- 1Beijing Key Laboratory of Development and Quality Control of Ornamental Crops, Department of Ornamental Horticulture and Landscape Architecture, China Agricultural University, Beijing, China
- 2National Engineering Research Center for Floriculture, Beijing Forestry University, Beijing, China
- 3Independent Researcher, Miki-cho, Japan
Thidiazuron (N-phenyl-N′-1,2,3-thiadiazol-5-ylurea; TDZ) is an artificial plant growth regulator that is widely used in plant tissue culture. Protocorm-like bodies (PLBs) induced by TDZ serve as an efficient and rapid in vitro regeneration system in Rosa species. Despite this, the mechanism of PLB induction remains relatively unclear. TDZ, which can affect the level of endogenous auxins and cytokinins, converts the cell fate of rhizoid tips and triggers PLB formation and plantlet regeneration in Rosa canina L. In callus-rhizoids, which are rhizoids that co-develop from callus, auxin and a Z-type cytokinin accumulated after applying TDZ, and transcription of the auxin transporter gene RcPIN1 was repressed. The expression of RcARF4, RcRR1, RcCKX2, RcCKX3, and RcLOG1 increased in callus-rhizoids and rhizoid tips while the transcription of an auxin response factor (RcARF1) and auxin transport proteins (RcPIN2, RcPIN3) decreased in callus-rhizoids but increased in rhizoid tips. In situ hybridization of rhizoids showed that RcWUS and RcSERK1 were highly expressed in columella cells and root stem cells resulting in the conversion of cell fate into shoot apical meristems or embryogenic callus. In addition, transgenic XVE::RcWUS lines showed repressed RcWUS overexpression while RcWUS had no effect on PLB morphogenesis. Furthermore, higher expression of the root stem cell marker RcWOX5 and root stem cell maintenance regulator genes RcPLT1 and RcPLT2 indicated the presence of a dedifferentiation developmental pathway in the stem cell niche of rhizoids. Viewed together, our results indicate that different cells in rhizoid tips acquired regeneration competence after induction by TDZ. A novel developmental pathway containing different cell types during PLB formation was identified by analyzing the endogenous auxin and cytokinin content. This study also provides a deeper understanding of the mechanisms underlying in vitro regeneration in Rosa.
Introduction
Rosa species are widely cultivated around the world because of their ornamental and high economic value (Debener and Linde, 2009). Over the past decade, genetic engineering and genomic approaches have been used to improve several traits in the genus Rosa, including disease resistance, recurrent flowering, scent production, flower color, and flower architecture (Tanaka et al., 2005; Zlesak, 2009). Several regeneration pathways have been explored and used for rose transformation (Firoozabady et al., 1994; Hsia and Korban, 1996; Kim et al., 2003, 2004; Chen et al., 2006, 2010; Pati et al., 2006; Randoux et al., 2014). However, regeneration systems of Rosa plants in vitro continue to be inefficient and time-consuming, seriously limiting the application of transgenic engineering to this ornamental. Tian et al. (2008) established an efficient and rapid regeneration system using protocorm-like bodies (PLBs) that formed in response to thidiazuron (N-phenyl-N′-1,2,3-thiadiazol-5-ylurea; TDZ) (Tian et al., 2008). In this PLB regeneration system, leaves excised from 6-week-old shoots were first used to induce callus and rhizoids (a root-like structure that has no root cap as in a normal root, but instead only possessing a root apical meristem (RAM) and root stem niche) in vitro in the dark on Murashige and Skoog (MS) (Murashige and Skoog, 1962) basal medium supplement with 1.5 mg/L 2,4-D. In that study, PLBs that formed on the tips of rhizoids turned green and were bulbous after subculture on PLB induction medium (PIM), which consists of half-strength (micro- and macronutrients) MS basal medium (1/2 MS) with 20 mg/L TDZ and 2% sucrose. PLBs, which are developmental structures generally associated with structures similar to seed-derived protocorms in orchids (Teixeira da Silva, 2014), have served as a practical approach for the in vitro regeneration of nine Rosa species (Guo, 2009). In a downstream application, the GUS gene was successfully transformed into Rosa canina L. PLBs (Bi et al., 2012). Histochemical observations showed that PLBs were derived from parenchyma cells adjacent to the epidermis of rhizoid tip cells (Liu et al., 2014). Other than this, little is known about how TDZ triggers PLB formation, especially the changes in the endogenous content of auxins and cytokinins (CKs), or in the expression of related genes.
TDZ, which is a synthetically derived urea displaying primarily CK-like activity, is the only plant growth regulator (PGR) that has been used to date to induce PLBs in R. canina. TDZ is used more generally in plant tissue culture for inducing shoots and somatic embryos (Li et al., 2002; Feyissa et al., 2005), protoplasts (Kim et al., 2003), and flowers (Chang and Chang, 2003) in vitro. TDZ can be used as a substitute for both auxin and CK (Thomas and Katterman, 1986; Visser et al., 1992; Casanova et al., 2004). However, there are few reports about the biochemical or genetic mechanisms underlying how cells acquire regeneration competence following induction by TDZ.
Plant regeneration involves successive developmental events in response to environmental cues (Fehér, 2015). The acquisition of regeneration competence is easily achieved in plant tissue culture by adjusting the exogenous levels of PGRs (Che et al., 2007). Indole-3-acetic acid (IAA) is a major form of auxin in plants (Brunoud et al., 2012). Auxin concentration gradients play a critical role in plant development both in vivo and in vitro, and auxin polarization and redirection through auxin transporter protein determine tissue morphogenesis, such as lateral organ initiation and definition of the apicobasal embryonic axis (Robert et al., 2013; Wabnik et al., 2013). Members of the PIN-FORMED (PIN) gene family encode components of the auxin efflux machinery, including PIN-FORMED1 (PIN1), PIN-FORMED2 (PIN2), PIN-FORMED3 (PIN3), PIN-FORMED4 (PIN4), and PIN-FORMED7 (PIN7), which function singly or in combination to direct auxin flux and reflux in Arabidopsis roots (Blilou et al., 2005; Adamowski and Friml, 2015). PIN1 appears to be the primary mediator of IAA movement through vascular tissues and is essential for acropetal transport (to the root tip) of auxin in root tissue (Galweiler et al., 1998; Blilou et al., 2005). PIN2 is notable for its polarized localization and root gravitropic response (Müller et al., 1998) and also determines the redistribution of auxin in the basipetal (out of the root tip) transport stream through cortical and epidermal cells in root tips (Benkova et al., 2003; Blilou et al., 2005). PIN3, which appears to function in the lateral redistribution of auxin, and is involved in phototropic and gravitropic growth, is localization in the cells of the shoot endodermis, gravi-responding root columella and pericycle cells (Friml et al., 2002a,b). Auxin response factors (ARFs), together with the TIR1/AFB F-box and Aux/IAA proteins, constitute the auxin signal transduction pathway. The ARF gene family plays an important role in regulating the transcription of auxin stimulation during plant development (Tan et al., 2007; Chapman and Estelle, 2009; Guilfoyle and Hagen, 2012). There are 23 genes coding for ARF proteins in Arabidopsis and which serve as B3-type transcription factors (Guilfoyle and Hagen, 2007). ARF proteins can be divided into activators and repressors of auxin signaling based on their activity in protoplasts: ARFs 5, 6, 7, 8, and 19 act as activators while other ARFs act as repressors (Guilfoyle and Hagen, 2012).
Cytokinins also participate in many aspects of plant development (Werner and Schmülling, 2009). The ribosides isopentenyladenosine (iPA) and zeatin riboside (ZR) are the translocated forms of the Z- and iP-type of CK, respectively (Takei et al., 2001). CK signaling is mediated by a two-component signaling pathway, which contains the CK receptor histidine kinases (HKs), histidine phosphotransferases (HPs), and response regulators (RRs) (Hwang and Sheen, 2001; Hwang et al., 2012). In Arabidopsis, ARR1 is considered to be a marker of CK signaling because of its immediate response to CK (Sakai et al., 2001) and has been reported to be critical in many processes during plant development (Jones et al., 2010). The AHK3/ARR1 two-component signaling pathway leads to decreased expression of PIN1, PIN3, and PIN7 and impairs cell differentiation (Dello Ioio et al., 2008). To metabolize CKs in plants, iP- and Z-type CKs are released from their nucleotide precursor formed by CK nucleoside 5′-monophosphate phosphoribohydrolase LONELY GUY (LOG1) and become active (Kurakawa et al., 2007). Wong et al. (2013) showed that the expression of GmLOG1 in the axillary shoot meristem was earlier than that of GmWUS or GmCLV3 during the initial stage of axillary shoot meristem development in soybean (Glycine max L.), which indicates that CKs act as one of the earliest signals in initiating and specifying the shoot stem cell population. Tamar et al. (2013) noted that ectopic expression of the LOG1 gene in tomato (Lycopersicon esculentum Mill.) induced aerial minitubers from tomato shoot buds. CK oxidase/dehydrogenase (CKX) are enzymes that catalyze the breakdown of all CKs in a single step (Werner et al., 2006). The CK-degrading enzyme CYTOKININ OXIDASE 3 (CKX3) is expressed in the organizing center of the shoot apical meristem (SAM) (Yadav et al., 2009; Bartrina et al., 2011), and ckx3-ckx5 double mutants displayed increased WUS expression and larger SAMs (Bartrina et al., 2011).
Many functional genes have been identified and confirmed to be determining factors in plant regeneration. For example, WUSCHEL RELATED HOMEOBOX 5 (WOX5) is specifically expressed in the quiescent center (QC) and is considered to be a marker of the root stem cell niche (Sarkar et al., 2007). PLETHORA 1 (PLT1) and PLETHORA 2 (PLT2) are master regulators involved in the maintenance of basal/root fate (Aida et al., 2004; Galinha et al., 2007). Sugimoto et al. (2010) showed that Arabidopsis could regenerate shoots from multiple tissues, but all indirectly through a callus pathway, and that the development of callus shared the same traits as in lateral root development. In Arabidopsis, the root stem cell regulators PLT1 and PLT2 must be activated to establish the competence of shoot regeneration progenitor cells (Kareem et al., 2015). WUSCHEL (WUS) is specifically expressed in the center of SAMs, and is considered to be a marker of SAM identity. WUS, together with CLAVATA 1/3 (CLV1/3), maintains the SAM stem cell niche through a feedback pathway in Arabidopsis (Schoof et al., 2000). WUS is also considered to induce shoot stem cell activity in roots (Gallois et al., 2004) and to convert RAMs to SAMs, depending on the exogenous PGR applied in vitro (Chatfield et al., 2013). SOMATIC EMBRYOGENESIS RECEPTOR KINASE 1 (SERK1), which is involved in the acquisition of embryogenic competence in plant tissue culture, is strongly expressed during early stages of zygotic and somatic embryogenesis in Arabidopsis (Hecht et al., 2001; Zhang et al., 2014), and has a broader role in Medicago truncatula Gaertn. organogenesis (Nolan et al., 2003).
In this study, using the R. canina PLB model developmental system, the main aim was to assess how rhizoid cells attain regeneration competence following induction by TDZ. Furthermore, it was our sub-objective to assess the content of endogenous auxin and CKs in developing PLBs. Finally, another sub-objective was to observe the transcription levels of genes related to auxin, CKs, the RAM and the SAM. In doing so, we hoped to better understand the molecular inductive function of TDZ during PLB formation in R. canina and thus provide a better understanding of the mechanistic basis of this unique in vitro developmental event in Rosa species.
