R. E., Ober, J. A., Lambrix, V. M., Mitchell-Olds, T., Gershenzon, J., et al. (2008). A novel 2-oxoacid-dependent dioxygenase involved in the formation of the goiterogenic 2-hydroxybut-3-enyl glucosinolate and generalist insect resistance in Arabidopsis. Plant Physiol. 148, 2096–2108. doi: 10.1104/pp.108.129981
Hansen, B. G., Kliebenstein, D. J., and Halkier, B. A. (2007). Identification of a flavin-monooxygenase as the S-oxygenating enzyme in aliphatic glucosinolate biosynthesis in Arabidopsis. Plant J. 50, 902–910. doi: 10.1111/j.1365-313X.2007.03101.x
Hansen, C. H., Wittstock, U., Olsen, C. E., Hick, A. J., Pickett, J. A., and Halkier, B. A. (2001). Cytochrome P450CYP79F1 from Arabidopsis catalyzes the conversion of dihomomethionine and trihomomethionine to the corresponding aldoximes in the biosynthesis of aliphatic glucosinolates. J. Biol. Chem. 276, 11078–11085. doi: 10.1074/jbc.M010123200
Hasegawa, T., Yamada, K., Kosemura, S., Yamamura, S., and Hasegawa, K. (2000). Phototropic stimulation induces the conversion of glucosinolate to phototropism-regulating substances of radish hypocotyls. Phytochemistry 54, 275–279. doi: 10.1016/S0031-9422(00)00080-7
Haughn, G. W., Davin, L., Giblin, M., and Underhill, E. W. (1991). Biochemical genetics of plant secondary metabolites in Arabidopsis thaliana: the glucosinolates. Plant Physiol. 97, 217–226. doi: 10.1104/pp.97.1.217
Hawkins, R. D., Hon, G. C., and Ren, B. (2010). Next-generation genomics: an integrative approach. Nat. Rev. Genet. 11, 476–486. doi: 10.1038/nrg2795
Hirai, M., Sugiyama, K., Sawada, Y., Tohge, T., Obayashi, T., Suzuki, A., et al. (2007). Omics-based identification of Arabidopsis Myb transcription factors regulating aliphatic glucosinolate biosynthesis Proc. Natl. Acad. Sci. U.S.A. 104, 6478–6483. doi: 10.1073/pnas.0611629104
Karban, R., and Baldwin, I. T. (1997). Induced Responses to Herbivory. Chicago, IL: University of Chicago Press.
Kerwin, R. E., Jiménez-Gómez, J. M., Fulop, D., Harmer, S. L., Maloof, J. N., and Kliebenstein, D. J. (2011). Network quantitative trait loci mapping of circadian clock outputs identifies metabolic pathway-to-clock linkages in Arabidopsis. Plant Cell 23, 471–485. doi: 10.1105/tpc.110.082065
Kim, S., Plagnol, V., Hu, T. T., Toomajian, C., Clark, R. M., Ossowski, S., et al. (2007). Recombination and linkage disequilibrium in Arabidopsis thaliana. Nat. Genet. 39, 1151–1155. doi: 10.1038/ng2115
Kliebenstein, D. J. (2007). “Metabolomics and plant quantitative trait locus analysis - the optimum genetical genomics platform?,” in Concepts in Plant Metabolomics, eds B. J. Nikolau and E. S. Wurtele. (Dordrect: Springer), 29–45.
Kliebenstein, D. J., Gershenzon, J., and Mitchell-Olds, T. (2001b). Comparative quantitative trait loci mapping of aliphatic, indolic and benzylic glucosinolate production in Arabidopsis thaliana leaves and seeds. Genetics 159, 359–370.
Kliebenstein, D. J., Kroymann, J., Brown, P., Figuth, A., Pedersen, D., Gershenzon, J., et al. (2001c). Genetic control of natural variation in Arabidopsis thaliana glucosinolate accumulation. Plant Physiol. 126, 811–825. doi: 10.1104/pp.126.2.811
Kliebenstein, D., Lambrix, V., Reichelt, M., Gershenzon, J., and Mitchell-Olds, T. (2001a). Gene duplication and the diversification of secondary metabolism: side chain modification of glucosinolates in Arabidopsis thaliana. Plant Cell 13, 681–693. doi: 10.1105/tpc.13.3.681
Kliebenstein, D., Pedersen, D., Barker, B., and Mitchell-Olds, T. (2002). Comparative analysis of quantitative trait loci controlling glucosinolates, myrosinase and insect resistance in Arabidopsis thaliana. Genetics 161, 325–332.
