- 1Botany and Plant Science, School of Natural Sciences, Ryan Institute, National University of Ireland Galway, Galway, Ireland
- 2Research Group Germline Biology, Centre for Organismal Studies (COS), Heidelberg University, Heidelberg, Germany
- 3Plant Abiotic Stress Research Group & Faculty of Applied Sciences, Ton Duc Thang University, Ho Chi Minh City, Vietnam
- 4Signaling Pathway Research Unit, RIKEN Center for Sustainable Resource Science, Tsurumi, Japan
As we march into the 21st century, the prevailing scenario of depleting energy resources, global warming and ever increasing issues of human health and food security will quadruple. In this context, genetic and metabolic engineering of green microalgae complete the quest toward a continuum of environmentally clean fuel and food production. Evolutionarily related, but unlike land plants, microalgae need nominal land or water, and are best described as unicellular autotrophs using light energy to fix atmospheric carbon dioxide (CO2) into algal biomass, mitigating fossil CO2 pollution in the process. Remarkably, a feature innate to most microalgae is synthesis and accumulation of lipids (60–65% of dry weight), carbohydrates and secondary metabolites like pigments and vitamins, especially when grown under abiotic stress conditions. Particularly fruitful, such an application of abiotic stress factors such as nitrogen starvation, salinity, heat shock, etc., can be used in a biorefinery concept for production of multiple valuable products. The focus of this mini-review underlies metabolic reorientation practices and tolerance mechanisms as applied to green microalgae under specific stress stimuli for a sustainable pollution-free future. Moreover, we entail current progress on genetic engineering as a promising tool to grasp adaptive processes for improving strains with potential biotechnological interests.
Introduction
Due to their taxonomic and biochemical diversity, microalgae symbolize an unconventional source of molecules (Stengel et al., 2011). For example, the inherent nature of microalgae to accumulate lipids, carbohydrates, and secondary metabolites under abiotic stress can be applied in a biorefinery concept for generation of value-added compounds (Markou and Nerantzis, 2013). Nonetheless, transgenic microalgae impact diverse businesses such as energy, human and animal nutraceuticals, pharmaceuticals, health, beauty, and exquisite chemicals (Rasala et al., 2014). As naturalized for bacteria, yeasts, and plants, genetic engineering, therefore, constitutes promising strategy for studying abiotic stress responses in microalgae through novel phenotypes and specific traits. Conversely, microalgae as model photosynthetic organisms represent an ideal experimental system to study major plant processes, as mimicking algal strategies to sense, respond and cope with abiotic stress can radically improve growth and metabolite productivity in plants. Over the years, significant advances in genetic manipulation of green microalgae have been achieved (Mayfield and Golden, 2015), as exemplified through production of omega-3 fatty acids, carotenoids, biofuels, as well as improved photosynthetic growth (Gimpel et al., 2015). Despite tremendous advances in sequenced genome, in particular for chlorophytes and heterokonts (i.e., diatoms), major obstacles feature need of additional well-established transformation vectors, potential inability to engineer and specifically localize transgenic proteins to sub-cellular locations and robust expression of multiple nuclear-encoded transgenes (Gong et al., 2011; Scranton et al., 2015). In this minireview, we discuss recent examples highlighting futuristic use of genetic engineering to design strains with potential biotechnological interests (Figure 1). Moreover, we surmise major abiotic stress resilience strategies envisaged in unicellular eukaryotic green microalgae.