Materials and Methods
Plant Material and In Vitro Regeneration
Callus-rhizoids (i.e., rhizoids that co-develop from callus) derived from 6-week-old leaves were transferred onto PIM, which was 1/2 MS supplemented with 20 mg/L TDZ based on a previously described protocol (Tian et al., 2008). After culture for 0, 1, 2, 3, 4, or 5 days on PIM, callus-rhizoids and the terminal 1 cm of rhizoid tips were collected and stored at -80°C for later use. Cultures were maintained at 25 ± 2°C under a 16-h photoperiod with a light intensity of 25 μmol m-2 s-1 provided by cool-white fluorescent tubes.
Three XVE::RcWUS transgenic plant lines (#5, #8, #9) were used to assess the percentage of PLBs that formed during the early stage (the first 10 days on PIM) of PLB formation.
Quantification of IAA, ZR, and iPA Using ELISA
Fresh callus (0.2 g) that included rhizoids (i.e., rhizoids-callus) obtained from PIM was ground into a fine powder with liquid nitrogen and dissolved in 2.0 mL of phosphate-buffered saline (PBS) containing 0.1% (v/v) Tween-20 and 0.1% (w/v) gelatin (pH 7.5) to quantify the amount of free IAA, ZR, and iPA, using an enzyme-linked immunosorbent assay (ELISA) (Yang et al., 2001). Mouse monoclonal antibodies against free IAA, ZR and iPA were produced at the Center of Crop Chemical Control, China Agricultural University, China (Weiler et al., 1981; Wang et al., 2012). ELISA data was calculated as described previously (Weiler et al., 1981).
Treatment of Callus-Rhizoids with Exogenous Factors
1-Naphthaleneacetic acid (NAA), N-1-naphthylphthalamic acid (NPA, an inhibitor of auxin transport) and kinetin (KT; 6-furfuryl aminopurine) were used to assess the effect of TDZ on PLB formation. The ratio of PLB formation [(number of PLBs/total number of rhizoids per callus-rhizoid) × 100] was calculated after each treatment. One callus-rhizoid indicates a single unit of callus-rhizoids derived from one leaf (Figures 2B,C). For auxin treatment, leaves with callus-rhizoids were cultured on PIM containing 0.01, 0.1, or 1.0 mg/L NAA. For the NPA treatments, leaves with callus-rhizoids were cultured on PIM supplement with 10 μL NPA while for the KT treatment, leaves with callus-rhizoids were cultured on PIM containing 0.01, 0.1, or 1.0 mg/L KT. Twenty callus-rhizoids were used for each treatment, and each experiment was performed five times. After culture for 9 days, the ratio of PLB formation in each treatment was calculated.
Quantitative Real-Time PCR
Total RNA was extracted from callus-rhizoids or rhizoids with a Huayueyang RNA extraction kit (Huayueyang, ZH120, Beijing, China). Two μL of RNA was used to synthesize cDNA in a reaction volume of 20 μL using a FastQuant RT Kit (Tiangen, KR106, Beijing, China), which contains gDNase and a random primer. cDNA was then used for quantitative real-time PCR (qRT-PCR) analysis on an ABI 7500 Real-Time PCR System (Applied Biosystems, Foster City, CA, USA) using a KAPATM SYBR® FAST qPCR kit (KapaBiosystems, Woburn, MA, USA) according to the manufacturer’s instructions. The PCR protocol was: denaturation at 95°C for 30 s, 40 cycles at 95°C for 5 s for product amplification and a final extension at 60°C for 30 s. The gene-specific primers used for qRT-PCR are listed in Supplementary Table S1. Gene expression levels were normalized to 18S and UBIQUTIN2 transcripts using the comparative ΔΔCt method (Livak and Schmittgen, 2001).
RNA In Situ Hybridization
Intact rhizoids approximately 1 cm long were excised from callus-rhizoids after culture on PIM for 0, 3, 5, or 9 days. Rhizoid tips (∼1 cm long) were fixed in FAA (formaldehyde, acetic acid, 50% ethanol 5:5:90, v/v/v) for a minimum of 24 h. After dehydration through an alcohol-xylol series, rhizoid tips were embedded in paraffin (Sigma–Aldrich, Mannheim, Germany) with a 58°C melting point (Liu et al., 2014). Serial longitudinal sections (10 μm thick) of the rhizoids made with a microtome (Leica RM2235, Solms, Germany) were used for in situ hybridization in which fragments of RcWUS, RcPLT1, or RcSERK1 were used to generate sense and antisense RNA probes by PCR amplification using a DIG RNA Labeling Kit, according to the manufacturer’s instructions (Roche, Mannheim, Germany). PCR conditions were as follows: 95°C for 30 s, 40 cycles at 95°C for 5 s, and 58°C for 30 s. The gene-specific primer sequences are listed in Supplementary Table S2. All procedures were performed at Prof. Zheng Meng’s Lab (Institute of Botany, Chinese Academy of Sciences). The slides were left in a sealed and humid box in a dark drawer to prevent light contamination for 18 h, and then stained sections were examined and photographed under a BX-50 light microscope (Leica DMRE, Solms, Germany).
Results
Endogenous Auxin and CK Levels Were Increased by TDZ
Auxin and CK determine regeneration in plant tissue culture. To clarify the effect of TDZ on endogenous levels of auxin and CK, we measured the concentration of IAA (endogenous auxin), iPA and ZR (both endogenous CKs). In callus-rhizoids, TDZ increased IAA and ZR levels (Figures 1A,B) and decreased the level of iPA (Figure 1C) during the first 5 days. The increase in IAA concentration was smoother than that of ZR and iPA and peaked on day 4 (Figure 1A). The level of ZR increased rapidly, peaking on day 2, dipping on day 3, then increasing gradually again on days 4 and 5 (Figure 1B). The content of iPA was lower than that of ZR and IAA and remained stable from day 2 to 5 (Figure 1C).
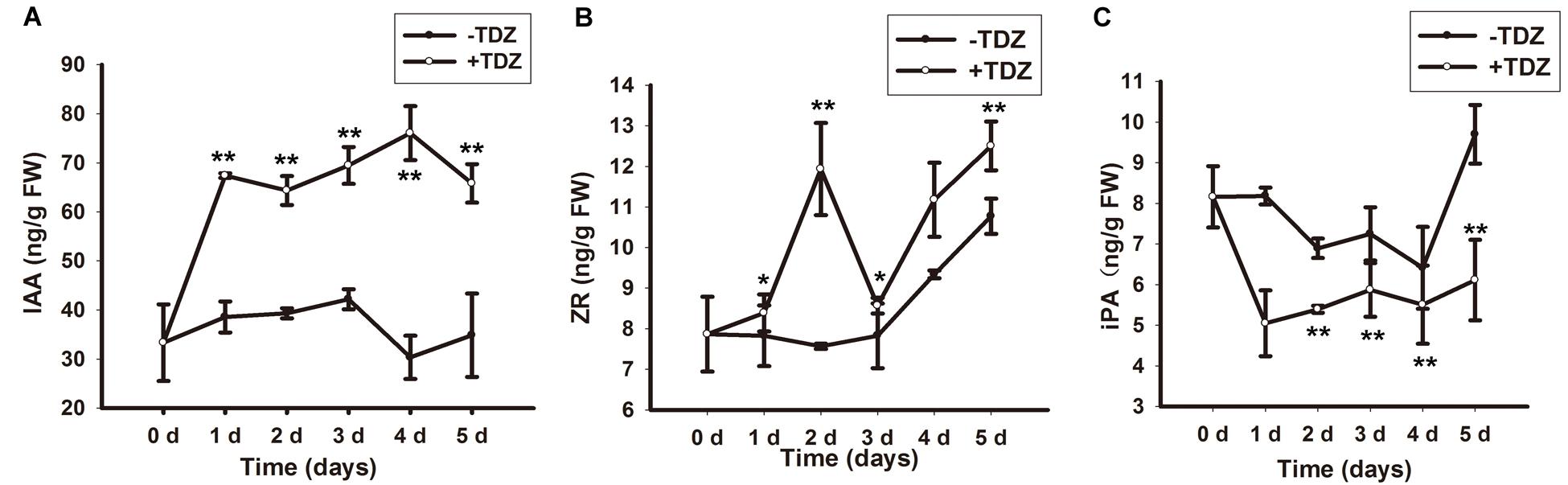
FIGURE 1. Endogenous IAA, ZR and iPA levels in callus-rhizoids after culture on PIM. Callus-rhizoids (0.2 g fresh) were collected after culture on PIM (1/2 MS + 20 mg/L TDZ) or 1/2 MS media without TDZ for 0, 1, 2, 3, 4, or 5 days. (A) The level of IAA and ZR was significantly increased by TDZ during the first 5 days of culture on PIM. (B) The endogenous level of ZR was repressed by TDZ. (C) The endogenous level of iPA was increased by TDZ, especially on day 2. Error bars represent the SE of three biological replicates. Asterisks indicate significant (∗P < 0.05) or highly significant (∗∗P < 0.01) differences calculated using the t-test (n = 9 for each treatment).
In brief, TDZ promoted the accumulation of endogenous IAA and ZR directly in callus-rhizoids. This is in agreement with previous results in which TDZ acted as a substitute for both auxin and CK during plant tissue culture (Visser et al., 1992; Casanova et al., 2004). Moreover, the level of IAA was a little lower and ZR was significantly higher on day 2 compared with days 1 and day 3.
TDZ Acts Primarily as a CK during PLB Formation
Since the level of iPA was reduced in callus-rhizoids, we applied an additional treatment to callus-rhizoids cultured on PIM to investigate the effect of TDZ during the first 10 days of PLB formation. NAA or KT at 0.01, 0.1, or 1.0 mg/L was added to PIM, and then the number of PLBs on each callus-rhizoid was counted after culture for 9 days. The percentage of PLBs that formed decreased (Figures 2B,G; Supplementary Table S4) as NAA concentration increased and 1.0 mg/L NAA induced the fewest PLBs (only 48.12%) compared to the control (54.56%). In contrast, KT promoted PLB morphogenesis (Figures 2C,G; Supplementary Table S4) with 0.1 mg/L KT being the optimal concentration (PLBs forming in 61.54% of explants). However, 1.0 mg/L KT did not promote PLB formation, suggesting that this concentration was too high for PLB morphogenesis. In addition, PLBs were large and white on PIM containing 1.0 mg/L NAA (Figures 2A,E), but were small and green on PIM containing 1.0 mg/mL KT (Figures 2D,F). Rhizoid tips turned green 1 day earlier (they turned green on day 3) after KT treatment compared with the control (Figures 2D,E). Thus, we propose that TDZ acts in synergy with KT to promote PLB morphogenesis, but that PLB morphogenesis was inhibited by auxin. To test this hypothesis, we added 1 μM NPA, which is an inhibitor of auxin transport, to PIM, noting that the percentage of PLB formation increased to 69.23% (Figure 2G; Supplementary Table S4). Consequently, we concluded that TDZ mainly acted as a Z-type CK during the early stage of PLB morphogenesis.
