- 1Laboratory of Plant Physiology, Department of Natural Products, Plant Biology and Soil Science, Faculty of Pharmacy, University of Barcelona, Barcelona, Spain
- 2Centro para Investigaciones en Granos y Semillas, Universidad de Costa Rica, San José, Costa Rica
Guazatine is a potent inhibitor of polyamine oxidase (PAO) activity. In agriculture, guazatine is used as non-systemic contact fungicide efficient in the protection of cereals and citrus fruits against disease. The composition of guazatine is complex, mainly constituted by a mixture of synthetic guanidated polyamines (polyaminoguanidines). Here, we have studied the effects from exposure to guazatine in the weed Arabidopsis thaliana. We report that micromolar concentrations of guazatine are sufficient to inhibit growth of Arabidopsis seedlings and induce chlorosis, whereas germination is barely affected. We observed the occurrence of quantitative variation in the response to guazatine between 107 randomly chosen Arabidopsis accessions. This enabled us to undertake genome-wide association (GWA) mapping that identified a locus on chromosome one associated with guazatine tolerance. CHLOROPHYLLASE 1 (CLH1) within this locus was studied as candidate gene, together with its paralog (CLH2). The analysis of independent clh1-2, clh1-3, clh2-3, clh2-2, and double clh1-2 clh2-3 mutant alleles indicated that CLH1 and/or CLH2 loss-of-function or expression down-regulation promote guazatine tolerance in Arabidopsis. We report a natural mechanism by which Arabidopsis populations can overcome toxicity by the fungicide guazatine.
Introduction
Arabidopsis thaliana (thereafter referred to as Arabidopsis) is a small weed mainly distributed in the northern hemisphere. It grows in open or recently disturbed habitats and its spread was facilitated by the expansion of agriculture (François et al., 2008). Arabidopsis exhibits extensive natural variation for different developmental, abiotic and biotic stress resistance traits (Koornneef et al., 2004; Alonso-Blanco et al., 2009; Atwell et al., 2010). Understanding the genetic bases for such variation enables the identification of potential mechanisms underlying local adaptation. Here, we have used genome-wide association studies (GWAS) to identify genes contributing to the natural variation in guazatine tolerance observed in this species. Multiple recombination events in the genetic history of populations produce close linkage disequilibrium (LD) of markers with causal loci for certain phenotypes. Such associations can be detected through GWAS. These type of approaches require the genetic validation of associations and have some limitations compared to, for example, QTL mapping (Korte and Farlow, 2013). In Arabidopsis, GWAS has been successfully applied to uncover the genetics of multiple traits (Atwell et al., 2010; Baxter et al., 2010; Li et al., 2010; Chan et al., 2011; Chao et al., 2012; Filiault and Maloof, 2012). Furthermore, the use of natural variation as source of genetic variability enables the analysis of how naturally occurring alleles evolve and may be selected (Alonso-Blanco et al., 2009).
Guazatine is a non-systemic, contact-based, aliphatic nitrogen fungicide used in agriculture that protects cereals against different diseases such as common bunt (Tilletia ssp.), common root rot (Helminthosporium), seedling blight (Fusarium ssp.), glume blotch (Septoria), and smut (Ustilago; Dreassi et al., 2007). In citrus fruits, guazatine also protects from infection by sour rot (Geotrichum candidum), green mold (Penicillium digitatum), and blue mold (Penicillium italicum; Wild, 1983). The mode of action of guazatine, at least in the ascomycete Alternaria, is inhibition of lipid biosynthesis and membrane destabilization (Yagura et al., 1984). The composition of guazatine is complex and constituted by a mixture of guanidated polyamines (PAs) referred to as polyaminoguanidines (PAGs). Most abundant PAGs in guazatine are diamines [octamethylenediamine H2N-(CH2)8-NH2] and triamines [iminodi(octamethylene)diamine H2N-(CH2)8-NH-(CH2)8-NH2], but also guanidated tetramines and carbamonitrile. In plants, guazatine is a potent inhibitor of PA oxidase activity (PAO) that has been extensively used to block PA oxidation or PA back-conversion in different species, thus contributing to decipher the biological functions of PAO in plants in relation to H2O2 production and ROS signaling (Federico et al., 2001; Yoda et al., 2006; Marina et al., 2008; Moschou et al., 2008; Fincato et al., 2011; Agudelo-Romero et al., 2014). The long alkyl chains, secondary amino groups and guanidine groups of PAGs constitute the structural requirements for the inhibition of PAO activity by guazatine (Cona et al., 2004). Despite its use in agriculture as fungicide, little is known about the physiological effects from long-term exposure to guazatine in weeds, such as Arabidopsis. We find that guazatine concentrations as low as 2.5 μM inhibit Arabidopsis shoot and root growth, and reduce total chlorophyll levels. We identified the occurrence of quantitative variation in response to guazatine in 107 natural Arabidopsis accessions from Europe and America. We performed genome-wide association mapping to determine the genetic bases for the variation observed. GWAS identified associations between guazatine tolerance and allelic variation at CHLOROPHYLLASE 1 (CLH1), encoding an enzyme that catalyzes the hydrolysis of the ester bond of chlorophyll producing chlorophyllide and phytol (Hörtensteiner, 2006). CLH1 and its paralog CLH2, were further validated for this association. The isolation and analysis of chl1-2, clh1-3, chl2-2, clh2-3, and double clh1-2 clh2-3 mutant alleles confirmed that CLH1 or CLH2 loss-of-function promote guazatine-tolerance in Arabidopsis. We conclude that a natural mechanism occurs which provides tolerance to guazatine in natural populations, involving enzymes in the chlorophyll degradation pathway.
Materials and Methods
Plant Materials and Growth Conditions
Accessions used in this work were obtained from the Nottingham Arabidopsis Stock Centre (NASC, www.arabidopsis.info) or kindly provided by Prof. Maarten Koornneef (Max Planck Institute for Plant Breeding Research, Cologne, Germany). A complete list of Arabidopsis accessions, origins and accession numbers is detailed in Table S1. Seed sterilization was performed by vigorous shaking of seeds in an aqueous solution containing 30% sodium hypochlorite supplemented with 0.5% TritonX-100 for 10 min, followed by three washes with sterile deionized H2O. For in vitro culture, sterilized seeds were sown on Growth Media (GM: 0.5 x Murashige & Skoog supplemented with vitamins, 1% sucrose, 0.8% Plant Agar (Duchefa Biochemie), pH 5.7 adjusted with KOH). Seeds were stratified in the dark at 4°C during 2–4 days. Seedlings were grown under 12 h dark/12 h light cycles at 20/22°C, 100–125 μmol photons m−2 s−1 of light intensity.
Isolation and Characterization of clh1-2, clh1-3, clh2-2, clh2-3 Double clh1-2 clh2-3 Mutants
clh1 and clh2 T-DNA insertion mutants were obtained from NASC (clh1-2, N653869; clh1-3, N871333; clh2-2, N827897; and clh2-3, N668619). Confirmation of the T-DNA insertion position and isolation of homozygous lines was performed by PCR-based genotyping and sequencing from genomic DNA, using T-DNA (LB) primer (5′-GCG TGGACCGCTTGCTGCAACT) and gene specific primers: clh1-2 (Fwd: 5′-TTTGTTAGTTCCTGCGACTGG and Rev: 5′-AGA GAGAGAGACGGAGGTTGG), clh1-3 (Fwd: 5′-CACATACAACCGGCC ATAAAC and Rev: 5′-GAA AAATCAACATTCTCCCCC), clh2-3 (Fwd: 5′-CGGATAATCTCCTTC CTCCAC and Rev: 5′-ACA AAGCCCATTCCTTGTACC), clh2-2 (Fwd: 5′-GAGGGTGGAGAG AATTTGAGG and Rev: 5′ GTCGCCTTAAAGAAATTTGGG). Genomic DNA was extracted using DNeasy plant mini Kit (Qiagen) according to manufacturer's instructions. PCR conditions were as follows: 95°C 5 min, 30 cycles (95°C 15 s, 55°C 45 s, 72°C 2 min), 72°C 10 min.