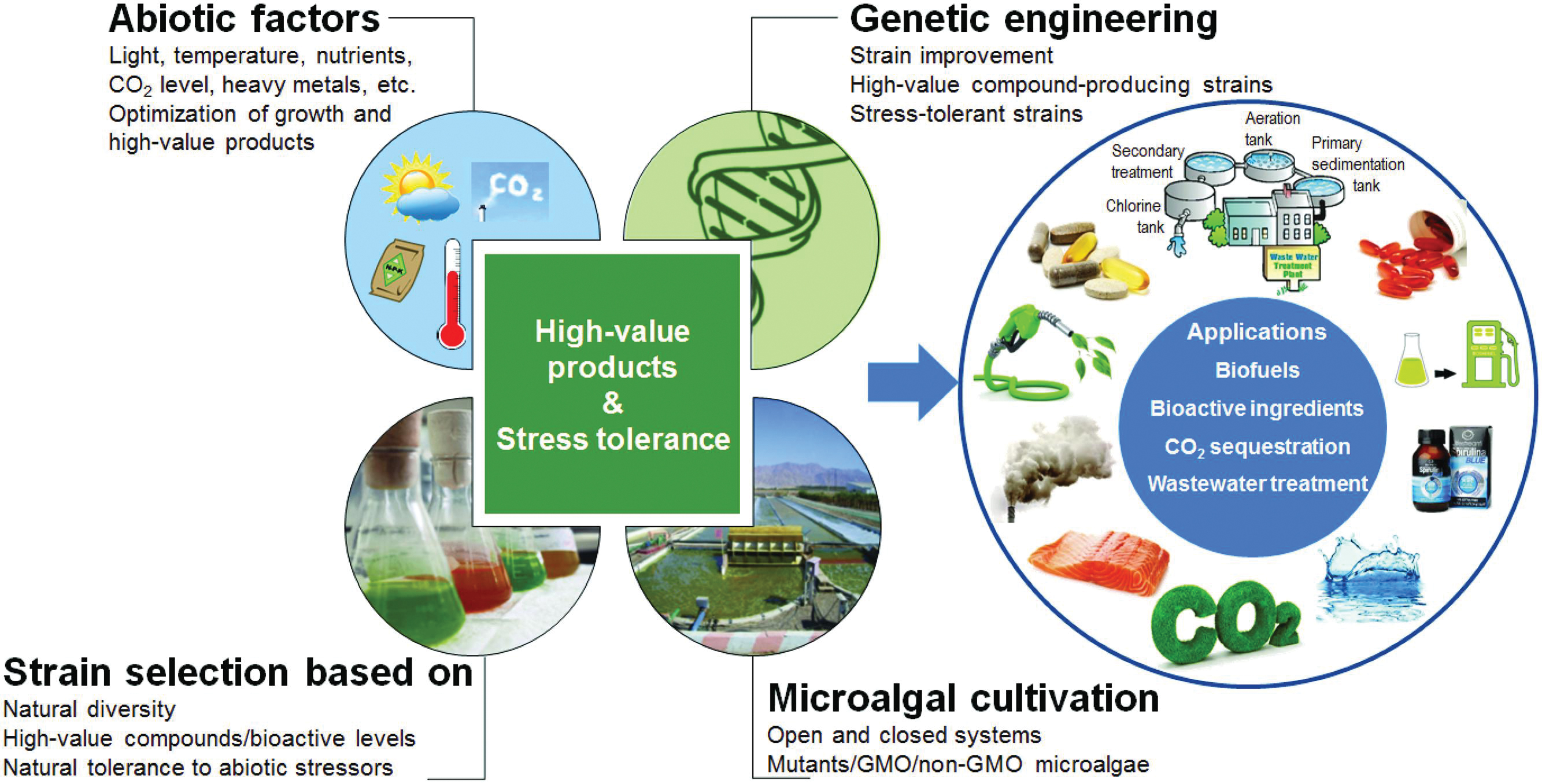
FIGURE 1. Illustrated scheme for improving microalgal strains for potential biotechnological interests via genetic engineering. GMO, genetically modified organism.
Current Progress on Genetic and Metabolic Engineering of Green Microalgae
What We Know and What Is Available?
The pursuit of stress-tolerant strains has seen an unprecedented surge of algal transgenesis for recombinant proteins, enhanced photosynthesis, and key metabolite pathways, as well as production of precious nutraceuticals, pharmaceuticals, and biofuels (Gangl et al., 2015). Coupled to this, advanced cultivation of novel species with genome-wide omics (genomics, transcriptomics, lipidomics, and metabolomics) has facilitated successful transformation of microalgae (e.g., Chlamydomonas reinhardtii, Phaeodactylum tricornutum, Thalassiosira pseudonana, Porphyridium sp., Nannochloropsis gaditana, Ostreococcus tauri, Haematococcus pluvialis, etc.). Previously, lack of genome and transcriptome data, as well as efficient tools for algal transformation prevented their genetic manipulation. Consequently, algal transformation systems had to be revisited, developed anew, including methods to introduce transgenic DNA, identification of suitable promoters, distinct selectable markers and expression vectors, as well as needs for codon usage optimization (Qin et al., 2012; Hlavova et al., 2015).