Lambrix, V., Reichelt, M., Mitchell-Olds, T., Kliebenstein, D. J., and Gershenzon, J. (2001). The Arabidopsis epithiospecifier protein promotes the hydrolysis of glucosinolates to nitriles and influences Trichoplusia ni herbivory. Plant Cell 13, 2793–2807. doi: 10.1105/tpc.13.12.2793
Li, B., Gaudinier, A., Taylor-Teeples, M., Nham, N. T., Ghaffari, C., Benson, D. S., et al. (2014). Promoter based integration in plant defense regulation. Plant Physiol. 166, 1803–1820. doi: 10.1104/pp.114.248716
Li, J., Hansen, B. G., Ober, J. A., Kliebenstein, D. J., and Halkier, B. A. (2008). Subclade of flavin-monooxygenases involved in aliphatic glucosinolate biosynthesis. Plant Physiol. 148, 1721–1733. doi: 10.1104/pp.108.125757
Mauricio, R. (1998). Costs of resistance to natural enemies in field populations of the annual plant Arabidopsis thaliana. Am. Nat. 151, 20–28. doi: 10.1086/286099
Mikkelsen, M. D., Naur, P., and Halkier, B. A. (2004). Arabidopsis mutants in the C-S lyase of glucosinolate biosynthesis establish a critical role for indole-3-acetaldoxime in auxin homeostasis. Plant J. 37, 770–777. doi: 10.1111/j.1365-313X.2004.02002.x
Nomoto, Y., Kubozono, S., Miyachi, M., Yamashino, T., Nakamichi, N., and Mizuno, T. (2012). A circadian clock- and PIF4-mediated double coincidence mechanism is implicated in the thermosensitive photoperiodic control of plant architectures in Arabidopsis thaliana. Plant Cell Physiol. 53, 1965–1973. doi: 10.1093/pcp/pcs141
Nordborg, M., Borevitz, J. O., Bergelson, J., Berry, C. C., Chory, J., Hagenblad, J., et al. (2002). The extent of linkage disequilibrium in Arabidopsis thaliana. Nat. Genet. 30, 190–193. doi: 10.1038/ng813
Nordborg, M., Hu, T. T., Ishino, Y., Jhaveri, J., Toomajian, C., Zheng, H., et al. (2005). The pattern of polymorphism in Arabidopsis thaliana. PLoS Biol. 3:e196. doi: 10.1371/journal.pbio.0030196
Nordborg, M., and Weigel, D. (2008). Next-generation genetics in plants. Nature 456, 720–723. doi: 10.1038/nature07629
Obayashi, T., Hayashi, S., Saeki, M., Ohta, H., and Kinoshita, K. (2009). ATTED-II provides coexpressed gene networks for Arabidopsis. Nucleic Acids Res. 37, D987–D991. doi: 10.1093/nar/gkn807
Paul-Victor, C., Züst, T., Rees, M., Kliebenstein, D. J., and Turnbull, L. A. (2010). A new method for measuring relative growth rate can uncover the costs of defensive compounds in Arabidopsis thaliana. New Phytol. 187, 1102–1111. doi: 10.1111/j.1469-8137.2010.03325.x
Ramakrishna, A., and Ravishankar, G. A. (2011). Influence of abiotic stress signals on secondary metabolites in plants. Plant Signal. Behav. 6, 1720–1731. doi: 10.4161/psb.6.11.17613
Schweizer, F., Fernandez-Calvo, P., Zander, M., Diez-Diaz, M., Fonseca, S., Glauser, G., et al. (2013). Arabidopsis basic helix-loop-helix transcription factors MYC2, MYC3, and MYC4 regulate glucosinolate biosynthesis, insect performance, and feeding behavior. Plant Cell 25, 3117–3132. doi: 10.1105/tpc.113.115139
Shen, X., Alam, M., Fikse, F., and Rönnegård, L. (2013). A novel generalized ridge regression method for quantitative genetics. Genetics 193, 1255–1268. doi: 10.1534/genetics.112.146720