The double homozygous clh1-2 clh2-3 mutant was isolated by genotyping 48 F2 plants derived from the cross of the respective parental lines with primers described above. Expression of CLH1 and CLH2 was determined by RT-PCR. Briefly, total RNA isolated from 7-days old seedlings was extracted using TRIzol reagent (Invitrogen). Two micrograms of RNA was treated with DNAse I (Invitrogen) and first strand cDNA synthesized using Superscript II (Invitrogen) and oligo dT. One microliter of cDNA was used for PCR amplification of CLH1 (Fwd: 5′-TTACATTCTTGTAGC CCCAC, Rev: 5′-GCG ACTGGATCAATTCCTAT) or CLH2 (Fwd: GCTTATGTTGCATGTCTCT, Rev: CGAGGAGTA CCCAAATTTCT) with LA Taq DNA polymerase (Takara) using the following PCR conditions: 95°C 5 min, 30 cycles (95°C 15 s, 55°C 45 s, 68°C 1 min), 68°C 10 min.
Guazatine Treatments
Guazatine acetate was obtained from KenoGard (Stockholm). Sterilized seeds of Arabidopsis accessions were sown directly on GM supplemented with or without 2.5 μM guazatine. Chlorophyll levels were determined 16 days after germination.
clh mutants were germinated and grown on a nylon mesh (43 μm) placed on top of the GM media. Four days after germination, the nylon mesh was transferred to GM supplemented with 5 μM guazatine. Samples for chlorophyll extraction were harvested 12 days after guazatine treatment.
Quantification of Chlorophyll Levels
Seedlings were harvested individually, weighted and placed in 2 ml tubes (Eppendorf safe-lock) in the presence of 100 μl of borosilicate beads (Ø 4 mm), submerged in liquid nitrogen and homogenized with Star-Beater device (VWR International). Buffered acetone (acetone/Tris-HCl 80:20 vol, pH 7.8) was added in a ratio of 1 ml per 20 mg fresh weight (FW). Samples were incubated in the dark at 70°C during 10 min and centrifuged at 12,000 rpm for 1 min. Chlorophylls were determined using UV2310 Spectrophotometer (DINKO Industries) at 663 nm for chlorophyll A and 645 nm for chlorophyll B. Chlorophyll levels were calculated according to Porra (2002).
Transmission Electron Microscopy
Fifteen randomly chosen leaf segments from guazatine-treated and untreated leaves, were cut into pieces of 5 mm length and fixed in a solution of 2% glutaraldehyde in 2.5% cacodylate buffer pH 7.4 (CB) at 4°C. The segments were washed five times for 10 min in CB and post-fixed for 2 h 15 min in a solution of 1% OsO4 and 0.8% FeCNK (w/v). After five additional washes with distilled H2O at 4°C, samples were dehydrated in acetone and embedded in Spurr Low-Viscosity Embedding kit (Sigma-Aldrich). Serial ultrathin sections (60–70 nm) were obtained using an ultramicrotome (Reichert-Jung, Wien, Austria), collected on 200 mesh uncoated copper grids and stained with 2% uranyl acetate and Reynolds lead citrate. Samples were observed under a TEM Bioscan Gatan, JEOL 1010 at the Scientific and Technological Centers (CCiT) of the University of Barcelona.
GWA Mapping
GWAS was performed using the GWAPP web interface (Seren et al., 2012). Mean chlorophyll values of 107 Arabidopsis accessions grown under control and guazatine conditions (as indicated above), were transformed using the square root. GWAS was conducted using the accelerated mixed model (AMM), and linear regression (LM; Seren et al., 2012). To correct for multiple testing, a Bonferroni correction with a threshold of 0.5 was performed. P-value bias due to population stratification was evaluated with Q–Q plots. The LD was visualized in the flanking region of the CLH1 gene (between 6.74 and 6.87 Mb on chromosome 1).
Root and Biomass Measurements
For root measurements, 4-days old seedlings germinated on GM were transferred into GM plates containing 1.5 μM guazatine. Plates were placed vertically and root measurements determined after 12 days of guazatine treatment using the SmartRoot software (Lobet et al., 2011).
Quantification of Free PAs
PAs from plant material were extracted using 5% (v/v) perchloric acid (PCA, 1 ml per 200 mg of fresh weight). Samples were vortexed vigorously, incubated on ice during 5 min and centrifuged at 16,000 g 10 min at 4°C. 200 μl of the PCA supernatant were taken for dansyl derivatization and detection according to Marcé et al. (1995).
Expression Analyses
The expression of CLH1 (At1g19670) and CLH2 (At5g43860) in Arabidopsis accessions was obtained from microarray data deposited in Genevestigator under experiment IDs AT-00283 and AT-00407 (Hruz et al., 2008). Expression values from three independent biological replicates were normalized to UBIQUITIN 10 (AT4G05320) and expressed relative to Col-0 accession.
Phylogenetic and Statistical Analyses
DNA sequences were obtained from the 1001 Genomes project (www.1001genomes.org). NJ tree was computed using MEGA6.06. Statistical analyses were performed using SPSS software v.22 (IBM SPSS Statistics, IBM, Chicago, IL).
Results
Effects of Long-Term Exposure to Guazatine in Arabidopsis
Due to the use of polyaminoguanidines (guazatine) as fungicide in agriculture, we studied the effects of guazatine treatment in germination and growth of the weed Arabidopsis. Exposure of Arabidopsis (Col-0) to increasing concentrations of guazatine from 0 to 25 μM did not affect germination (Figure 1A). Conversely, treatment with guazatine inhibited growth of Arabidopsis seedlings, produced chlorosis and affected chloroplast integrity (Figures 1A–C). Accumulation of osmophilic bodies that resembled plastoglobules was observed in chloroplasts of guazatine-treated leaves (Figure 1C). Guazatine concentrations as low as 2.5 μM were sufficient to inhibit growth in different Arabidopsis accessions, whereas chlorosis exhibited a dose-dependent response depending on the accession (Figures 1A,B). We concluded that long-term exposure to μM concentrations of guazatine is detrimental for Arabidopsis growth and reduces chlorophyll levels, for which quantitative variation between accessions was observed.