Despite gigantic successes being achieved, only a few algal species still show firm and stable expression of foreign proteins. Increasing evidence suggests intrinsic algal gene silencing mechanisms; albeit repressive histone H3 lysine methylation, DNA cytosine methylation, RNA interference and microRNA-mediated gene regulation for instable foreign transcript or protein degradation (Kim et al., 2015). Likewise, the knowledge of such transgene evading mechanisms will be useful in regulating efficient protein production in metabolically engineered microalgae. Additionally, the emergence of fast and reliable next-generation sequencing techniques have sped up genome sequencing programs and transcriptomic research (Morozova and Marra, 2008). As a result, it is now possible to directly correlate the ensuing transcriptomic, proteomic, and metabolomic profile with respect to pre-determined environmental stimuli, bridging the gap between microalgal genotype and observed phenotype (Dong and Chen, 2013). In future, a combination of transcriptomic, proteomic, and metabolomic studies will be crucial in demarcating gateways to metabolic flux for boosting algal strain-engineering strategies (Jones and Mayfield, 2012). In addition, a battery of bioinformatic softwares like Algal Functional Annotation Tool, GreenCut2, AlgaGEM, Bag2D, PredAlgo (de Oliveira Dal’Molin et al., 2011; Karpowicz et al., 2011; Lopez et al., 2011; Tardif et al., 2012; Yao et al., 2015), and in silico kinetic and metabolic reconstruction models1 are now available for gene/protein characterization, gene metabolic alterations, and predicting proteins subcellular localizations at a whole genome level in various algal species. For example, an in silico metabolic reconstruction map allowed characterization of light-induced metabolic response for >1000 genes in Chlamydomonas (Chang et al., 2011). Likewise, the Chlamydomonas resource center2 harbors a collection of ~300 plasmids and >2700 strains for sustaining research within diverse aspects of algal cell biology. Additionally, a genome-wide Chlamydomonas insertional mutant library3 containing >20,000 mutants covering 10,301 genes (58% of the genome) and cataloged at Chlamydomonas resource center has been recently reported (Li et al., 2016).
Genetic Engineering: Progress and Future Prospects
With an eye toward the future, methods to engineer entire biochemical pathways or multigene traits via homologous/heterologous recombination and development of optimized cloning vectors are underway, as demonstrated by stable integration and subcellular localization of reporter proteins in the chloroplast and nuclear genomes of C. reinhardtii (Noor-Mohammadi et al., 2012; Rasala et al., 2014; Scranton et al., 2016). Such a strategy can be advantageous for foreign gene integration, omitting positional side effects and transcriptional/post-transcriptional gene silencing processes associated with nuclear expression (Kim et al., 2015). In addition, successful yet simple nuclear gene targeting systems have been described in Chlamydomonas (Zorin et al., 2009). Novel permutations of promoter and 5′UTR, like 16S rRNA promoter and atpA 5′UTR have also been illustrated for sufficient heterologous transgene expression (Rasala et al., 2011) and robust expression of transgenic proteins within the C. reinhardtii chloroplast (Tissot-Lecuelle et al., 2014). Examples of metabolic engineering in microalgae comprise codon optimized expression of genes hemH and lba in the chloroplast of C. reinhardtii for optimum bio-hydrogen generation (Wu et al., 2011). Recently, synthetic promotors were generated to drive robust nuclear gene expression in Chlamydomonas (Scranton et al., 2016).