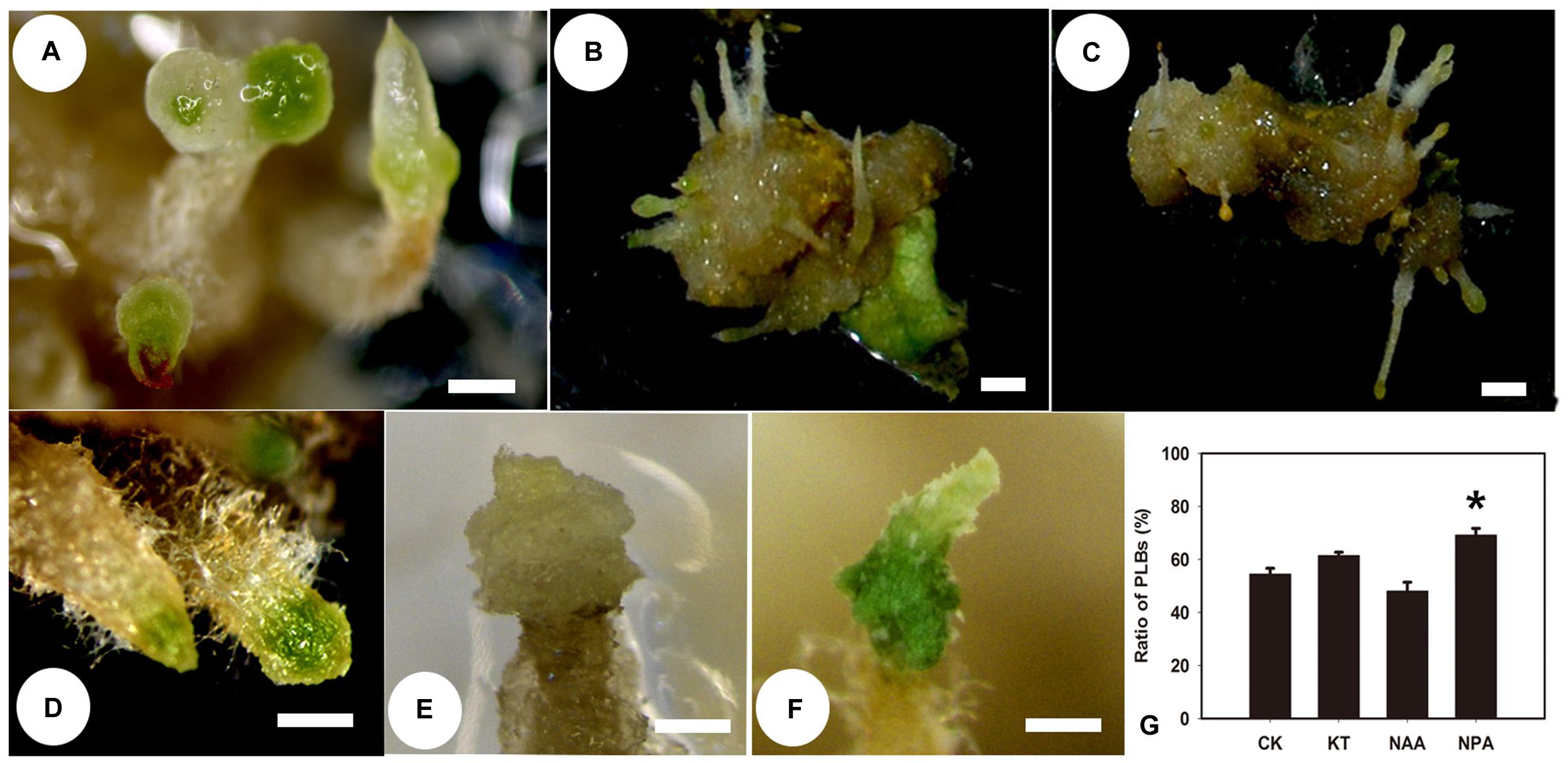
FIGURE 2. Protocorm-like bodies (PLB) formation is affected by supplementary NAA, KT, and NPA. Rhizoid tips began to swell and turn green after callus-rhizoids were transferred to PIM. This process was defined as PLB formation. The percentage of callus-rhizoids forming PLBs was calculated after culture on PIM for 9 days. (A) PLBs normally formed after 9 days of culture on PIM, and the rhizoid tip swelled and turned green. (B,E) PLB formation was inhibited after culture on PIM supplemented with 1.0 mg/L NAA, but some large white PLBs formed. (C,D,F) PLB formation increased when callus-rhizoids were cultured on PIM containing 0.1 mg/L KT. Rhizoid tips turned green earlier after KT treatment compared with the control. (G) Rate of PLB formation on medium with different PGRs compared with CK. Error bars represent the SE of three biological replicates. Asterisks indicate highly significant differences calculated using the t-test (∗P < 0.05) (n = 9–12 for each treatment).
Expression of CK Signaling and Metabolism-Related Genes was Promoted by TDZ
The expression pattern of genes related to CK signaling and metabolism was evaluated to obtain insight into the function of TDZ during PLB formation. The expression of RcRR1 increased in both callus-rhizoids and rhizoid tips (Figures 3B,D), which implied that CK signaling was promoted by TDZ. RcLOG1 was used to analyze endogenous CK synthesis. Our results show that RcLOG1 increased markedly in callus-rhizoids but the promoting effect of TDZ on RcLOG1 transcription was weaker in rhizoid tips. These results indicate that TDZ promotes endogenous CK synthesis.
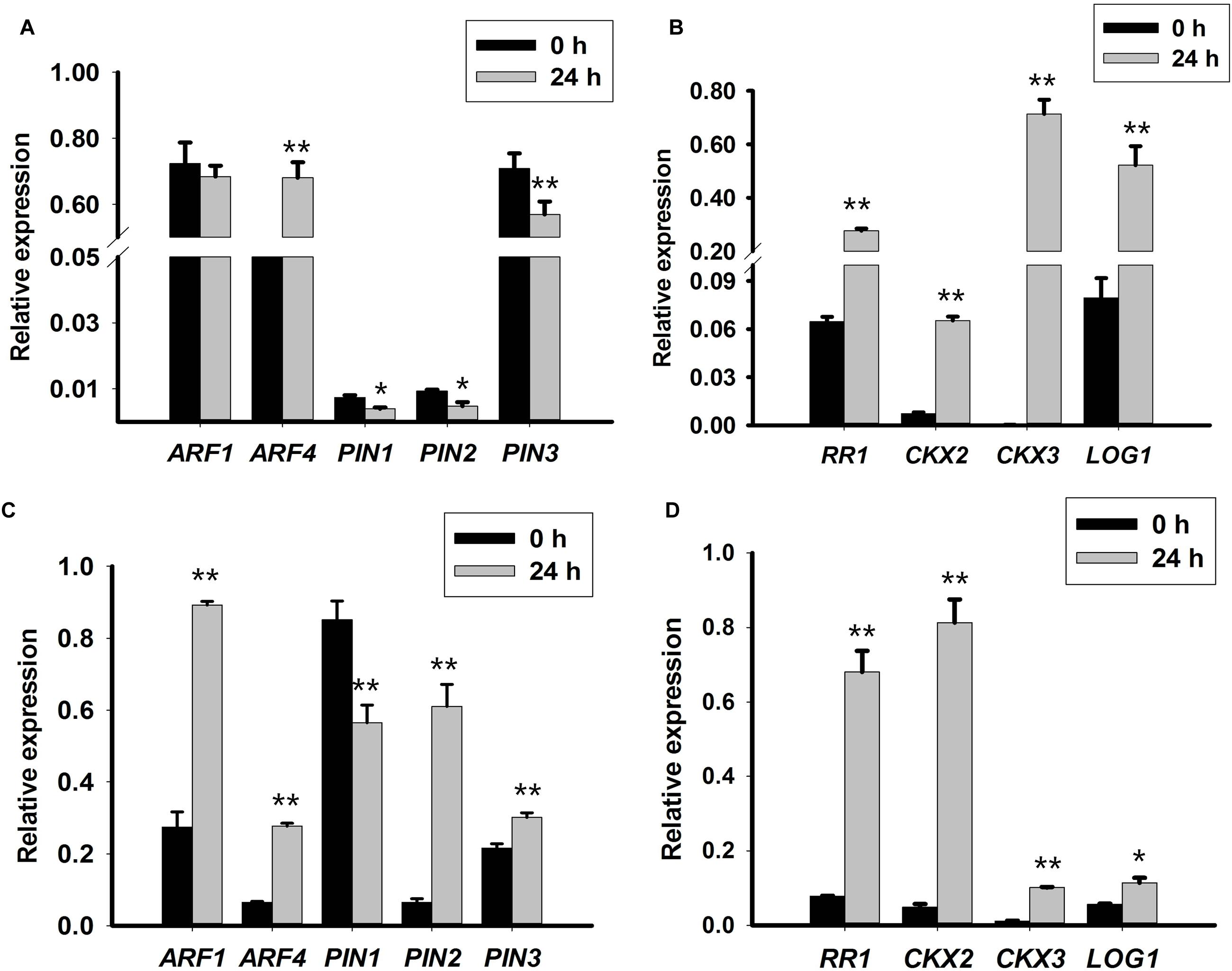
FIGURE 3. Expression levels of auxin- and cytokinin-related genes in callus-rhizoids or rhizoid tips. Callus-rhizoids (0.1–0.2 g fresh) or rhizoids only were collected after culture on PIM for 0 or 24 h. Quantitative real-time PCR was used to analyze genes expression levels. (A,B) Gene expression in callus-rhizoids. (C,D) Gene expression in rhizoid tips. Error bars represent the SE of three biological replicates. Error bars represent the SE of three biological replicates. Asterisks indicate significant (∗P < 0.05) or highly significant (∗∗P < 0.01) differences calculated using the t-test (n = 3 for each treatment).
As mentioned above, the level of iP-type of CK (iPA) was reduced. Thus, the expression pattern of RcCKX2 and RcCKX3 was evaluated. The results show that both RcCKX2 and RcCKX3 expression increased both in callus-rhizoids and rhizoid tips (Figures 3B,D). However, RcCKX2 transcription was more significantly promoted in rhizoid tips while transcription of RcCKX3 was more significantly promoted in callus-rhizoids. This also confirmed our opinion that TDZ acted mainly as a CK during PLB morphogenesis.
Most importantly, we concluded that CK signaling, biosynthesis, and degradation were promoted during PLB formation by TDZ induction, and that genes related to CK signaling, biosynthesis and degradation participated in PLB development.
Auxin Signaling Was Promoted but Auxin Transporters Were Repressed by TDZ
Transcription of RcARF1 (Figures 3A,C) was repressed in callus-rhizoids but was slightly promoted in rhizoid tips. The transcription of RcARF4, which is a repressor of auxin signaling, was promoted (Figures 3A,C) both in callus-rhizoids and rhizoid tips. These results indicate that auxin signaling was mainly repressed through the promotion of ARF repressors even though the overall concentration of IAA increased in callus-rhizoids.
We assessed the transcription levels of RcPIN1, RcPIN2, and RcPIN3 to clarify the role of auxin during PLB morphogenesis by TDZ induction. The results show that RcPIN1 was repressed in both callus-rhizoids and rhizoid tips. Transcription of RcPIN2 was inhibited in callus-rhizoids but was promoted in rhizoid tips. The expression level of both RcPIN1 and RcPIN2 was down-regulated in callus-rhizoids more than in rhizoid tips. Repression of RcPIN1 expression may account for the accumulation of auxin (i.e., a decrease in its efflux) in callus-rhizoids (Figures 3A,C). The level of RcPIN2 transcription was significantly promoted in rhizoid tips but that of RcPIN1 was repressed. Transcription of RcPIN3 was repressed in callus-rhizoids but promoted in rhizoid tips, indicating that RcPIN3 expression was down-regulated by TDZ directly.