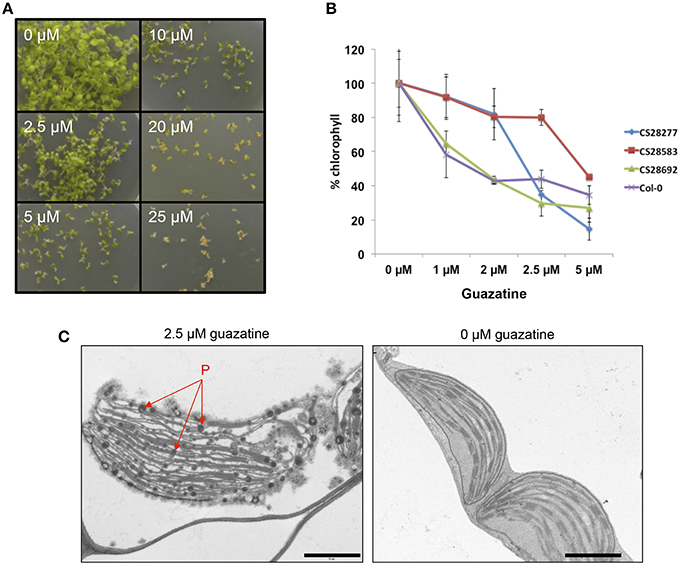
Figure 1. Effects of guazatine treatment in Arabidopsis germination, growth and chlorophyll content. (A) Phenotype of 12-days-old Arabidopsis seedlings germinated and grown under different concentrations of guazatine from 0 to 25 μM. (B) Quantitation of chlorophyll loss by guazatine treatment in four genetically different accessions (CS28277, Ge-1; CS28583, Old-1; CS28692, Rou-0; Col-0). Values are normalized to chlorophyll levels at 0 μM guazatine for every genotype and expressed as %. Values are the mean from at least five independent biological replicates ±SD. (C) TEM images of 12-days-old wild-type Col-0 seedlings treated with 2.5 μM guazatine (+) or 0 μM guazatine (−). P, plastoglobules. Scale bar: 1 μM.
Quantitative Variation of Chlorophyll Levels in Response to Guazatine in 107 Arabidopsis Accessions
We selected 2.5 μM for the quantitative analysis of the natural variation in response to guazatine in 107 Arabidopsis accessions originally collected from worldwide. Higher concentrations were lethal for most natural accessions, whereas 2.5 μM guazatine was optimal to generate a large degree of phenotypic variation (Figures 2A,B and Table S1). We determined total chlorophyll levels as proxy for the quantification of guazatine tolerance traits. Quantification of chlorophyll in guazatine treated and untreated seedlings evidenced the occurrence of quantitative variation for this trait, with some accessions exhibiting high sensitivity and others increased tolerance to the fungicide (Figure 2B). Guazatine resistant and sensitive accessions were evenly distributed in populations from Austria, Czech Republic, France, Germany, The Netherlands, Portugal, UK and USA, with a high frequency of guazatine tolerance in accessions from Germany. We concluded that guazatine tolerant and sensitive accessions are not geographically restricted. Their distributions do not exhibit evident population patterns, although the frequencies of tolerant and sensitive accessions vary between populations (Figure 2B).
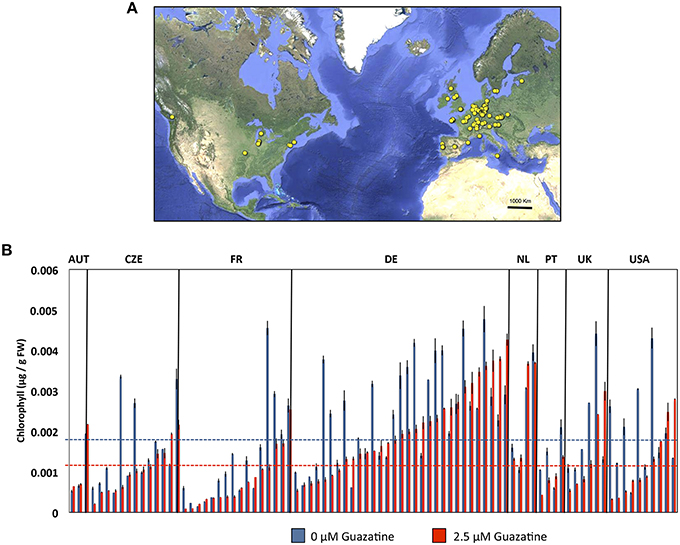
Figure 2. (A) Geographical distribution of Arabidopsis accessions used in this work. The origins of accessions are indicated in yellow spots and detailed in Table S1. (B) Levels of chlorophylls in Arabidopsis accessions grown in the presence of 2.5 or 0 μM guazatine for 12 days. Accessions were sorted according to populations and guazatine tolerance. Only populations for which four or more individuals were available are shown. Values are the mean of at least five biological replicates ± SD.
GWAS Analysis for Chlorophyll Levels in Response to Guazatine
GWAS was conducted to identify genetic factors underlying the response to guazatine in Arabidopsis natural populations using chlorophyll levels. The GWAS profiles showed a complex regulation of guazatine tolerance using both the accelerated mixed model (AMM) and linear regression (LM) methods (Figures 3A,B; Seren et al., 2012). Confounding due to population structure between both methods was assessed using Q–Q plots (Figure S1). The AMM method presented lower deviation from the identity line than the LM method, indicating an efficient control for population structure (Figure S1). Several strong associations were identified on the top of chromosome one between 6.74 and 6.87 Mb (Figure 3B) using the LM method. Remarkably, this association was absent under control conditions (Figure S2). The difference between methods seems to be due to the correction for population structure. The risk of P-value overcorrection is absent in the LM method when applied to traits correlated with population structure. Considering the advantages and disadvantages of both methods, we investigated potential gene candidates obviously associated with the variation of chlorophyll content within the associated region. CHLOROPHYLLASE 1 (CLH1, At1g19670), involved in the chlorophyll degradation pathway (Hörtensteiner, 2006), is located in the associated region on chromosome one (Figure 3C). Pairwise linkage disequilibrium (LD) between SNPs for this region indicated LD values higher than 0.4 near CLH1 (Figure 3D), denoting strong LD. The CLH1 gene has one gene paralog, CLH2 (At5g43860), located on chromosome 5 for which associations could not be detected regardless of the method (Figures 3A,B and Figure S3). We concluded that CLH1 was an obvious candidate for gene validation studies.
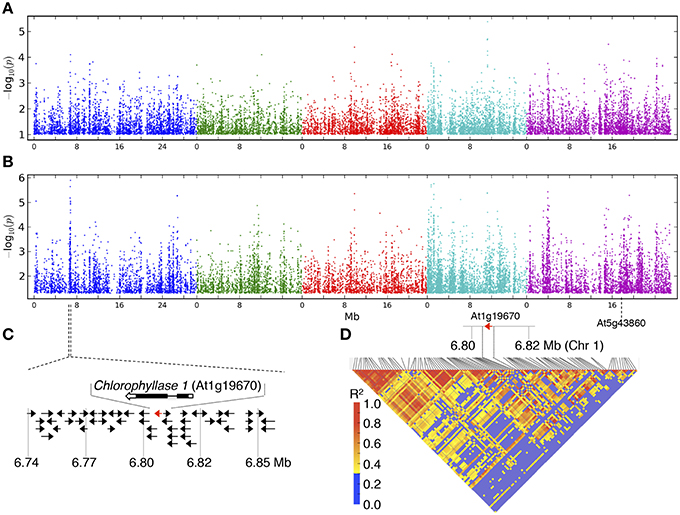
Figure 3. GWA mapping for guazatine tolerance in 107 Arabidopsis accessions. GWA mapping profiles using the (A) accelerated mixed model (AMM) and (B) linear regression (LM) methods. Chromosomes are shown in different colors. (C) Schematic representation of the CLH1 flanking region. (D) LD triangle plot for the CLH1 genomic region.