In search of optimal methods for exogenous DNA delivery within rigid algal cell walls, a zinc oxide nanowire array microdevice system was recently elaborated for C. reinhardtii (Bae et al., 2015) with recorded efficiency 6.52 × 104- and 9.66 × 104-fold higher than traditional glass bead beating and electroporation methods. Besides, a biocontainment method for transplastomic microalgae was reported via codon reassignment in the chloroplast of C. reinhardtii (Young and Purton, 2015). Such a codon reassignment strategy can be potentially useful for solving imminent problems in chloroplast engineering of genes whose products are toxic to Escherichia coli cells. Similarly, a self-cloning-based (cloning DNA from a donor into a recipient, between which the natural exchange of DNA is possible) positive selection system for breeding and genetic transformation of Pseudochoricystis ellipsoidea was developed (Kasai et al., 2015). Such recombinant microalgae are considered natural (non-GMO) in Japan and are not governed under the Cartagena domestic law with regards to GMO biosafety (Lusser et al., 2012; USDA Japan Reports, 2014). Such algal molecular breeding techniques may form basis for future large-scale cultivation in outdoor open pond cultivation (Ho et al., 2014).
Finally, reports for high level targeted genome editing in C. reinhardtii via zinc finger nucleases (Sizova et al., 2013), transcription activator-like effectors (TALE; Gao et al., 2014), and lately clustered regularly interspersed short palindromic repeat (CRISPR) system are emerging (Jiang et al., 2014). CRISPR-associated protein 9 (CAS9) is an RNA-guided DNA nuclease successfully practiced for targeted mutations into eukaryotic genomes (Dominguez et al., 2016). Although stable transformants of C. reinhardtii expressing the CAS9 protein could not be recovered, in future, such studies would serve as gold standard for dedicated nuclear, chloroplast, and mitochondria genome-editing strategies.
Microalgal Stress Response Strategies
Abiotic Stress Tolerance in Green Microalgae
Owing to incredible metabolic plasticity of microalgae (Stengel et al., 2011), numerous studies have outlined ramifications of abiotic stress influencing algal biology and metabolism. High light, temperature, salinity, metals, CO2 levels, and nutrient depletion affect growth, photosynthesis, and biochemical composition in a species-specific manner (Perales-Vela et al., 2006; Solovchenko and Khozin-Goldberg, 2013).
One of the most detrimental abiotic stressor damaging algae is high light intensity, when absorption of light energy surpasses the capacity for light utilization in photosynthesis. Several mechanisms termed as non-photochemical quenching (NPQ) exist, to avoid this photodamage (Allorent et al., 2013; Mayank et al., 2015). NPQ consists of three components: qE (energy-dependent quenching), qI (photoinhibitory quenching), and qT (light-state transition; Eberhard et al., 2008; Depauw et al., 2012). The qE increases the thermal dissipation of excessive light energy and represents the major photo-protective process characterizing vascular plants, green microalgae and diatoms (Müller et al., 2001; Roháček et al., 2014). In model green alga C. reinhardtii, NPQ is stimulated by variations in pH in the thylakoid lumen, in response to high light intensity, triggering the xanthophyll cycle (XC) and activating the PSII LHC stress-related (LhcSR) proteins (Finazzi et al., 2006; Maruyama et al., 2014; Wobbe et al., 2016). The latter initiates an onset of NPQ by perceiving lumenal pH environment and in a concentration-dependent manner aggravates fluorescence quenching; the XC plays key role in boosting light harvesting and photoprotection (Goss and Jakob, 2010). Nevertheless, studies of their NPQ and XC activities have suggested species-specific differences in process of excessive energy dissipation between six green microalgae (Quaas et al., 2015). Of interest, microalgal species like Dunalliela spp. and H. pluvialis display the capacity to synthetize and accumulate tremendous amounts of secondary carotenoids (β-carotene and astaxanthin, respectively) under stress (Lamers et al., 2012; Scibilia et al., 2015). In photosynthetic organisms, response to high light involves enormous changes in expression of light-regulated genes and their regulatory components (Lemoine and Schoefs, 2010). Over the years, dedicated gene expression studies with microalgae exposed to different light regimes were undertaken, unveiling peculiar molecular actors associated with light stress responses (e.g., Lhcx gene group in diatoms, known to be closely related to LhcSR genes of C. reinhardtii; Maruyama et al., 2014).