In summary, auxin transport was repressed in rhizoids by TDZ induction, and the balance of hormones in rhizoid tips was disturbed, promoting the conversion of cell fate.
Expression of Root and Shoot Stem Cell-Related Genes Increased Following TDZ Induction
To further understand how TDZ converted cell fate during PLB formation, root and shoot stem cell-related genes were analyzed in the first 10 days of PLB formation. The expression levels of RcWUS, RcCLV1, RcPLT1, RcPLT2, RcWOX5, RcWUS, and RcSERK1 were evaluated to interpret the genetic mechanism underlying PLB morphogenesis. The results show that transcription of RcPLT1 (Figure 4B) was repressed in callus-rhizoids but significantly promoted in rhizoid tips (Figure 4D). The expression of RcPLT2 (Figure 4B) increased in callus-rhizoids and rhizoid tips (Figure 4D), but especially in rhizoids. RcWOX5 expression (Figure 4B) decreased in callus-rhizoids but increased in rhizoids (Figure 4B). The expression of RcSERK1 did not change in callus-rhizoids but increased significantly in rhizoid tips (Supplementary Figure S2A).
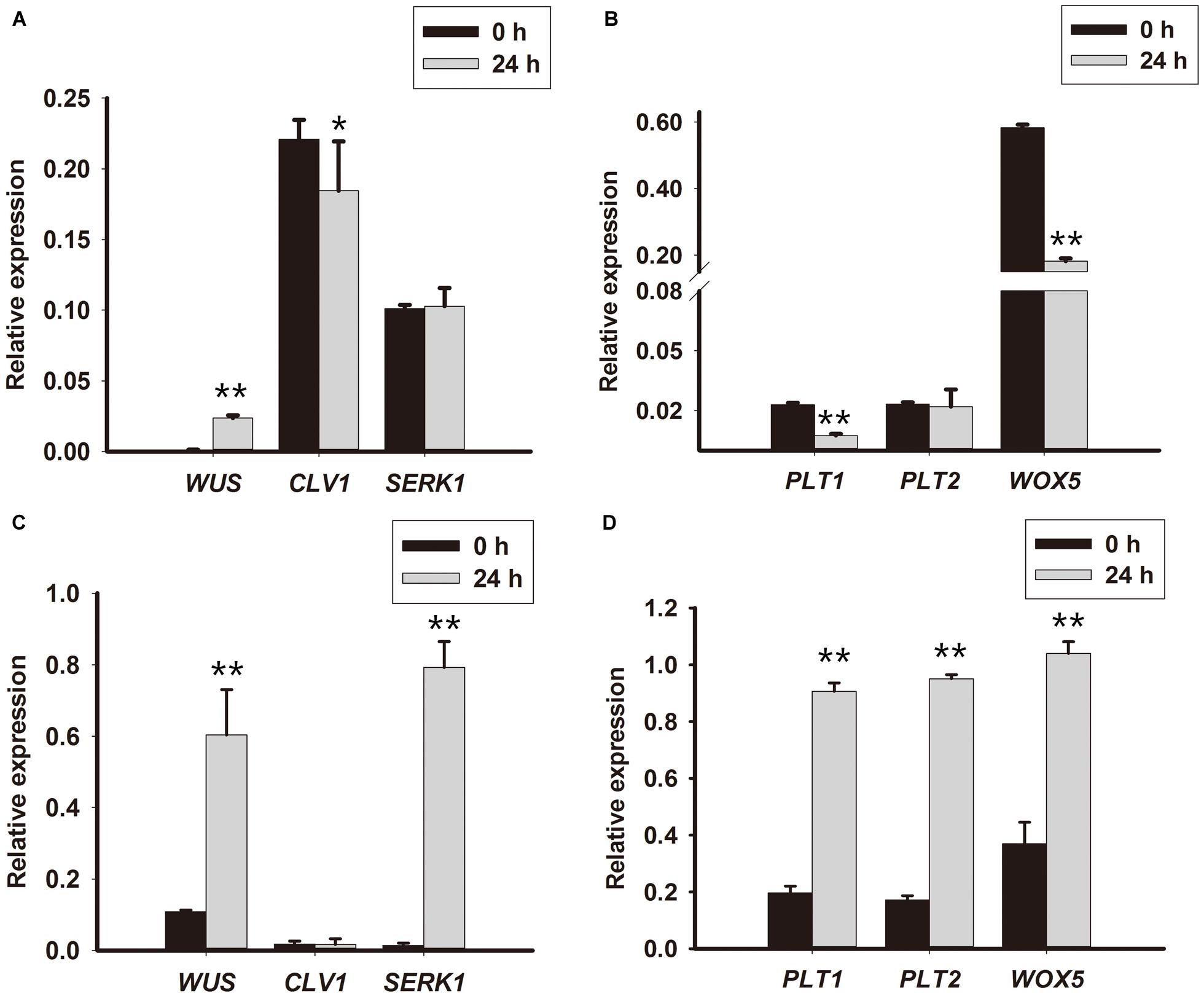
FIGURE 4. Expression levels of root and shoot stem niche-related genes in rhizoid tips. Callus-rhizoids (0.1–0.2 g fresh) and rhizoids were collected after culture on PIM for 0 or 24 h. Quantitative real-time PCR was used to analyze genes expression levels. (A,B) Gene expression in callus-rhizoids. (C,D) Gene expression in rhizoid tips. Error bars represent the SE of three biological replicates. Asterisks indicate significant (∗P < 0.05) or highly significant (∗∗P < 0.01) differences calculated using the t-test (n = 3 for each treatment).
RcWUS transcription was promoted both in callus-rhizoids and rhizoids (Figures 4A,C), but RcCLV1 expression was reduced in callus-rhizoids (Figure 4C) but only changed slightly in rhizoid tips (Figure 4C) after culture for 24 h. In addition, RcWUS expression increased in callus-rhizoids as TDZ concentration increased after culture for 16 h, with 20 mg/L TDZ being the optimal concentration to promote RcWUS transcription (Supplementary Figure S1). These results suggest that RcWUS could be induced directly by TDZ and that a feedback between RcWUS and RcCLV1 had not been established within 24 h following TDZ induction.
The RcWUS gene is important for SAM and marks the position of shoot stem cell identity in plants. In our study, there was a significant increase in the expression of RcWUS in rhizoid tips, indicating that SAMs were established during the early stage of PLB morphogenesis. Consequently, we propose that TDZ triggered PLB formation following an increase in RcWUS expression.
Cell Fate Was Converted to a Different Development Pathway in Rhizoid Tips
To understand the developmental pathway during the early stage of PLB morphogenesis, we analyzed the expression of RcSERK1, RcWUS and RcPLT1 in rhizoid tips using qRT-PCR and RNA in situ hybridization during the early stage of PLB formation. The results show that RcWUS expression increased significantly during the first 24–72 h (Figure 5A) then decreased on day 3. RNA in situ hybridization showed that RcWUS was expressed mainly in the outer layers (Figure 5B) of rhizoid tips before TDZ treatment (day 0). After treatment with TDZ for 3 days, RcWUS expression extended toward the epidermis, columella cells and even the QC (Figure 5C). However, on day 5, RcWUS expression decreased once again. The columella cells, which began to enlarge or convert into SAMS (Figure 5D), showed increased RcWUS expression on day 3.
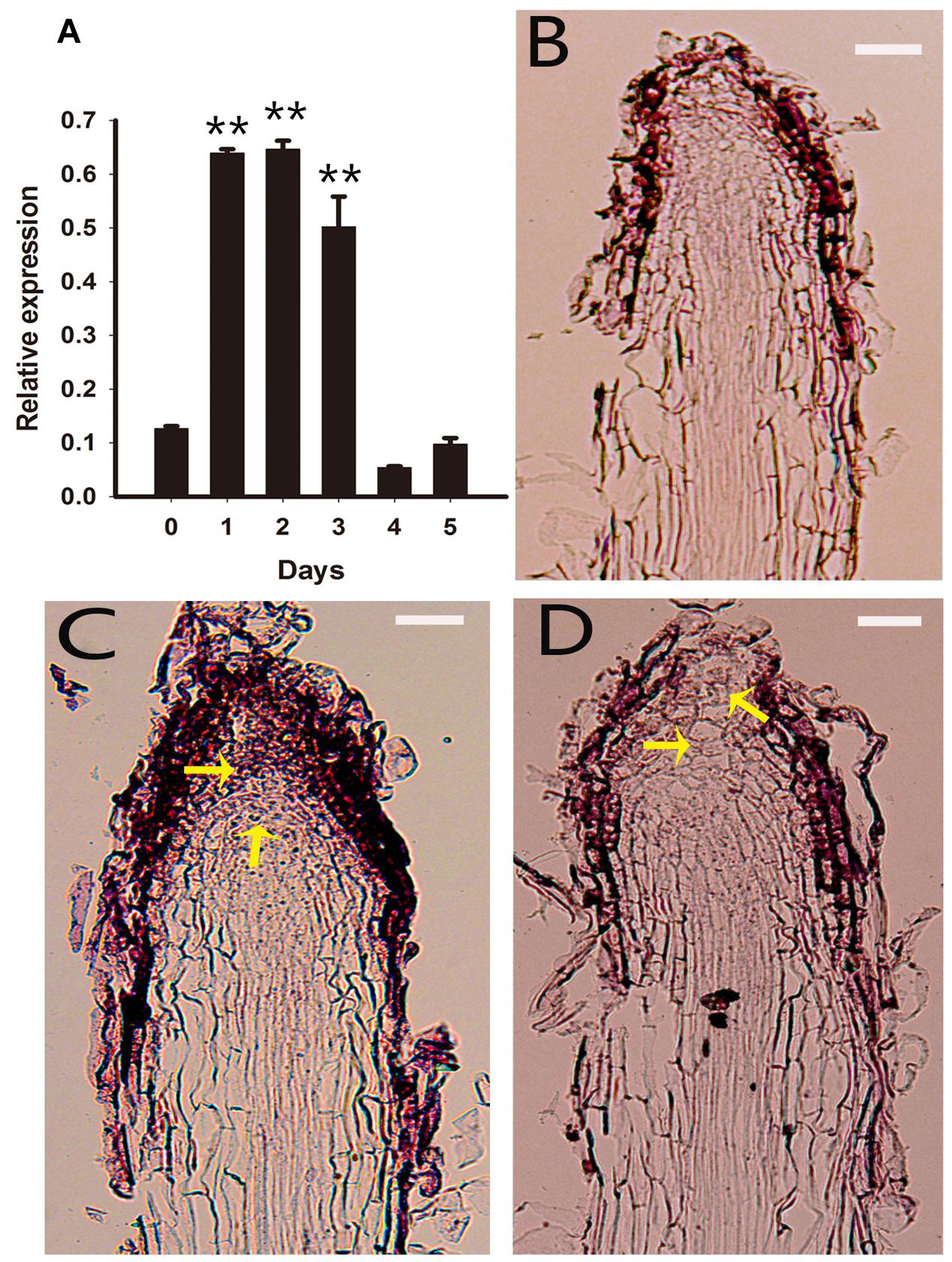
FIGURE 5. Expression pattern of RcWUS in rhizoid tips. Rhizoid tips (1 cm long) were collected after culture on PIM for 0, 1, 2, 3, 4, and 5 days. (A) Quantitative real-time PCR analysis of RcWUS expression pattern during the first 5 days of PLB formation. Error bars represent the SE of three biological replicates. Asterisks indicate significant differences calculated using the t-test (**P < 0.01) (n = 3 for each treatment). (B–D) In situ hybridization in longitudinal sections of rhizoid tips. RcWUS expression in rhizoid tips (B) before culture on PIM, (C) after culture for 3 days, or (D) after culture for 5 days. Bars = 2 μm.