Characterization of clh1 and clh2 Mutants in Response to Guazatine Treatment
We isolated clh1 (clh1-2 and clh1-3) and clh2 (clh2-2 and clh2-3) T-DNA insertion mutants that exhibited reduced or no expression of CLH1 and CLH2 genes, respectively, (Figure S4). In agreement with that previously reported for clh1-1, clh2-1, and clh2-2, mutants (Schenk et al., 2007), clh1-2, clh1-3, and clh2-3 in this work did not show visually evident phenotypes on development or natural senescence differing from wild-type plants. We tested the tolerance of these genotypes to 5 μM guazatine, which is twice the concentration at which most Arabidopsis wild-type accessions, including Col-0, exhibited susceptibility (Figure 1B). Remarkably, loss of chlorophyll and growth inhibition induced by 5 μM guazatine treatment was significantly attenuated in clh1-2, clh1-3, clh2-2, and clh2-3 seedlings compared to the wild-type (Figures 4A,B). This indicated that CLH1 and/or CLH2 loss-of-function or expression down-regulation enhances guazatine tolerance. The double clh1-2 clh2-3 mutant exhibited higher chlorophyll and biomass in the presence of guazatine than single clh1-2, clh1-3, or clh2-2, clh2-3 mutants, which is consistent with an additive effect by individual mutations (Figures 4A,B). In the root system, we observed that guazatine concentrations as low as 1.5 μM inhibited primary root elongation in wild-type Arabidopsis seedlings and this response was attenuated in clh1-2 and clh1-3 but not so significantly in clh2-2 or clh2-3 (Figure 4C and Figure S5). The lower dosage required for root growth inhibition might be due to direct uptake by root cells without the need of transport. The double clh1-2 clh2-3 responded similarly to clh1 loss of function (Figure 4C). We concluded that CLH1 and/or CLH2 loss-of-function or their down-regulation promote guazatine tolerance in Arabidopsis.
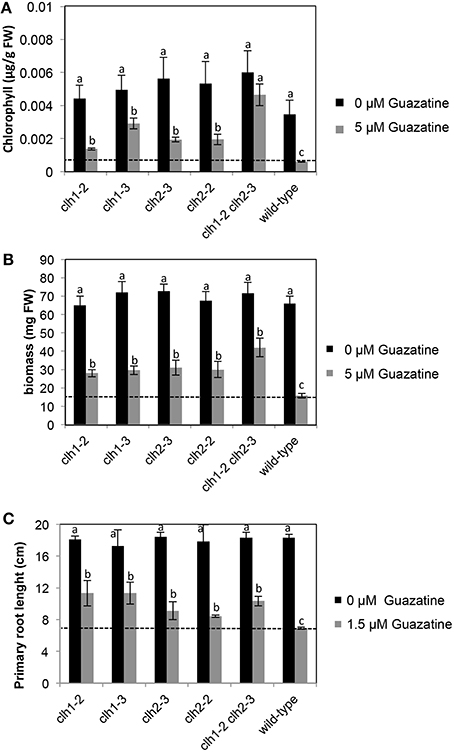
Figure 4. Chlorophyll levels (A), biomass (B) and primary root length (C) in clh, clh2 and double clh1 clh2 mutants grown in the presence of 5 or 0 μM guazatine. Values are the mean of at least five biological replicates ±SD. Letters indicate values that are significantly different according to Student-Newman-Keuls test at P < 0.05.
Polyamine Levels in Response to Guazatine Treatment
Free putrescine (Put), spermidine (Spd) and spermine (Spm) levels were quantified in clh1-2, clh1-3, clh2-2, clh2-3, double clh1-2 clh2-3 and wild-type seedlings treated or not with 5 μM guazatine during 16 days. Free Put levels accumulated up to 6.7-fold in guazatine-treated seedlings compared to untreated controls (Figure 5). No evident differences in Put levels were apparent between clh1-2, clh1-3, clh2-2, clh2-3 or double clh1-2 clh2-3 mutants and the wild-type (Figure 5). The levels of free Spd did not change in response to guazatine treatment, whereas those of free Spm were slightly reduced in all genotypes tested (Figure 5). We concluded that guazatine leads to accumulation of free Put and slight reduction of Spm, and this response was similar in clh1, clh2 and wild-type plants. Therefore, CLH1 and CLH2 mutations do not affect PA responsiveness to guazatine.
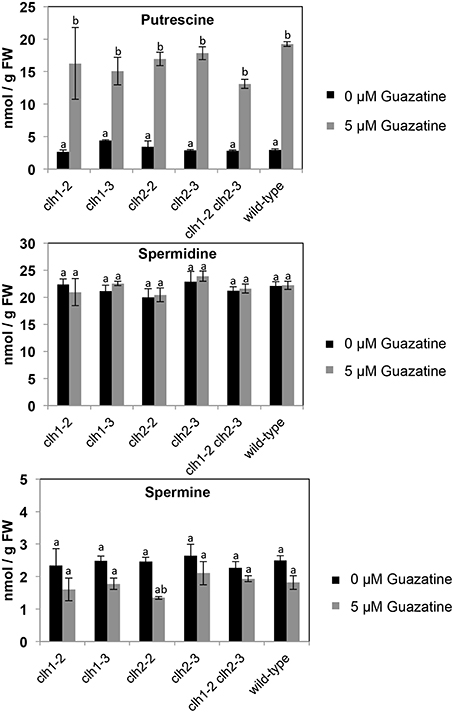
Figure 5. Levels of free puterscine, spermidine, and spermine in clh1, clh2 and double clh1 clh2 mutants grown in the presence of 5 or 0 μM guazatine. Values are the mean of at least five biological replicates ± SD. Letters indicate values that are significantly different according to Student-Newman-Keuls test at P < 0.05.
Natural Allelic Variation at clh1 and clh2
The sequence of CLH1 and CLH2 genes from 53 accessions used in this study (Table S1) was obtained from the 1001 genomes project (www.1001genomes.org) and used to construct CLH1 and CLH2 phylogenies (Figure 6). CLH1 alleles from guazatine tolerant accessions were found in different branches of the CLH1 tree. However, we observed that 10 out of the 22 guazatine tolerant accessions analyzed in this phylogeny, clustered together in the same branch of the tree (clade III), which indicated that they carry similar CLH1 alleles. Guazatine tolerant accessions in this clade belonged to populations from Germany (Kl-5, Mnz-0, Do-0, and Ga-0), Austria (Gr-1 and Gr-5), Italy (Sei-0), Czech Republic (Da1-12) and Sweden (Lom1-1). Most guazatine tolerant accessions in this CLH1 clade did not cluster together in the CLH2 phylogeny, for which variation was higher (Figure 6). Hence, the CLH1 clade III was not likely due to simple genetic relationship between accessions, except for Gr-1 and Gr-5. Because of the contribution of both CLH1 and CLH2 genes to guazatine tolerance, and the high diversity of CLH2 alleles detected that does not correlate with CLH1 phylogeny, we could not identify straightforward associations between specific CLH1 polymorphism(s) and guazatine tolerance traits by simple comparison between tolerant and sensitive variants.