Microalgae further picture a suite of adaptive mechanisms in response to changes in salinity, i.e., passive “osmometer” behavior. Such a profile largely depends on physical and chemical characteristics of the cell wall membrane, regulation of water fluxes, mechanisms to equilibrate intracellular ionic levels using ion-selective channels and carriers, and synthesis or degradation of low-molecular-weight organic solutes (Kirst, 1990). The halotolerant green alga, Dunaliella salina, has been described as a model alga for deducing response of plant cells to salt stress (Cowan et al., 1992). Employing a proteomic approach for salinity stress induced proteins in Dunaliella, Liska et al. (2004) decoded up-regulation of major Calvin cycle enzymes, starch mobilization enzymes and enzymes involved in redox energy generation; factors involved in protein production and degradation; and an analog of bacterial Na++-redox transporters. The results are suggestive of Dunaliella’s response to high salinity by enhancing photosynthetic CO2 consumption and allocating carbon and energy budget for synthesis of glycerol, its osmo-protective component. On the same lines, salt-stress up-regulated the carotenoid ketolase (BKT) in Chlorella zogingiensis and enhanced the accumulation of canthaxanthin and astaxanthin. High salinity also stimulated the generation of reactive oxygen species, which in turn triggered the up-regulation of distinct carotenogenic genes and increased build-up of antioxidant carotenoids (Li et al., 2009).
Changes in temperature are known to strongly affect growth, photosynthetic activity and biochemical composition in microalgae (Geider, 1987; Renaud et al., 2002; Claquin et al., 2008). Indeed, higher temperatures often result in decline in protein production and rise in lipid and carbohydrates levels. An increase in polyunsaturated fatty acids (PUFAs) might be one of the ways microalgae adjust to low-temperature and preserve membrane fluidity (Jiang and Gao, 2004). Interestingly, few microalgae exhibit high temperature tolerance up to 40–42°C (Hanagata et al., 1992; Sakai et al., 1995; de-Bashan et al., 2008). Heat shock proteins (HSPs) are molecular chaperones, crucial during heat shock response and ensuing adaptive homeostases against environmental stresses. 17 small HSPs has been identified from complete genome sequences of five diverse algae: C. reinhardtii, Cyanidioschyzon merolae, Ostreococcus lucimarinus, O. tauri, and T. pseudonana (Waters and Rioflorido, 2007). However, sequence analysis reveals that the diversity and abundance of algal small HSPs does not fully correlate with acclimation to extreme temperatures. Recently, two small HSPs have been shown to be up-regulated and activated at a threshold temperature of ~36°C in C. reinhardtii (Kobayashi et al., 2014). These results stress the advantage of an exclusive temperature-sensing system in the evolution and resilience to elevated temperatures among microalgae.
Mechanisms of CO2 Concentration and Heavy Metal Tolerance
Rise in atmospheric O2 and decrease in oceanic, dissolved inorganic carbon during eco-genesis enabled photoautotrophic organisms to formulate sophisticated CO2-concentrating mechanisms (CCMs). Such mechanisms enhance CO2 uptake by increasing CO2 concentration around ribulose-1,5-bisphosphate carboxylase/oxygenase (Rubisco), an enzyme of the chloroplast stroma that catalyzes the entry of CO2 into the Calvin–Benson–Bassham cycle to maintain sufficient photosynthesis (Raven, 2010). To date, numerous published reports have described low-CO2-acclimation/adaptation within some cyanobacteria and unicellular eukaryotes; but, information regarding high-CO2 tolerance processes remains elusive. One CO2 mitigation strategy consists of selecting and developing strains that stand rigorously high CO2 levels to couple microalgal-mediated CO2 fixation and production of biofuels and other secondary metabolites (Bhola et al., 2014). Since CO2 tolerance varies greatly between species, microalgae have been arbitrarily divided into CO2-sensitive (inhibited by <2–5% CO2) and CO2-tolerant (surviving up to 20% CO2) groups (Miyachi et al., 2003). An example of such CO2-tolerant species includes chlorophytes Chlorella sp. KR-1 and Chlorococcum littorale that demonstrate rapid growth at 40 and 60% CO2, respectively (Iwasaki et al., 1996; Sung et al., 1999). Principal factors responsible for high CO2 tolerance in such extremophile symbiotic species constitute state transitions in photosynthetic apparatus that augment ATP generation, enhanced activity of H+-ATPase for pumping protons out of the cell, hasty shutdown of CCMs, adjustment of fatty acid composition and diversion of excess photosynthates to generation of energy-rich compounds, such as triacylglycerols (Solovchenko and Khozin-Goldberg, 2013).