In brief, our results show that the expression of RcWUS was transiently increased by TDZ during the early stage of PLB formation. RcSERK1 expression increased significantly at 24 h (Supplementary Figure S2A) on PIM, corresponding to the RcSERK1 in situ hybridization results (Supplementary Figures S2B–D). These expression patterns of RcSERK1 and RcWUS showed that cell fate converted to shoot stem cells and that developmental plasticity was induced in the RAM.
RcPLT1 is required for establishing competence to regenerate shoot progenitor cells in Arabidopsis (Kareem et al., 2015). Thus, we analyzed the expression pattern of RcPLT1 to understand de novo shoot regeneration in PLB formation. Our results show that RcPLT1 expression increased gradually from day 2 onward (Figure 6A). RNA in situ hybridization showed that the zone of RcPLT1 expression extended toward the outer layer of the rhizoid around the stem cell niche (Figures 6B,C) where PLBs originated. In addition, RcPLT1 expression increased significantly (Figure 6D) and extended to the whole rhizoid tip before swelling to a fully formed PLB. These results imply that a de novo shoot regeneration pathway may be activated and that cell fate was changed by TDZ induction.
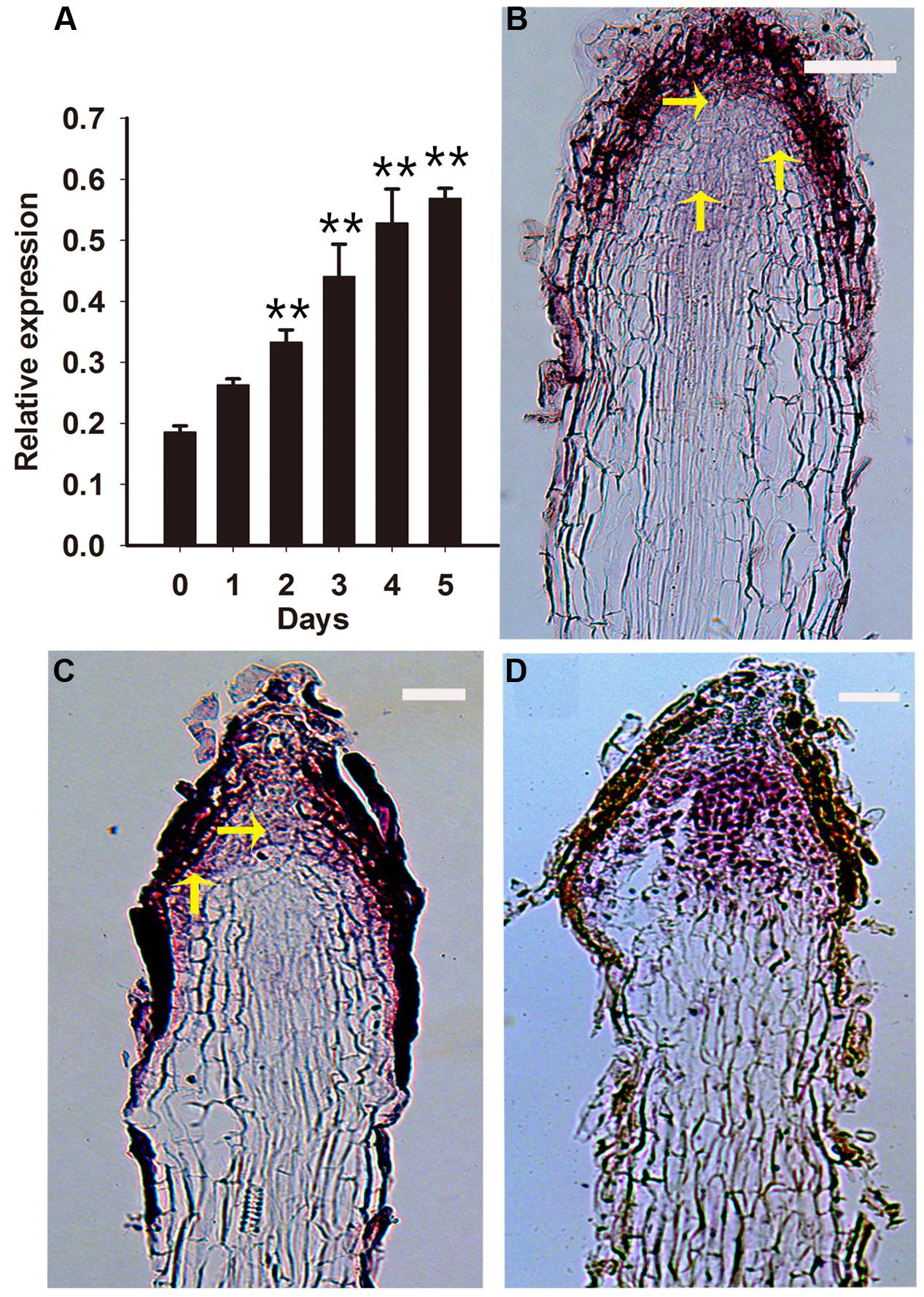
FIGURE 6. Expression pattern of RcPLT1 in rhizoid tips. Rhizoid tips were collected after culture on PIM for 0, 1, 2, 3, 4, and 5 days. (A) Quantitative real-time PCR analysis of RcPLT1 expression pattern during the first 5 days of PLB formation. Error bars represent the SE of three biological replicates. Asterisks indicate highly significant differences calculated using the t test (∗∗P < 0.01) (n = 3 for each treatment). (B–D) In situ hybridization in longitudinal sections of rhizoids. RcPLT1 expression in rhizoid tips (B) before culture on PIM, (C) after culture for 3 days, or (D) after culture for 9 days. Yellow arrows show the position of RcPLT1 expression. Bars = 2 μm.
Sustainable Overexpression of RcWUS Repressed PLB Formation
RcWUS transcription was directly affected by TDZ (Supplementary Figure S1). In tobacco (Nicotiana tabacum L.), shoots can form from root tips following overexpression of the WUS gene by applying exogenous CKs (Rashid et al., 2007). Therefore, the XVE::WUS transgenic line was used to further explore the role of RcWUS during PLB formation. In this experiment, RcWUS transcription was controlled by the β-estradiol-inducible expression system in the pER8 vector. The callus-rhizoids that originated from the three transgenic lines (#5, #8, #9) were subcultured on PIM supplemented with or without 5 mM β-estradiol. PLB formation was slightly or significantly repressed (Supplementary Table S3) by an increase in RcWUS expression (Figures 7A–C). These results suggest that continuous expression of RcWUS repressed PLB formation and that RcWUS acted as a repressor during the early stage of PLB formation.
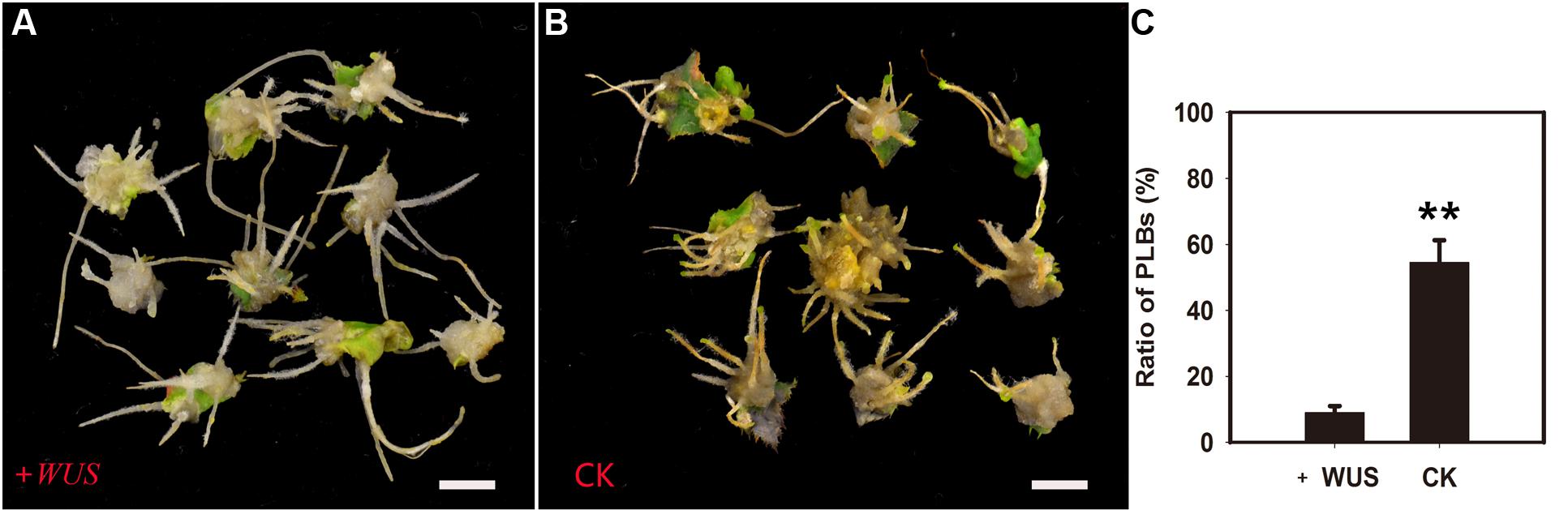
FIGURE 7. Overexpression of RcWUS had little effect on PLB formation. The leaves of XVE::WUS transgenic plants were used to produce callus-rhizoids according to Tian et al. (2008), which were then transferred to PIM that was supplemented or not with β-estradiol. (A) PLB formation was repressed by overexpression of RcWUS on PIM supplemented with 5 μM β-estradiol in transgenic line #8. (B) PLBs formed normally on PIM supplemented with 5 μM ethanol in transgenic line #8. (C) Percentage of PLB formation after culture in light for 9 days. Error bars represent the SE of three biological replicates. Asterisks indicate highly significant differences calculated using the t-test (∗∗P < 0.01) (n = 9–12 for each treatment).
Discussion
In Rosa, the process of PLB formation can be divided into three stages: early, bulking and shoot regeneration. The first 10 days are defined as the early stage in which cells of the rhizoid tip begin to divide and turn green. The bulking stage is defined as days 10–20 in which the rhizoid tip swells, the RAM disappears and multiple shoot meristem centers become established. From day 20 onward, leaf-like structures, pre-embryos, and secondary PLBs were visible, while shoot regeneration was complete after about 45 days (Liu et al., 2014). In the past several years, some research has focused on the genetic mechanisms underlying PLB formation in R. canina. RcWUS was isolated from R. canina PLBs and was ectopically expressed in tobacco resulting in shoot regeneration from in vivo leaves (Jiang et al., 2012). RcSERK1 (Xu et al., 2011), RcLEC1 (unpublished), RcBBM, and RcABI3 (Yang et al., 2014a,b), which were identified from, and expressed exclusively in R. canina PLBs, may also be involved in PLB morphogenesis.