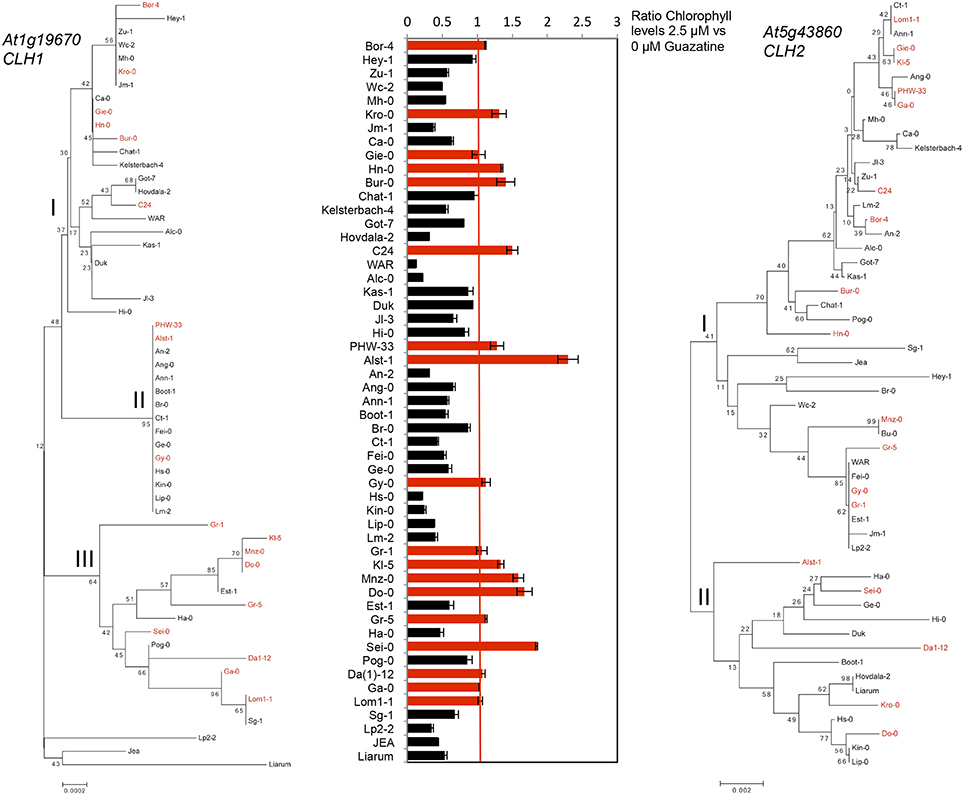
Figure 6. Neighbor-Joining (NJ) tree of At1g19670 (CLH1) and (At5g43860) CLH2 genes. Bootstrap values for different nodes are indicated (% from 1000 replicates). Alleles from guazatine-tolerant accessions are highlighted in red.
CLH1 and CLH2 expression was studied in 11 natural accessions that showed contrasted guazatine-tolerance traits (Figure 7). This analysis evidenced the absence of variation in CLH1 and CLH2 transcript levels between the accessions. Therefore, changes in CLH1 and CLH2 expression are unlikely to underlie guazatine tolerance in Arabidopsis natural populations. Rather, we suggest that non-synonymous substitutions in the coding sequence of CLH1 and CLH2 may cause the quantitative variation observed (Figure 7).
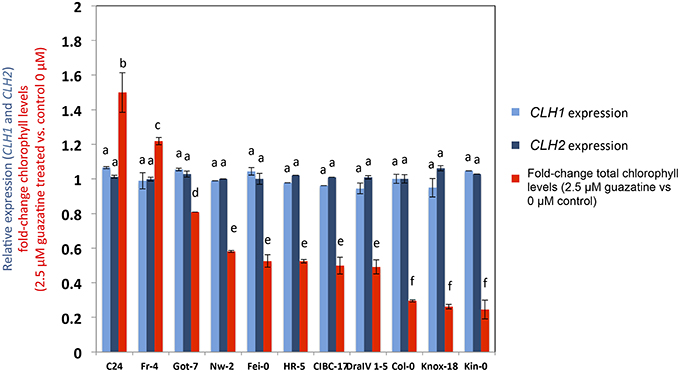
Figure 7. Gene expression analyses of CLH1 and CLH2 and guazatine tolerance. Expression values in different accessions were obtained from Genevestigator (Hruz et al., 2008). Values were obtained from biological triplicates, normalized to UBIQUITIN 10 as housekeeping gene, and expressed relative to Col-0 ± SD. Guazatine tolerance was measured by calculating the fold-change of total chlorophyll levels in 2.5 μM guazatine-treated seedlings vs. untreated control (see Materials and Methods). Letters indicate values that are significantly different according to Student-Newman-Keuls test at P < 0.05.
Overall, we report that genetic variation at both CLH1 and CLH2 genes conditions guazatine tolerance in Arabidopsis. The occurrence of multiple (rare) CLH2 alleles contributing to guazatine tolerance may limit the identification of associations between CLH2 and guazatine tolerance by GWAS, which was validated by mutant analysis.
Discussion
In this work, we report the deleterious effects derived from the exposure to low (2.5 μM) concentrations of guazatine in Arabidopsis seedlings, the occurrence of extensive natural variation for guazatine tolerance traits in a set of 107 accessions, and the identification of genes involved in this response by GWA mapping. Guazatine is used in agriculture as fungicide recommended for cereals and citrus fruits. We have observed that treatment of the weed Arabidopsis with micromolar concentrations of guazatine inhibits growth, primary root elongation and depletes chlorophyll levels (Figures 1, 4). Due to these effects, we conclude that guazatine may be used as herbicide. The 107 accessions selected were sufficient to perform GWA mapping for guazatine tolerance traits and identify CLH1 as candidate gene (Figure 3C), that together with CLH2, were further validated using loss-of-function mutants.
The identification of CLH genes underlying guazatine-tolerance traits indicates the involvement of chlorophyll degradation pathways in this response. Arabidopsis carries two CLH coding genes (CLH1 and CLH2; Benedetti, 1998; Tsuchiya et al., 1999; Benedetti and Arruda, 2002) but associations with guazatine tolerance were only detected for CLH1 (Figure 3). Because CLH2 exhibits higher allelic diversity (Figure 6), we reason that the occurrence of multiple, low frequent CLH2 alleles contributing to guazatine tolerance, might affect the identification of this locus by GWAS. Furthermore, predominant activity of CLH1 over CLH2 in Arabidopsis has been reported (Schenk et al., 2007). Interestingly, no variation in CLH1 and CLH2 expression was evidenced in Arabidopsis accessions differing in their tolerance to guazatine (Figure 7). We suggest that SNPs leading to non-synonymous substitutions in the coding sequence of CLH1 and CLH2 may underlie the naturally occurring variation observed, which is compatible with GWAS analysis.