Microalgae also possess molecular machinery that allows them to distinguish between non-essential and essential heavy metals (Perales-Vela et al., 2006). This ability makes microalgae better suited for treatment of heavy metal polluted water, employing algal-based biotechnologies for waste-water remediation (Suresh Kumar et al., 2015). Indeed, microalgae take up and store toxic metals from the environment (Perales-Vela et al., 2006). For instance, microalgae can preferentially synthesize peptides capable to bind heavy metals. These peptides, as part of organometallic complexes, are additionally partitioned inside vacuoles to aid pertinent control of the cytoplasmic concentration of metal ions, thus nullifying their potential toxic effect (Cobbett and Goldsbrough, 2002). C. reinhardtii has been described as a model photosynthetic eukaryotic for investigating heavy metal tolerance or homeostasis due to molecular and genetic tools available for this species (Hanikenne, 2003). For example, phytochelatins (PC), low-molecular weight peptides, containing sulfur rich cysteine amino acid have been elicited as major intracellular cadmium (Cd) chelators in C. reinhardtii (Hu et al., 2001). In Dunaliella tertiolecta, PC, inducibly synthesized by zinc treatment, can function both in detoxifying heavy metals and alleviation of oxidative stress (Tsuji et al., 2002). Thioredoxins (TRXs) are also known to accord heavy metal detoxification in Chlamydomonas as exemplified by two TRX genes being differentially stimulated by Cd and mercury (Lemaire et al., 1999). As glutathione (GSH)-heavy metal adducts serve as substrates for PC synthase, an earlier study centered on two strains of Scenedesmus acutus (wild type and Cr6+ tolerant) reported the constitutive cysteine concentration higher in the Cr6+-tolerant strain (Torricelli et al., 2004). When the cells were subjected to Cd2+, the tolerant strain had increased levels of reduced GSH and class III metallothioneins (MtIII) as compared to the wild-type strain. Finally, proline (Pro) has also been reported to play a dominant role in alleviating environmental stress including heavy metal stress in plants, microorganisms and microalgae (Siripornadulsil et al., 2002).
Clearly, abiotic stress has the ability to reorient a holistic and sometimes specific microalgal metabolism as an efficient means for increasing the production of selected compounds. Keeping this in mind we highlight in the next section recent advances in microalgal engineering which, when combined with sophisticated physiological and biophysical approaches, may enhance microalgal stress resilience under disparate conditions.
Examples of Stress-Resilience in Microalgae via Genetic Engineering
Despite most engineering strategies favoring increased production of high value compounds (e.g., antioxidant pigments and PUFAs) and biofuel-molecules (e.g., hydrogen and triacylgycerol), recent works have been driven to develop resilient strains with applications like CO2 sequestration and heavy metal biomitigation (Table 1). For example, a moth bean δ1-pyrroline-5-carboxylate synthetase (P5CS) gene has been expressed in C. reinhardtii, with transformants displaying 80% higher free-Pro levels, rapid growth at toxic Cd concentrations, and tremendous binding at four-fold higher Cd concentration compared to wild-type cells (Siripornadulsil et al., 2002). The results propose role of free-Pro as an antioxidant in Cd-stressed cells with resulting higher GSH levels facilitating enhanced phytochelatin synthesis and sequestration of Cd. Expeditious transgenesis has been reported using such microalgae to enhance heavy metal sensitivity and binding specificity for contaminated wastewaters and sediments (Rajamani et al., 2007). Much interest has also been diverted toward engineering small and large subunits of Rubisco (rbcS and rbcL) as targets for increasing net CO2 fixation (photosynthesis) and promoting CO2 sequestration through improvement of growth (Genkov et al., 2010; Whitney et al., 2011). In this regard, Chlamydomonas is a notable host for genetic manipulation, as changes can be made to both rbcS and rbcL genes. For instance, hybrid Rubiscos have been reported, by combining plant (Arabidopsis, spinach and sunflower) small (rbcS) subunits with algal large (rbcL) subunits via transformation of a C. reinhardtii mutant deficient in rbcS gene (Genkov et al., 2010). Although the hybrid enzymes show 3–11% increase in CO2/O2 affinity, they retain optimal V max values and catalytically proficient Rubisco. The hybrid strains, however, lack chloroplast pyrenoids and display reduced photosynthesis. As asserted by Whitney et al. (2011), future research to engineer and test superior Rubiscos will considerably rely on algal model systems, particularly Chlamydomonas.