In the hypocotyl tissue of Pelargonium X hortorum Bailey, TDZ enhanced the accumulation and translocation of auxin (Murch and Saxena, 2001). In the tissue culture of lentil (Lens culinaris Medik.) cotyledonary nodes, TDZ at a concentration lower than 2.0 μM induced shoots buts at higher concentration (2.5–15 μM), somatic embryos formed. In plant tissue culture, TDZ exhibits CK activity in bioassays and is a highly stable compound. Previous studies have suggested that TDZ can modify the metabolism of endogenous CK (Capelle et al., 1983), or mimic an auxin response (Visser et al., 1992). Our results show that TDZ promoted the accumulation of IAA and a Z-type CK, ZR (Figures 1A,B) but reduced the level of an iP-type CK, iPA (Figures 1C) in callus-rhizoids of R. canina. KT induced PLB formation but NAA repressed it (Figure 2G; Supplementary Table S4). In addition, in callus-rhizoids, both auxin and CK responses were promoted by increased expression of RcARF4 and RcRR1 while auxin translocation was repressed by decreased expression of RcPIN1, RcPIN2, and RcPIN3 (Figures 3A,B). Increased expression of RcCKX2, RcCKX3 and RcLOG1 in callus-rhizoids suggested that CK metabolism was altered by TDZ (Figures 3C,D). Moreover, application of NPA significantly promoted PLB formation (Figure 2G). Leaf-derived callus, which serves as an intermediary PLB-inducing tissue, subsequently forming rhizoids, responds directly to TDZ in PIM during PLB morphogenesis (Figures 2B,C). Consequently, these results suggest that TDZ acted mainly as a Z-type CK in the early stage (first 10 days) of PLB formation and that auxin transport was altered (Figures 3A,C).
Protocorm-like bodies originated from rhizoids tips and TDZ alone was able to trigger PLB morphogenesis. Cell fate of the RAM in rhizoid tips was converted and a SAM was established after triggering callus with TDZ. Thus, the effect of TDZ on rhizoid tips was different than that on callus-rhizoids. Our results showed that in rhizoid tips, both auxin and CK response were promoted by an increase in RcARF1, RcARF4, RcRR1, RcPIN2 and RcPIN3 expression (Figures 3A,C,D) but a decrease in the expression of the auxin transport protein gene RcPIN1 (Figure 3C). CKs affect lateral root development by modulating auxin transport protein PIN1 (Rashotte et al., 2005; Marhavý et al., 2014). RcRR1 was involved in rhizoid organogenesis in R. canina and primary root length and lateral root density increased and AtPIN1 was repressed following over-expression of RcRR1 in Arabidopsis (Gao et al., 2013). In the root elongation zone, the PIN1-dependent auxin transport stream flows into root tips through vascular tissue, and PIN2, PIN3, and PIN7 mediate auxin flow out of root tips via epidermis cells, or ‘reflux’ of auxin basipetal transport (Friml et al., 2003; Blilou et al., 2005). Our results thus imply that auxin transport toward the RAM was inhibited by reducing the expression of RcPIN1 in the vasculature whereas reverse transport was promoted by increasing the expression of RcPIN2 and RcPIN3 in epidermal cells. Furthermore, RcRR1 maybe act as a repressor of RcPIN1 transcription. These results also suggest that auxin efflux from rhizoid tips and the auxin gradient were altered by auxin transport in rhizoid tips, thereby disturbing the balance of auxin and CKs around stem cells, subsequently providing conditions that promoted the conversion of cell fate.
CKX2, CKX3 and LOG1 genes reportedly play an important role during SAM development in Arabidopsis, rice, soybean and tomato (Kurakawa et al., 2007; Yadav et al., 2009; Bartrina et al., 2011; Tamar et al., 2013; Wong et al., 2013). AtCKX2 and AtCKX3 were up-regulated by exogenous CKs (Werner et al., 2006), and transgenic Pro35S:AtCKX2 and Pro35S:AtCKX3 Arabidopsis plant lines confirmed that endogenous CK is required during auxin-induced organogenesis in vitro (Pernisová et al., 2009). In Arabidopsis, ckx3-ckx5 double mutants displayed increased WUS expression and larger SAMs (Bartrina et al., 2011). Expression of the WUS gene defines the organizing center of a SAM and WUS protein acts as a non-autonomous signal to maintain stem cells, at least in SAMs, and is sufficient to promote stem cell identity (Mayer et al., 1998; Yadav et al., 2010, 2013). In Arabidopsis, WUS expression has a complex interaction with CK signaling, biosynthesis, and degradation (Leibfried et al., 2005; Dello Ioio et al., 2008; Gordon et al., 2009; Yadav et al., 2009; Bartrina et al., 2011; Chickarmane et al., 2012). WUS represses type A-ARR inhibitors of CK signaling, thereby increasing CK signaling, which may in turn reinforce WUS expression (Leibfried et al., 2005). Dello Ioio et al. (2008) reported that the CK response factor ARR1 activates transcription of the Aux/IAA gene SHY2/IAA3 and that IAA3 protein in turns represses transcription of PIN1, resulting in a change of auxin distribution that promotes cell differentiation. In our results, the expression of RcCKX2, RcCKX3 and RcLOG1 increased in rhizoid tips, but that of RcCKX2 was more significantly increased than in callus-rhizoids (Figure 3). RcWUS can respond to TDZ (Supplementary Figure S1) and was promoted both in callus-rhizoids and rhizoid tips (Figures 4A,C). However, RcWUS expression was repressed after a transient increase in expression in rhizoid tips (Figure 5A) and the location of RcWUS expression was limited to epidermal and columella cells (Figures 5B,C,D) before the bulking stage. An increase in RcCKX2 and RcCKX3 expression (Figures 3B,D) may have resulted in repressed expression of RcWUS and degradation of an iP-type CK, iPA. Together with the increased expression of RcRR1, we deduce that TDZ caused the increased in expression of RcRR1, RcCKX2 and RcCKX3 and that this increase in expression of RcCKX2 and RcCKX3 resulted in a decrease in RcWUS expression in epidermal and columella cells.
Ectopic WUS expression can result in shoot formation from the root tip region via a β-estradiol-inducible expression system in tobacco (Rashid et al., 2007). However, exogenous CK must be supplied for root tip bulking and shoot formation. The SERK1 gene was used to indicate embryonic competence in Medicago truncatula and Arabidopsis (Hecht et al., 2001; Nolan et al., 2003). In our study, using three RcWUS transgene lines (#5, #8, #9), we found that sustained promotion of RcWUS transcription had no effect – or only a repressive effect – on PLB formation at an early stage (Figures 7A–C; Supplementary Table S3). The expression of RcSERK1 showed that TDZ induced the embryonic competence of rhizoid tip cells (Supplementary Figure S2). In addition, RcWUS expression was significantly promoted from 24 to 72 h in rhizoid tips (Figure 5A), and the level of ZR was significantly higher on day 3 (Figure 1B) than earlier and later days. These results suggest that developmental events occurred in the callus-rhizoids or rhizoid tips within the first 5 days. RcWUS expression was transiently activated in epidermal and columella cells (Figures 5B,C,D), implying that SAM identity was established before the PLB bulking stage. This may be responsible for the formation of leaf-like structures or shoots directly on the epidermis of PLBs.
In Rosa PLBs, cell fate of the RAM in rhizoid tips is converted and a SAM becomes established within callus after being triggered by TDZ. WOX5, a marker of the root stem cell niche (Sarkar et al., 2007), maintains root fate with PLT1, PLT2 (the mainly regulators of root stem cells) in Arabidopsis (Aida et al., 2004; Galinha et al., 2007). Previous research showed that shoots could be regenerated from multiple tissues through a lateral root developmental pathway in Arabidopsis, in which the root marker gene WOX5 was activated during callus induction (Sugimoto et al., 2010; Ikeuchi et al., 2013). In our study, the expression of root stem cell genes RcWOX5, RcPLT1 and RcPLT2 was reduced in callus-rhizoids but increased significantly in rhizoid tips. Accompanying the bulking stage of PLBs, we deduced that the dedifferentiation of rhizoids was triggered via an intermediate callus pathway in the early stage of PLB formation. In Arabidopsis, the root stem cell regulator PLT1 must be activated to establish the competence of shoot regeneration progenitor cells (Kareem et al., 2015). In our study, we showed that RcPLT1 was activated in root stem cell niches (Figure 6A) and then expanded to the entire rhizoid tip (Figures 6B–D). Our results of the increased expression of root stem cell-related genes (RcWOX5, RcPLT1, and RcPLT2) (Figures 4B,D and 6) suggest that the dedifferentiation pathway and de novo shoot regeneration program were activated in the center of rhizoid tips. WOX5 reportedly represses the differentiation of columella cells in Arabidopsis (Sarkar et al., 2007), so an increase in RcWOX5 expression in our study (Figure 4D) may be another cause for the decrease in RcWUS expression (Figure 5A) in epidermal and columella cells (Figures 5B–D). This is the first study to demonstrate the mechanism of conversion of a RAM to a SAM.
Our study has elaborated how TDZ triggers PLB morphogenesis via a change in the levels of endogenous auxin and CKs, and offers unique insight into the development pathway of columella cells and root stem cells during PLB formation in Rosa. The molecular mechanism of TDZ action in auxin and CK signaling, biosynthesis, and degradation was dissected, providing a better theoretical reference for using TDZ in practice, i.e., in vitro experiments in Rosa related to developmental studies. These hormones and developmental pathways intersect at multiple levels, and further studies are required to identify the direct regulator and stress signaling factors during plant regeneration in vitro induced by TDZ.
Author Contributions
YK and LZ conceived and designed the research. CY and YK conducted the experiments. YK and CY performed the assays. QZ, GL, and JN prepared the plant material. YK, CC, and ZM took the photographs and analyzed the data. JT interpreted the experimental design and data. YK, CY, SJ, JT, and LZ co-wrote the manuscript. All the authors read and approved the manuscript.
Funding
This work was supported by the National Natural Science Foundation of China [grant number 31171993].
Conflict of Interest Statement
The authors declare that the research was conducted in the absence of any commercial or financial relationships that could be construed as a potential conflict of interest.
Acknowledgments
The authors thank Prof. Zheng Meng and Feng Wu (Institute of Botany, Chinese Academy of Sciences) for their kindly assistance with in situ hybridization, and Dr. Junna He and Mr. Jian Wu (China Agriculture University) for their helpful suggestions during manuscript revision.
Supplementary Material
The Supplementary Material for this article can be found online at: http://journal.frontiersin.org/article/10.3389/fpls.2016.00557
References
Adamowski, M., and Friml, J. (2015). PIN-dependent auxin transport: action, regulation, and evolution. Plant Cell 27, 20–32. doi: 10.1105/tpc.114.134874
Aida, M., Beis, D., Heidstra, R., Willemsen, V., and Blilou, I. (2004). The PLETHORA genes mediate patterning of the Arabidopsis root stem cell niche. Cell 119, 109–120. doi: 10.1016/j.cell.2004.09.018
Bartrina, I., Otto, E., Strnad, M., Werner, T., and Schmülling, T. (2011). Cytokinin regulates the activity of reproductive meristems, flower organ size, ovule formation, and thus seed yield in Arabidopsis thaliana. Plant Cell 23, 69–80. doi: 10.1105/tpc.110.079079
Benkova, E., Michniewicz, M., Sauer, M., Teichmann, T., Seifertova, D., Jürgens, G., et al. (2003). Local, efflux-dependent auxin gradients as a common module for plant organ formation. Cell 115, 591–602. doi: 10.1016/S0092-8674(03)00924-3
Bi, L., Liu, F. L., Dong, A. X., Zhou, X. Y., Ma, N., and Zhao, L. J. (2012). Establishment of genetic transformation system in protocorm like-body (PLB) of Rosa canina. J. Nuclear Agric. Sci. 26, 270–274.