CLH and pheophytinase (PPH) activities catalyze the cleavage of the lipophilic phytol chain of chlorophyll to produce chlorophyllide, a more hydrophilic derivative (Hörtensteiner, 2006). However, the biological assessment of CLH function in Arabidopsis indicated that CLH1 and CLH2 are not involved in senescence-related chlorophyll breakdown (Schenk et al., 2007; Hu et al., 2015). In Arabidopsis, PPH is localized in chloroplasts (Schelbert et al., 2009) whereas CLH1 and CLH2 are not plastidial proteins (Schenk et al., 2007). CLH1 is located in the ER and tonoplast of plant cells (Hu et al., 2015). Because of the CLH1 localization, chlorophyll could only be substrate of CLH activity upon release of chlorophyll from the thylakoid membranes. This may be caused by different types of abiotic and biotic stresses that damage plant tissues (Karpinski et al., 2003), or the use of guazatine (Figure 1C). In high amounts, some tetrapyrroles can generate ROS and induce cell death (Kruse et al., 1995; Meskauskiene et al., 2001; Hörtensteiner, 2006; Hirashima et al., 2009). Hu et al. (2015) suggested that CLH and chlorophyll constitute a binary defense system effective against certain chewing herbivores, due to the inducible production of chlorophyllide upon attack, which is toxic for Spodoptera litura larvae. Similarly, accumulation of chlorophyllide is a defense mechanism against infection by the necrotrophic fungus Alternaria brassicicola in Arabidopsis (Kariola et al., 2005). Yagura et al. (1984) reported that the fungal activity of guazatine is due to alterations in membrane integrity, permeability and composition. In Arabidopsis, the physiological effects of guazatine application have been less studied. Its use as PAO inhibitor does not require long-term exposure and, for PAO inhibition, guazatine is frequently added to protein extracts for in vitro enzymatic reactions. In this work, we report that long-term exposure to guazatine induces membrane damage in Arabidopsis, which was evidenced in the alteration of chloroplast integrity followed by chlorophyll degradation. Accumulation of osmophilic bodies, which resembled plastoglobules, was evidenced in guazatine-treated leaves (Figure 1C). Such particles accumulate in response to different stresses and senescence, in parallel to the break-down of thylakoid integrity (Austin et al., 2006). Interestingly, guazatine toxicity is not evident in monocots like oat (Capell et al., 1993). For auxinic herbicides, selectivity between dicots and monocots is due to differences in auxin translocation, degradation, perception, and vascular physiology (Gauvrit and Gaillardon, 1991; Monaco et al., 2002; Kelley and Reichers, 2007). Similar mechanisms may underlie guazatine selectivity between dicots and monocots. However, within-species variation in Arabidopsis can be explained by genetic determinisms involving natural variation at CLH1 and CLH2 genes.
CLH1 and/or CLH2 loss-of-function or expression down-regulation attenuate guazatine toxicity in Arabidopsis. We suggest that loss of CLH activity limits chlorophyll degradation under stress conditions that damage the integrity of chloroplast membranes. This would prevent ROS generation and cell death induced by CLH enzymatic activity. Interestingly, no significant differences were observed between the PA profiles of wild-type, clh1 and clh2 mutants treated with guazatine. These observations suggest that guazatine effects under long-term exposure of Arabidopsis seedlings is not due to its activity as PAO inhibitor, but to other mechanisms involving oxidative stress and/or membrane damage.
PAs in the chloroplast are found as free or conjugated forms, the latter forms produced by transglutaminase activities that bind polyamines to stromal and thylakoid proteins (Kotzabasis et al., 1993; Del Duca et al., 1994; Della Mea et al., 2004; Ioannidis et al., 2009; Hamdani et al., 2011). PAs in the photosynthetic apparatus are beneficial and protect against photoinhibition and ROS production (Navakoudis et al., 2003; Demetriou et al., 2007; Hamdani et al., 2011; Yaakoubi et al., 2014). Surprisingly, guazatine application in osmotically stressed oat leaves resulted beneficial and enhanced Spd and Spm levels, which led to the prevention of chlorophyll loss and senescence (Capell et al., 1993). This contrasts with the effects observed in Arabidopsis, in which Put accumulated but Spd or Spm increases were absent (Figure 5). Guazatine application to Vitis vinifera also induced Put accumulation with no concomitant changes in the levels of Spd or Spm (Agudelo-Romero et al., 2014). In this case, the raise in Put levels was likely due to activation of ABA pathway and increased expression of Arginine Decaborxylase (ADC), encoding the first biosynthetic step in the ADC pathway to Put biosynthesis (Agudelo-Romero et al., 2014). We conclude that PA profiles by guazatine treatment vary between species, which may be related to the predominance of terminal catabolism and/or PA back-conversion pathways between species.
Overall, we report natural mechanisms by which Arabidopsis populations can overcome toxicity by polyaminoguanidine-based fungicides used in agriculture, which might be the result of local adaptation processes.
Author Contributions
KA performed all the experimental research. LB performed the GWAS analyses. KA, AT and RA planned the experiments. RA analyzed the data and wrote the paper with contributions from all authors.
Conflict of Interest Statement
The authors declare that the research was conducted in the absence of any commercial or financial relationships that could be construed as a potential conflict of interest.
The reviewer, MC and handling Editor declared their shared affiliation, and the handling Editor states that the process nevertheless met the standards of a fair and objective review.
Acknowledgments
RA acknowledges funding support from the Ramón y Cajal Program (RYC-2011-07847) of the Ministerio de Ciencia e Innovación (Spain), the BFU2013-41337-P grant of the Programa Estatal de Fomento de la Investigación Científica y Técnica de Excelencia (Ministerio de Economía y Competitividad, Spain) co-financed by FEDER (Fondo Europeo de Desarrollo Regional), and the Marie Curie Career Integration Grant (DISEASENVIRON, PCIG10-GA-2011-303568) of the European Union. A.F.T. acknowledges funding support the Spanish Ministerio de Ciencia e Innovación (BIO2011-29683). RA and AT are members of the Grup de Recerca Consolidat 2014 SGR-920 of the Generalitat de Catalunya, Spain. We thank Prof. Maarten Koornneef for Arabidopsis accessions, support, and scientific inspiration on the occasion of his retirement.
Supplementary Material
The Supplementary Material for this article can be found online at: http://journal.frontiersin.org/article/10.3389/fpls.2016.00401
Figure S1. Quantile-Quantile (Q–Q) plots for GWAS analysis of chlorophyll levels in response to guazatine using AMM and LM methods.
Figure S2. Genome wide association mapping profile for chlorophyll levels under control conditions (0 μM guazatine) in 107 Arabidopsis accessions analyzed with the AMM and LM methods.
Figure S3. Detailed view of the genome wide association mapping profile for guazatine tolerance in 107 Arabidopsis accessions analyzed with the AMM and LM methods in the CLH1 (At1g19670) and CLH2 (At5g43860) loci.
Figure S4. Schematic representation of 5′ and 3′ UTRs (white), exons (black), introns (lines), and promoter region (gray) in CLH1 (At1g19670) and CLH2 (At5g43860) genes. The position of T-DNA insertion in clh1-2, clh1-3, clh2-2, and clh2-3 is indicated. The expression of CLH1 and CLH2 in 7-days-old clh1 and clh2 seedlings, respectively, was determined by RT-PCR using gene-specific primers and ACTIN2 as housekeeping control.
Figure S5. Root phenotype of clh1-2, clh1-3, clh2-2, clh2-3 and double clh1-2 clh2-3 16-days-old seedlings. Seedlings were germinated and grown in the absence of guazatine during 4 days, and then transferred to vertical plates containing 1.5 μM guazatine. Pictures were taken 12 days after treatment.
Table S1. List of Arabidopsis thaliana accessions used in this work.