Sønderby, I. E., Burow, M., Rowe, H. C., Kliebenstein, D. J., and Halkier, B. A. (2010). A complex interplay of three R2R3 MYB transcription factors determines the profile of aliphatic glucosinolates in Arabidopsis. Plant Physiol. 153, 348–363. doi: 10.1104/pp.109.149286
Sønderby, I. E., Hansen, B. G., Bjarnholt, N., Ticconi, C., Halkier, B. A., and Kliebenstein, D. J. (2007). A systems biology approach identifies a R2R3 MYB gene subfamily with distinct and overlapping functions in regulation of aliphatic glucosinolates. PLoS ONE 2:e1322. doi: 10.1371/journal.pone.0001322
Turchi, L., Carabelli, M., Ruzza, V., Possenti, M., Sassi, M., Penalosa, A., et al. (2013). Arabidopsis HD-Zip II transcription factors control apical embryo development and meristem function. Development 140, 2118–2129. doi: 10.1242/dev.092833
War, A. R., Paulraj, M. G., Ahmad, T., Buhroo, A. A., Hussain, B., Ignacimuthu, S., et al. (2012). Mechanisms of plant defense against insect herbivores. Plant Signal. Behav. 7, 1306–1320. doi: 10.4161/psb.21663
Wentzell, A. M., Rowe, H. C., Hansen, B. G., Ticconi, C., Halkier, B. A., and Kliebenstein, D. J. (2007). Linking metabolic QTLs with network and cis-eQTLs controlling biosynthetic pathways. PLoS Genet. 3:e162. doi: 10.1371/journal.pgen.0030162
Wittstock, U., and Halkier, B. A. (2002). Glucosinolate research in the Arabidopsis era. Trends Plant Sci. 7, 263–270. doi: 10.1016/S1360-1385(02)02273-2
Yamada, K., Hasegawa, T., Minami, E., Shibuya, N., Kosemura, S., Yamamura, S., et al. (2003). Induction of myrosinase gene expression and myrosinase activity in radish hypocotyls by phototropic stimulation. J. Plant Physiol. 160, 255–259. doi: 10.1078/0176-1617-00950
Zhao, K. Y., Aranzana, M. J., Kim, S., Lister, C., Shindo, C., Tang, C. L., et al. (2007). An Arabidopsis example of association mapping in structured samples. PLoS Genetics 3:e4. doi: 10.1371/journal.pgen.0030004
Keywords: Arabidopsis, allyl GSL, plant biomass, defense metabolism, GWAS, novel genes
Citation: Francisco M, Joseph B, Caligagan H, Li B, Corwin JA, Lin C, Kerwin RE, Burow M and Kliebenstein DJ (2016) Genome Wide Association Mapping in Arabidopsis thaliana Identifies Novel Genes Involved in Linking Allyl Glucosinolate to Altered Biomass and Defense. Front. Plant Sci. 7:1010. doi: 10.3389/fpls.2016.01010
Received: 20 May 2016; Accepted: 27 June 2016;
Published: 12 July 2016.
Edited by:
Basil J. Nikolau, Iowa State University, USAReviewed by:
Hideki Takahashi, Michigan State University, USARubén Alcázar, Universitat de Barcelona, Spain
Copyright © 2016 Francisco, Joseph, Caligagan, Li, Corwin, Lin, Kerwin, Burow and Kliebenstein. This is an open-access article distributed under the terms of the Creative Commons Attribution License (CC BY). The use, distribution or reproduction in other forums is permitted, provided the original author(s) or licensor are credited and that the original publication in this journal is cited, in accordance with accepted academic practice. No use, distribution or reproduction is permitted which does not comply with these terms.
*Correspondence: Daniel J. Kliebenstein, a2xpZWJlbnN0ZWluQHVjZGF2aXMuZWR1