Blilou, I., Xu, J., Wildwater, M., Willemsen, V., Paponov, I., Friml, J., et al. (2005). The PIN auxin efflux facilitator network controls growth and patterning in Arabidopsis roots. Nature 433, 39–44. doi: 10.1038/nature03184
Brunoud, G., Wells, D. M., Oliva, M., Larrieu, A., Mirabet, V., Burrow, A. H., et al. (2012). A novel sensor to map auxin response and distribution at high spatio-temporal resolution. Nature 482, 103–106. doi: 10.1038/nature10791
Capelle, S. C., Mok, D. W. S., Kirclmer, S. C., and Mok, M. C. (1983). Effects of TDZ on cytokinin autonomy and the metabolism of N6-(2-isopentenyl)[8-14C] adenosine in callus tissues of Phaseolus lunatus L. Plant Physiol. 73, 796–802. doi: 10.1007/BF00195667
Casanova, E., Valdés, A. E., Fernández, B., Moysset, L., and Trillas, M. I. (2004). Levels and immunolocalization of endogenous cytokinins in thidiazuron-induced shoot organogenesis in carnation. J. Plant Physiol. 61, 95–104. doi: 10.1078/0176-1617-00957
Chang, C., and Chang, W. C. (2003). Cytokinins promotion of flowering in Cymbidium ensifolium var. misericors in vitro. Plant Growth Regul. 39, 217–221. doi: 10.1023/A:1022892402536
Chapman, E. J., and Estelle, M. (2009). Mechanism of auxin-regulated gene expression in plants. Annu. Rev. Genet. 43, 265–285. doi: 10.1146/annurev-genet-102108-134148
Chatfield, S. P., Capron, R., Severino, A., Penttila, P. A., Alfred, S., Nahal, H., et al. (2013). Incipient stem cell niche conversion in tissue culture: using a systems approach to probe early events in WUSCHEL-dependent conversion of lateral root primordial into shoot meristems. Plant J. 73, 798–813. doi: 10.1111/tpj.12085
Che, P., Lall, S., and Howell, S. H. (2007). Developmental steps in acquiring competence for shoot development in Arabidopsis tissue culture. Planta 226, 1183–1194. doi: 10.1007/s00425-007-0565-4
Chen, J. R., Liu, R., Chen, S. Y., and Wang, H. F. (2006). Plant regeneration of transgenic China rose (Rosa chinensis Jacq.) from organogenic callus. For. Stud. China 8, 92–97.
Chen, J. R., Lü, J. J., and Liu, R. (2010). DREB1C from Medicago truncatula enhances freezing tolerance in transgenic M. truncatula and China rose (Rosa chinensis Jacq.). Plant Growth Regul. 60, 199–211. doi: 10.1007/s10725-009-9434-4
Chickarmane, V. S., Gordon, S. P., Tarr, P. T., Heisler, M. G., and Meyerowitz, E. M. (2012). Cytokinin signaling as a positional cue for patterning the apical–basal axis of the growing Arabidopsis shoot meristem. Proc. Natl. Acad. Sci. U.S.A. 109, 4002–4007. doi: 10.1073/pnas.1200636109
Debener, T., and Linde, M. (2009). Exploring complex ornamental genomes: the rose as a model plant. Crit. Rev. Plant Sci. 28, 267–280. doi: 10.1080/07352680903035481
Dello Ioio, R., Nakamura, K., Moubayidin, L., Perilli, S., Taniquchi, M., Morita, M. T., et al. (2008). A genetic framework for the control of cell division and differentiation in the root meristem. Science 322, 1380–1384. doi: 10.1126/science.1164147
Fehér, A. (2015). Somatic embryogenesis – stress-induced remodeling of plant cell fate. Biochim. Biophys. Acta 1849, 385–402. doi: 10.1016/j.bbagrm.2014.07.005
Feyissa, T., Welander, M., and Negash, L. (2005). In vitro regeneration of Hagenia abyssinica (Bruce) J.F. Gmel. (Rosaceae) from leaf explants. Plant Cell Rep. 24, 392–400. doi: 10.1007/s00299-005-0949-5
Firoozabady, E., Moy, Y., Courtney-Gutterson, N., and Robinson, K. (1994). Regeneration of transgenic rose (Rosa hybrida) plants from embryogenic tissue. Biotechnology 12, 609–613. doi: 10.1007/BF00045906
Friml, J., Benková, E., Blilou, I., Wisniewska, J., Hamann, T., Ljung, K., et al. (2002a). AtPIN4 mediates sink-driven auxin gradients and root patterning in Arabidopsis. Cell 108, 661–673. doi: 10.1016/S0092-8674(02)00656-6
Friml, J., Vieten, A., Sauer, M., Weijers, D., Schwarz, H., Hamann, T., et al. (2003). Efflux-dependent auxin gradients establish the apical–basal axis of Arabidopsis. Nature 426, 147–153. doi: 10.1038/nature02085
Friml, J., Wisniewska, J., Benkova, E., Mendgen, K., and Palme, K. (2002b). Lateral relocation of auxin efflux regulator PIN3 mediates tropism in Arabidopsis. Nature 415, 806–809. doi: 10.1038/nature02085
Galinha, C., Hofhuis, H., Luijten, M., Willemsen, V., and Blilou, I. (2007). PLETHORA proteins as dose-dependent master regulators of Arabidopsis root development. Nature 449, 1053–1057. doi: 10.1038/nature06206
Gallois, J. L., Nora, F. R., Mizukami, Y., and Sablowski, R. (2004). WUSCHEL induces shoot stem cell activity and developmental plasticity in the root meristem. Genes Dev. 18, 375–380. doi: 10.1101/gad.291204
Galweiler, L., Guan, C., Müller, A., Wisman, E., Mendgen, K., Yephremov, A., et al. (1998). Regulation of polar auxin transport by AtPIN1 in Arabidopsis vascular tissue. Science 282, 2226–2230. doi: 10.1126/science.282.5397.2226
Gao, B., Fan, L. S., Li, X. X., Yang, H. F., Liu, F. L., Wang, L., et al. (2013). RcRR1, a Rosa canina type-A response regulator gene, is involved in cytokinin-modulated rhizoids organogenesis. PLoS ONE 8:e72914. doi: 10.1371/journal.pone.0072914
Gordon, S. P., Chickarmane, V. S., Ohno, C., and Meyerowitz, E. M. (2009). Multiple feedback loops through cytokinin signaling control stem cell number within the Arabidopsis shoot meristem. Proc. Natl. Acad. Sci. U.S.A. 106, 16529–16534. doi: 10.1073/pnas.0908122106
Guilfoyle, T. J., and Hagen, G. (2007). Auxin response factors. Curr. Opin. Plant Biol. 10, 453–460. doi: 10.1016/j.pbi.2007.08.014
Guilfoyle, T. J., and Hagen, G. (2012). Getting a grasp on domain III/IV responsible for Auxin Response Factor-IAA protein interactions. Plant Sci. 190, 82–88. doi: 10.1016/j.plantsci.2012.04.003
Guo, Y. C. (2009). Studies of Plant Regeneration via Protocorm-Like Body (PLB) Pathway in Rosa spp. MSc thesis, China Agricultural University, Beijing.
Hecht, V., Vielle-Calzada, J. P., Harto, M. V., Schmidt, E. D., Boutilier, K., Grossniklaus, U., et al. (2001). The Arabidopsis SOMATIC EMBRYOGENESIS RECEPTOR KINASE 1 gene is expressed in developing ovules and embryos and enhances embryogenic competence in culture. Plant Physiol. 127, 803–816. doi: 10.1104/pp.010324
Hsia, C. N., and Korban, S. S. (1996). Organogenesis and somatic embryogenesis in callus cultures of Rosa hybrid and Rosa chinensis minima. Plan Cell Tiss. Organ Cult. 44, 1–6. doi: 10.1007/BF00045906
Hwang, I., and Sheen, J. (2001). Two-component circuitry in Arabidopsis cytokinin signal transduction. Nature 413, 383–389. doi: 10.1038/35096500
Hwang, I., Sheen, J., and Müller, B. (2012). Cytokinin signaling networks. Annu. Rev. Plant Biol. 63, 353–380. doi: 10.1146/annurev-arplant-042811-105503
Ikeuchi, M., Sugimoto, K., and Iwase, A. (2013). Plant callus: mechanisms of induction and repression. Plant Cell 25, 3159–3173. doi: 10.1105/tpc.113.116053
Jiang, F. X., Liu, F. L., Ma, N., Zhang, J. F., and Zhao, L. J. (2012). Constitutive expression of RcWUS gene in Rosa canina on morphological alteration of transgenic tobacco leaves. J. Nucl. Agric. Sci. 26, 262–269.