References
Agudelo-Romero, P., Ali, K., Choi, Y. H., Sousa, L., Verpoorte, R., Tiburcio, A. F., et al. (2014). Perturbation of polyamine catabolism affects grape ripening of Vitis vinifera cv. Trincadeira. Plant Physiol. Biochem. 74, 141–155. doi: 10.1016/j.plaphy.2013.11.002
Alonso-Blanco, C., Aarts, M. G. M., Bentsink, L., Keurentjes, J. J. B., Reymond, M., Vreugdenhil, D., et al. (2009). What has natural variation taught us about plant development, physiology, and adaptation? Plant Cell 21, 1877–1896. doi: 10.1105/tpc.109.068114
Atwell, S., Huang, Y. S., Vilhjálmsson, B. J., Willems, G., Horton, M., Li, Y., et al. (2010). Genome-wide association study of 107 phenotypes in Arabidopsis thaliana inbred lines. Nature 465, 627–631. doi: 10.1038/nature08800
Austin, J. R. I. I., Frost, E., Vidi, P.-A., Kessler, F., and Staehlin, L. A. (2006). Plastoglobules are lipoprotein subcompartments of the chloroplast that are permanently coupled to thylakoid membranes and contain biosynthetic enzymes. Plant Cell 18, 1693–1703. doi: 10.1105/tpc.105.039859
Baxter, I., Brazelton, J. N., Yu, D., Huang, Y. S., Lahner, B., Yakubova, E., et al. (2010). A coastal cline in sodium accumulation in Arabidopsis thaliana is driven by natural variation of the sodium transporter AtHKT1;1. PLoS Genet. 6:e1001193. doi: 10.1371/journal.pgen.1001193
Benedetti, C. E. (1998). Differential expression of a novel gene in response to coronatine, methyl jasmonate, and wounding in the coi1 mutant of Arabidopsis. Plant Physiol. 116, 1037–1042. doi: 10.1104/pp.116.3.1037
Benedetti, C. E., and Arruda, P. (2002). Altering the expression of the chlorophyllase gene ATHCOR1 in transgenic Arabidopsis caused changes in the chlorophyll-to-chlorophyllide ratio. Plant Physiol. 128, 1255–1263. doi: 10.1104/pp.010813
Capell, T., Campos, J. L., and Tiburcio, A. F. (1993). Antisenescence properties of guazatine in osmotically stressed oat leaves. Phytochemistry 32, 785–788. doi: 10.1016/0031-9422(93)85205-6
Chan, E. K. F., Rowe, H. C., Corwin, J. A., Joseph, B., and Kliebenstein, D. J. (2011). Combining genome-wide association mapping and transcriptional networks to identify novel genes controlling glucosinolates in Arabidopsis thaliana. PLoS Biol. 9:e1001125. doi: 10.1371/journal.pbio.1001125
Chao, D.-Y., Silva, A., Baxter, I., Huang, Y. S., Nordborg, M., Danku, J., et al. (2012). Genome-wide association studies identify heavy metal ATPase3 as the primary determinant of natural variation in leaf cadmium in Arabidopsis thaliana. PLoS Genet. 8:e1002923. doi: 10.1371/journal.pgen.1002923
Cona, A., Manetti, F., Leone, R., Corelli, F., Tavladoraki, P., Polticelli, F., et al. (2004). Molecular basis for the binding of competitive inhibitors of maize polyamine oxidase. Biochemistry 43, 3426–3435. doi: 10.1021/bi036152z
Del Duca, S., Tidu, V., Bassi, R., Esposito, C., and Serafmi-Fracassini, D. (1994). Identification of chlorophyll-a/b proteins as substrates of transglutaminase activity in isolated chloroplasts of Helianthus tuberosus L. Planta 193, 283–289. doi: 10.1007/BF00192542
Della Mea, M., Di Sandro, A., Dondini, L., Del Duca, S., Vantini, F., Bergamini, C., et al. (2004). A Zea mays 39-kDa thylakoid transglutaminase catalyses the modification by polyamines of light-harvesting complex II in a light-dependent way. Planta 219, 754–764. doi: 10.1007/s00425-004-1278-6
Demetriou, G., Neonaki, C., Navakoudis, E., and Kotzabasis, K. (2007). Salt stress impact on the molecular structure and function of the photosynthetic apparatus–the protective role of polyamines. Biochim. Biophys. Acta 1767, 272–280. doi: 10.1016/j.bbabio.2007.02.020
Dreassi, E., Zizzari, A. T., D'Arezzo, S., Visca, P., and Botta, M. (2007). Analysis of guazatine mixture by LC and LC-MS and antimycotic activity determination of principal components. J. Pharm. Biomed. Anal. 43, 1499–1506. doi: 10.1016/j.jpba.2006.10.029
Federico, R., Leone, L., Botta, M., Binda, C., Angelini, R., Venturini, G., et al. (2001). Inhibition of pig liver and Zea mays L. polyamine oxidase: a comparative study. J. Enzyme Inhib. 16, 147–155. doi: 10.1080/14756360109162364
Filiault, D. L., and Maloof, J. N. (2012). A genome-wide association study identifies variants underlying the Arabidopsis thaliana shade avoidance response. PLoS Genet. 8:e1002589. doi: 10.1371/journal.pgen.1002589
Fincato, P., Moschou, P. N., Spedaletti, V., Tavazza, R., Angelini, R., Federico, R., et al. (2011). Functional diversity inside the Arabidopsis polyamine oxidase gene family. J. Exp. Bot. 62, 1155–1168. doi: 10.1093/jxb/erq341
François, O., Blum, M. G. B., Jakobsson, M., and Rosenberg, N. A. (2008). Demographic history of european populations of Arabidopsis thaliana. PLoS Genet. 4:e1000075. doi: 10.1371/journal.pgen.1000075
Gauvrit, C., and Gaillardon, P. (1991). Effect of low temperatures on 2,4-D behaviour in maize plants. Weed Res. 31, 135–142. doi: 10.1111/j.1365-3180.1991.tb01752.x
Hamdani, S., Gauthier, A., Msilini, N., and Carpentier, R. (2011). Positive charges of polyamines protect PSII in isolated thylakoid membranes during photoinhibitory conditions. Plant Cell Physiol. 52, 866–873. doi: 10.1093/pcp/pcr040
Hirashima, M., Tanaka, R., and Tanaka, A. (2009). Light-independent cell death induced by accumulation of pheophorbide a in Arabidopsis thaliana. Plant Cell Physiol. 50, 719–729. doi: 10.1093/pcp/pcp035
Hörtensteiner, S. (2006). Chlorophyll degradation during senescence. Annu. Rev. Plant Biol. 57, 55–77. doi: 10.1146/annurev.arplant.57.032905.105212
Hruz, T., Laule, O., Szabo, G., Wessendorp, F., Bleuler, S., Oertle, L., et al. (2008). Genevestigator V3: a reference expression database for the meta-analysis of transcriptomes. Adv. Bioinformatics 2008:420747. doi: 10.1155/2008/420747
Hu, X., Makita, S., Schelbert, S., Sano, S., Ochiai, M., Tsuchiya, T., et al. (2015). Reexamination of chlorophyllase function implies its involvement in defense against chewing herbivores. Plant Physiol. 167, 660–670. doi: 10.1104/pp.114.252023
Ioannidis, N. E., Ortigosa, S. M., Veramendi, J., Pintó-Marijuan, M., Fleck, I., Carvajal, P., et al. (2009). Remodeling of tobacco thylakoids by over-expression of maize plastidial transglutaminase. Biochim. Biophys. Acta 1787, 1215–1222. doi: 10.1016/j.bbabio.2009.05.014
Kariola, T., Brader, G., Li, J., and Palva, E. T. (2005). Chlorophyllase 1, a damage control enzyme, affects the balance between defense pathways in plants. Plant Cell 17, 282–294. doi: 10.1105/tpc.104.025817