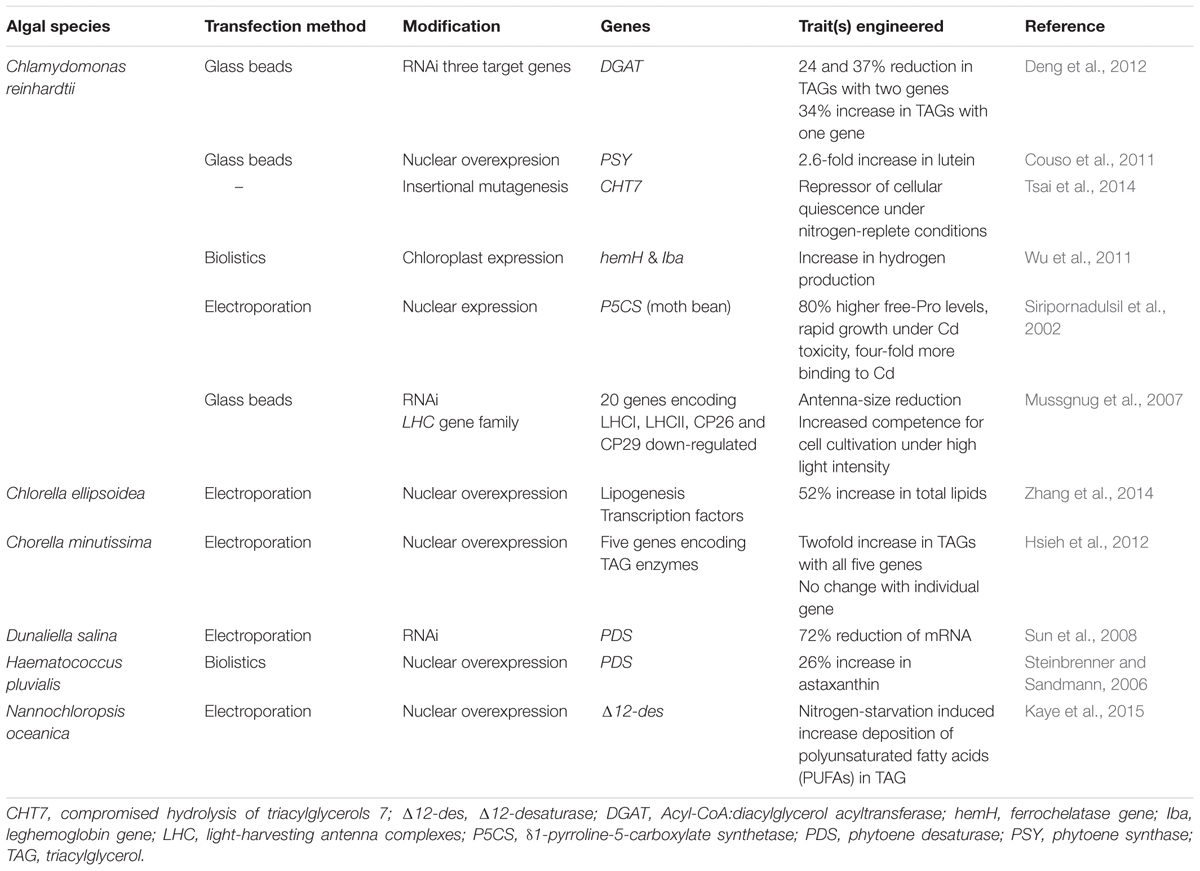
TABLE 1. Examples of green algal species aptly transformed to date together with methods applied and trait(s) enhanced for proficient metabolic or genetic engineering.