Jones, B., Gunnereas, S. A., Petersson, S. V., Tarkowski, P., Graham, N., May, S., et al. (2010). Cytokinin regulation of auxin synthesis in Arabidopsis involves a homeostatic feedback loop regulated via auxin and cytokinin signal transduction. Plant Cell 22, 2956–2969. doi: 10.1105/tpc.110.074856
Kareem, A., Durgaprasad, K., Sugimoto, K., Du, Y. J., Pulianmackal, A. J., Trivedi, Z. B., et al. (2015). PLETHORA genes control regeneration by a two-step mechanism. Curr. Biol. 25, 1–14. doi: 10.1016/j.cub
Kim, C. K., Chung, J. D., Park, S. H., Burrell, A. M., Kamo, K. K., and Byrne, D. H. (2004). Agrobacterium tumefaciens-mediated transformation of Rosa hybrida using the green fluorescent protein (GFP) gene. Plant Cell Tissue Organ Cult. 78, 107–111. doi: 10.1023/B:TICU.0000022529.16697.90
Kim, S. W., Swung, C. O., Dong, S. I., and Liu, J. R. (2003). Plant regeneration of rose (Rosa hybrida) from embryogenic cell-derived protoplasts. Plant Cell Tissue Organ Cult. 73, 15–19. doi: 10.1023/A:1022693605436
Kurakawa, T., Ueda, N., Maekawa, M., Kobayashi, K., Kojima, M., Nagato, Y., et al. (2007). Direct control of shoot meristem activity by a cytokinin-activating enzyme. Nature 445, 652–655. doi: 10.1038/nature05504
Leibfried, A., To, J. P., Busch, W., Stehling, S., and Kehle, A. (2005). WUSCHEL controls meristem function by direct regulation of cytokinin-inducible response regulators. Nature 438, 1172–1175. doi: 10.1038/nature04270
Li, X. Q., Krasnyanski, S. F., and Korban, S. S. (2002). Somatic embryogenesis, secondary somatic embryogenesis, and shoot organogenesis in Rosa. J. Plant Physiol. 159, 313–319. doi: 10.1078/0176-1617-00688
Liu, F. L., Abdurazak, I., Xi, L., Gao, B., Wang, L., Tian, C. W., et al. (2014). Morphohistological analysis of the origin and development of Rosa canina protocorm-like bodies. Sci. Hortic. 167, 107–116. doi: 10.1016/j.scienta.2014.01.001
Livak, K. J., and Schmittgen, T. D. (2001). Analysis of relative gene expression data using real-time quantitative PCR and the 2-ΔΔCT method. Methods 25, 402–408. doi: 10.1006/meth.2001.1262
Marhavý, P., Duclercq, J., Weller, B., Feraru, E., Bielach, A., Offringa, R., et al. (2014). Cytokinin controls polarity of PIN1-dependent auxin transport during lateral root organogenesis. Curr. Biol. 24, 1031–1037. doi: 10.1016/j.cub.2014.04.002
Mayer, K. F., Schoof, H., Haecker, A., Lenhard, M., Jürgens, G., and Laux, T. (1998). Role of WUSCHEL in regulating stem cell fate in the Arabidopsis shoot meristem. Cell 95, 805–815. doi: 10.1016/S0092-8674(00)81703-1
Müller, A., Guan, C., Gälweiler, L., Tänzler, P., Huijser, P., Marchant, A., et al. (1998). AtPIN2 defines a locus of Arabidopsis for root gravitropism control. EMBO J. 17, 6903–6911. doi: 10.1093/emboj/17.23.6903
Murashige, T., and Skoog, F. (1962). A revised method for rapid growth and bioassays with tobacco tissue cultures. Physiol. Plant. 15, 472–497. doi: 10.1111/j.1399-3054.1962.tb08052.x
Murch, S. J., and Saxena, P. K. (2001). Molecular fate of thidiazuron and its effects on auxin transport in hypocotyls tissues of Pelargonium × hortorum Bailey. Plant Growth Regul. 35, 269–275. doi: 10.1023/A:1014468905953
Nolan, K., Irwanto, R. R., and Rose, R. J. (2003). Auxin up-regulates MtSERK1 expression in both Medicago truncatula root-forming and embryogenic cultures. Plant Physiol. 133, 218–230. doi: 10.1104/pp.103.020917
Pati, P. K., Rath, S. P., Sharma, M., Sood, A., and Ahuja, P. S. (2006). In vitro propagation of rose – a review. Biotechnol. Adv. 24, 94–114. doi: 10.1016/j.biotechadv.2005.07.001
Pernisová, M., Klíma, P., Horák, J., Válková, M., Malbeck, J., Soucek, P., et al. (2009). Cytokinins modulate auxin-induced organogenesis in plants via regulation of the auxin efflux. Proc. Natl. Acad. Sci. U.S.A. 106, 3609–3614. doi: 10.1073/pnas.0811539106
Randoux, M., Davière, J.-M., Jeauffre, J., Thouroude, T., Pierre, S., Toualbia, Y., et al. (2014). RoKSN, a floral repressor, forms protein complexes with RoFD and RoFT to regulate vegetative and reproductive development in rose. New Phytol. 202, 161–173. doi: 10.1111/nph.12625
Rashid, S. Z., Yamaji, N., and Kyo, M. (2007). Shoot formation from root tip region: a developmental alternation by WUS in transgenic tobacco. Plant Cell Rep. 26, 1449–1455. doi: 10.1007/s00299-007-0342-7
Rashotte, A. M., Chae, H. S., Maxwell, B. M., and Kieber, J. J. (2005). The interaction of cytokinin with other signals. Physiol. Plant. 123, 184–194. doi: 10.1111/j.1399-3054.2004.00445.x
Robert, H. S., Grones, P., Stepanova, A. N., Robles, L. M., Lokerse, A. S., Alonso, J. M., et al. (2013). Local auxin sources orient the apical-basal axis in Arabidopsis embryos. Curr. Opin. Plant Biol. 23, 2506–2512. doi: 10.1016/j.cub.2013.09.039
Sakai, H., Honma, T., Aoyama, T., Sato, S., Kato, T., Tabata, S., et al. (2001). ARR1, a transcription factor for genes immediately responsive to cytokinins. Science 294, 1519–1521. doi: 10.1126/science.1065201
Sarkar, A., Luijten, M., Miyashima, S., Lenhard, M., and Hashimoto, T. (2007). Conserved factors regulate signalling in Arabidopsis thaliana shoot and root stem cell organizers. Nature 446, 811–814. doi: 10.1038/nature05703
Schoof, H., Lenhard, M., Haecker, A., Mayer, K. F., Jürgens, G., and Laux, T. (2000). The stem cell population of Arabidopsis shoot meristems is maintained by a regulatory loop between the CLAVATA and WUSCHEL genes. Cell 100, 635–644. doi: 10.1016/S0092-8674(00)80700-X
Sugimoto, K., Jiao, Y., and Meyerowitz, E. M. (2010). Arabidopsis regeneration from multiple tissues occurs via a root development pathway. Dev. Cell 18, 463–471. doi: 10.1016/j.devcel.2010.02.004
Takei, K., Sakakibara, H., Taniguchi, M., and Sugiyama, T. (2001). Nitrogen-dependent accumulation of cytokinins in root and the translocation to leaf: implication of cytokinin species that induces gene expression of maize response regulator. Plant Cell Physiol. 42, 85–93. doi: 10.1093/pcp/pce009
Tamar, E. R., Akiva, S. K., Louise, C. M., Ziva, A., Yuval, E., and Eliezer, L. (2013). A cytokinin-activating enzyme promotes tuber formation in tomato. Curr. Opin. Plant Biol. 23, 1057–1064. doi: 10.1016/j.cub.2013.04.061
Tan, X., Calderon-Villalobos, L. I., Sharon, M., Zheng, C., Robinson, C. V., Estelle, M., et al. (2007). Mechanism of auxin perception by the TIR1 ubiquitin ligase. Nature 446, 640–645. doi: 10.1038/nature05731
Tanaka, Y., Katsumoto, Y., Brugliera, F., and Mason, J. (2005). Genetic engineering in floriculture. Plant Cell Tissue Organ Cult. 80, 1–24. doi: 10.1007/s11240-004-0739-8
Teixeira da Silva, J. A. (2014). Should the term protocorm-like body be used exclusively for orchids? J. Plant Dev. 21, 161–166.
Thomas, J. C., and Katterman, F. R. (1986). Cytokinin activity induced by thidiazuron. Plant Physiol. 81, 681–683. doi: 10.1104/pp.81.2.681
Tian, C. W., Chen, Y., Zhao, X. L., and Zhao, L. J. (2008). Plant regeneration through protocorm-like bodies induced from rhizoids using leaf explants of Rosa spp. Plant Cell Rep. 27, 823–831. doi: 10.1007/s00299-007-0504-7
Visser, C., Qureshi, J. A., Gill, R., and Saxena, P. K. (1992). Morphoregulatory role of thidiazuron. Plant Physiol. 99, 1704–1707. doi: 10.1104/pp.99.4.1704
Wabnik, K., Robert, H. S., Smith, R. S., and Friml, J. (2013). Modeling framework for the establishment of the apical-basal embryonic axis in plants. Curr. Opin. Plant Biol. 23, 2513–2518. doi: 10.1016/j.cub.2013.10.038
Wang, Y., Li, B., Du, M., Eneji, A. E., Wang, B., Duan, L., et al. (2012). Mechanism of phytohormone involvement in feedback regulation of cotton leaf senescence induced by potassium deficiency. J. Exp. Bot. 63, 5887–5901. doi: 10.1093/jxb/ers238
Weiler, E. W., Jourdan, P. S., and Conrad, W. (1981). Level of indole-3-acetic acid in intact and decapitated coleoptiles as determined by a specific and highly sensitive solid phase enzyme immunoassay. Planta 152, 561–571. doi: 10.1007/BF00385542
Werner, T., Köllmer, I., Bartrina, I., Holst, K., and Schmülling, T. (2006). New insights into the biology of cytokinin degradation. Plant Biol. 8, 371–381. doi: 10.1055/s-2006-923928
Werner, T., and Schmülling, T. (2009). Cytokinin action in plant development. Curr. Opin. Plant Biol. 12, 527–538. doi: 10.1016/j.pbi.2009.07.002
Wong, C. E., Singh, M. B., and Bhalla, P. L. (2013). Spatial expression of CLAVATA3 in the shoot apical meristem suggests it is not a stem cell marker in soybean. J. Exp. Bot. 68, 5641–5649. doi: 10.1093/jxb/ert341
Xu, K. D., Liu, Q. L., Yang, H. F., Zeng, L., Dong, L. L., Liu, F. L., et al. (2011). Isolation and molecular characterization of RcSERK1: a Rosa canina gene transcriptionally induced during initiation of protocorm-like bodies. Afr. J. Biotechnol. 10, 4011–4017. doi: 10.5897/AJB10.1520
Yadav, R. K., Girke, T., Pasala, S., Xie, M., and Reddy, G. V. (2009). Gene expression map of the Arabidopsis shoot apical meristem stem cell niche. Proc. Natl. Acad. Sci. U.S.A. 106, 4941–4946. doi: 10.1073/pnas.0900843106
Yadav, R. K., Perales, M., Gruel, J., Ohno, C., Heisler, M., Girke, T., et al. (2013). Plant stem cell maintenance involves direct transcriptional repression of differentiation program. Mol. Syst. Biol. 9, 654. doi: 10.1038/msb.2013.8
Yadav, R. K., Tavakkoli, M., and Reddy, G. V. (2010). WUSCHEL mediates stem cell homeostasis by regulating stem cell number and patterns of cell division and differentiation of stem cell progenitors. Development 137, 3581–3589. doi: 10.1242/dev.054973
Yang, H. F., Kou, Y. P., Gao, B., Soliman, T. M. A., Xu, K. D., Ma, N., et al. (2014a). Identification and functional analysis of BABY BOOM genes from Rosa canina. Biol. Plant. 58, 427–435. doi: 10.1007/s10535-014-0420-y
Yang, H. F., Xu, K. D., Ishak, A., Li, J. X., Liu, F. L., Ma, N., et al. (2014b). Molecular cloning and functional analysis of ABI3 from Rosa canina. Pak. J. Bot. 46, 803–810.
Yang, J. C., Zhang, J. H., Wang, Z. Q., Zhu, Q. S., and Wang, W. (2001). Hormonal changes in the grains of rice subjected to water stress during grain filling. Plant Physiol. 127, 315–323. doi: 10.1104/pp.127.1.315
Zhang, Y., Peng, L., Wu, Y., Shen, Y. Y., Wu, X., and Wang, J. (2014). Analysis of global gene expression profiles to identify differentially expressed genes critical for embryo development in Brassica rapa. Plant Mol. Biol. 86, 425–442. doi: 10.1007/s11103-014-0238-1
Keywords: auxin, cytokinin, PLB, rhizoids, Rosa canina, stem cell, TDZ
Citation: Kou YP, Yuan CQ, Zhao QC, Liu GQ, Nie J, Ma ZM, Cheng CX, Teixeira da Silva JA and Zhao LJ (2016) Thidiazuron Triggers Morphogenesis in Rosa canina L. Protocorm-Like Bodies by Changing Incipient Cell Fate. Front. Plant Sci. 7:557. doi: 10.3389/fpls.2016.00557
Received: 12 January 2016; Accepted: 11 April 2016;
Published: 04 May 2016.
Edited by:
Lin Xu, Shanghai Institutes for Biological Sciences, ChinaReviewed by:
Songjun Zeng, Chinese Academy of Sciences, ChinaDeyang Xu, University of Copenhagen, Denmark
Copyright © 2016 Kou, Yuan, Zhao, Liu, Nie, Ma, Cheng, Teixeira da Silva and Zhao. This is an open-access article distributed under the terms of the Creative Commons Attribution License (CC BY). The use, distribution or reproduction in other forums is permitted, provided the original author(s) or licensor are credited and that the original publication in this journal is cited, in accordance with accepted academic practice. No use, distribution or reproduction is permitted which does not comply with these terms.
*Correspondence: Liangjun Zhao, emhhb2xqNTA3M0BzaW5hLmNvbQ==