Karpinski, S., Gabrys, H., Mateo, A., Karpinska, B., and Mullineaux, P. M. (2003). Light perception in plant disease defence signalling. Curr. Opin. Plant Biol. 6, 390–396. doi: 10.1016/S1369-5266(03)00061-X
Kelley, K. B., and Reichers, D. E. (2007). Recent developments in auxin biology and new opportunities for auxinic herbicide research. Pesticide Biochem. Physiol. 89, 1–11. doi: 10.1016/j.pestbp.2007.04.002
Koornneef, M., Alonso-Blanco, C., and Vreugdenhil, D. (2004). Naturally occurring genetic variation in Arabidopsis thaliana. Annu. Rev. Plant Biol. 55, 141–172. doi: 10.1146/annurev.arplant.55.031903.141605
Korte, A., and Farlow, A. (2013). The advantages and limitations of trait analysis with GWAS: a review. Plant Methods 9:29. doi: 10.1186/1746-4811-9-29
Kotzabasis, K., Fotinou, C., Roubelakis-Angelakis, K. A., and Ghanotakis, D. (1993). Polyamines in the photosynthetic apparatus: photosystem II highly resolved subcomplexes are enriched in spermine. Photosyn. Res. 38, 83–88. doi: 10.1007/BF00015064
Kruse, E., Mock, H. P., and Grimm, B. (1995). Coproporphyrinogen III oxidase from barley and tobacco–sequence analysis and initial expression studies. Planta 196, 796–803. doi: 10.1007/BF01106776
Li, Y., Huang, Y., Bergelson, J., Nordborg, M., and Borevitz, J. O. (2010). Association mapping of local climate-sensitive quantitative trait loci in Arabidopsis thaliana. Proc. Natl. Acad. Sci. U.S.A. 107, 21199–21204. doi: 10.1073/pnas.1007431107
Lobet, G., Pagès, L., and Draye, X. (2011). A novel image-analysis toolbox enabling quantitative analysis of root system architecture. Plant Physiol. 157, 29–39. doi: 10.1104/pp.111.179895
Marcé, M., Brown, D. S., Capell, T., Figueras, X., and Tiburcio, A. F. (1995). Rapid high-performance liquid chromatographic method for the quantitation of polyamines as their dansyl derivatives: application to plant and animal tissues. J. Chromatogr. B Biomed. Appl. 666, 329–335. doi: 10.1016/0378-4347(94)00586-T
Marina, M., Maiale, S. J., Rossi, F. R., Romero, M. F., Rivas, E. I., Gárriz, A., et al. (2008). Apoplastic polyamine oxidation plays different roles in local responses of tobacco to infection by the necrotrophic fungus Sclerotinia sclerotiorum and the biotrophic bacterium Pseudomonas viridiflava. Plant Physiol. 147, 2164–2178. doi: 10.1104/pp.108.122614
Meskauskiene, R., Nater, M., Goslings, D., Kessler, F., op den Camp, R., and Apel, K. (2001). FLU: a negative regulator of chlorophyll biosynthesis in Arabidopsis thaliana. Proc. Natl. Acad. Sci. U.S.A. 98, 12826–12831. doi: 10.1073/pnas.221252798
Monaco, T. J., Weller, S. C., and Ashton, F. M. (2002). Weed Science: Principles and Practices. New York, NY: Wiley-Blackwell.
Moschou, P. N., Sanmartin, M., Andriopoulou, A. H., Rojo, E., Sanchez-Serrano, J. J., and Roubelakis-Angelakis, K. A. (2008). Bridging the gap between plant and mammalian polyamine catabolism: a novel peroxisomal polyamine oxidase responsible for a full back-conversion pathway in Arabidopsis. Plant Physiol. 147, 1845–1857. doi: 10.1104/pp.108.123802
Navakoudis, E., Lütz, C., Langebartels, C., Lütz-Meindl, U., and Kotzabasis, K. (2003). Ozone impact on the photosynthetic apparatus and the protective role of polyamines. Biochim. Biophys. Acta 1621, 160–169. doi: 10.1016/S0304-4165(03)00056-4
Porra, R. J. (2002). The chequered history of the development and use of simultaneous equations for the accurate determination of chlorophylls a and b. Photosyn. Res. 73, 149–156. doi: 10.1023/A:1020470224740
Schelbert, S., Aubry, S., Burla, B., Agne, B., Kessler, F., Krupinska, K., et al. (2009). Pheophytin pheophorbide hydrolase (pheophytinase) is involved in chlorophyll breakdown during leaf senescence in Arabidopsis. Plant Cell 21, 767–785. doi: 10.1105/tpc.108.064089
Schenk, N., Schelbert, S., Kanwischer, M., Goldschmidt, E. E., Dörmann, P., and Hörtensteiner, S. (2007). The chlorophyllases AtCLH1 and AtCLH2 are not essential for senescence-related chlorophyll breakdown in Arabidopsis thaliana. FEBS Lett. 581, 5517–5525. doi: 10.1016/j.febslet.2007.10.060
Seren, Ü., Vilhjálmsson, B. J., Horton, M. W., Meng, D., Forai, P., Huang, Y. S., et al. (2012). GWAPP: a Web application for genome-wide association mapping in Arabidopsis. Plant Cell 24, 4793–4805. doi: 10.1105/tpc.112.108068
Tsuchiya, T., Ohta, H., Okawa, K., Iwamatsu, A., Shimada, H., Masuda, T., et al. (1999). Cloning of chlorophyllase, the key enzyme in chlorophyll degradation: finding of a lipase motif and the induction by methyl jasmonate. Proc. Natl. Acad. Sci. U.S.A. 96, 15362–15367. doi: 10.1073/pnas.96.26.15362
Wild, B. L. (1983). Double resistance by citrus green mould Penicillium digitatum to the fungicides guazatine and benomyl. Ann. Appl. Biol. 103, 237–241. doi: 10.1111/j.1744-7348.1983.tb02760.x
Yaakoubi, H., Hamdani, S., Bekalé, L., and Carpentier, R. (2014). Protective action of spermine and spermidine against photoinhibition of photosystem I in isolated thylakoid membranes. PLoS ONE 9:e112893. doi: 10.1371/journal.pone.0112893
Yagura, Y., Kirinuki, T., and Matsunaya, S. (1984). Mode of action of the fungicide guazatine in Alternaria kikuchiana. J. Pestic. Sci. 9, 425–431. doi: 10.1584/jpestics.9.425
Keywords: guazatine, polyaminoguanidines, GWAS, natural variation, population genetics
Citation: Atanasov KE, Barboza-Barquero L, Tiburcio AF and Alcázar R (2016) Genome Wide Association Mapping for the Tolerance to the Polyamine Oxidase Inhibitor Guazatine in Arabidopsis thaliana. Front. Plant Sci. 7:401. doi: 10.3389/fpls.2016.00401
Received: 11 December 2015; Accepted: 14 March 2016;
Published: 05 April 2016.
Edited by:
Riccardo Angelini, Roma Tre University, ItalyReviewed by:
Günther F. E. Scherer, Leibniz Universität Hannover, GermanyAutar Krishen Mattoo, United States Department of Agriculture, USA
Manuela Cervelli, Roma Tre University, Italy
Copyright © 2016 Atanasov, Barboza-Barquero, Tiburcio and Alcázar. This is an open-access article distributed under the terms of the Creative Commons Attribution License (CC BY). The use, distribution or reproduction in other forums is permitted, provided the original author(s) or licensor are credited and that the original publication in this journal is cited, in accordance with accepted academic practice. No use, distribution or reproduction is permitted which does not comply with these terms.
*Correspondence: Rubén Alcázar, cmFsY2F6YXJAdWIuZWR1