Conclusion
Genetic manipulation of microalgae can provide feasible respite by meeting the immediate and long-term demands for food and fuel production on a sustainable basis. A clear step toward the realization of this goal is the development of microalgae-microbial fuel cells (mMFCs) that channelize the solar energy to electrical energy via algal metabolic pathways (Lee et al., 2015). Besides, genetic engineering of microalgae would supplement land plants and industrial biotechnology for value-added products, thereby saving land and air pollution. Already much is achieved through reinforced tools, including rapid sequencing of algal genomes, control of environmental stress conditions, as well as mining of new genes for enhancing lipid and bioactive productions, reducing photo-inhibition, and manipulation of Rubisco- and Calvin-cycle enzymes for metabolic engineering. The field of algal genetic engineering will require renewed attention both at basic and applied levels to envision a prosperous future.
Author Contributions
FG and AK designed and conceptualized the idea and thereafter wrote different section or subsections in consultation with L-ST. All the authors read and finalized the version before submission.
Conflict of Interest Statement
The authors declare that the research was conducted in the absence of any commercial or financial relationships that could be construed as a potential conflict of interest.
Footnotes
References
Allorent, G., Tokutsu, R., Roach, T., Peers, G., Cardol, P., Girard-Bascou, J., et al. (2013). A dual strategy to cope with high light in Chlamydomonas reinhardtii. Plant Cell 25, 545–557. doi: 10.1105/tpc.112.108274
Bae, S., Park, S., Kim, J., Choi, J. S., Kim, K. H., Kwon, D., et al. (2015). Exogenous gene integration for microalgal cell transformation using a nanowire-incorporated microdevice. ACS Appl. Materials Interf. 7, 27554–27561. doi: 10.1021/acsami.5b09964
Bhola, V., Swalaha, F., Ranjith Kumar, R., Singh, M., and Bux, F. (2014). Overview of the potential of microalgae for CO2 sequestration. Int. J. Environ. Sci. Technol. 11, 2103–2118. doi: 10.1007/s13762-013-0487-6
Chang, R. L., Ghamsari, L., Manichaikul, A., Hom, E. F. Y., Balaji, S., Fu, W., et al. (2011). Metabolic network reconstruction of Chlamydomonas offers insight into light-driven algal metabolism. Mol. Syst. Biol. 7:518. doi: 10.1038/msb.2011.52
Claquin, P., Probert, I., Lefebvre, S., and Veron, B. (2008). Effects of temperature on photosynthetic parameters and TEP production in eight species of marine microalgae. Aquatic Microbial Ecology 51, 1–11. doi: 10.3354/ame01187
Cobbett, C., and Goldsbrough, P. (2002). Phytochelatins and metallothioneins: roles in heavy metal detoxification and homeostasis. Annu. Rev. Plant Biol. 53, 159–182. doi: 10.1146/annurev.arplant.53.100301.135154
Couso, I., Vila, M., Rodriguez, H., Vargas, M. A., and León, R. (2011). Overexpression of an exogenous phytoene synthase gene in the unicellular alga Chlamydomonas reinhardtii leads to an increase in the content of carotenoids. Biotechnol. Prog. 27, 54–60. doi: 10.1002/btpr.527
Cowan, A. K., Rose, P. D., and Horne, L. G. (1992). Dunaliella salina: a model system for studying the response of plant cells to stress. J. Exp. Bot. 43, 1535–1547. doi: 10.1093/jxb/43.12.1535
de-Bashan, L. E., Trejo, A., Huss, V. A. R., Hernandez, J.-P., and Bashan, Y. (2008). Chlorella sorokiniana UTEX 2805, a heat and intense, sunlight-tolerant microalga with potential for removing ammonium from wastewater. Bioresour. Technol. 99, 4980–4989. doi: 10.1016/j.biortech.2007.09.065
de Oliveira Dal’Molin, C. G., Quek, L.-E., Palfreyman, R. W., and Nielsen, L. K. (2011). AlgaGEM – a genome-scale metabolic reconstruction of algae based on the Chlamydomonas reinhardtii genome. BMC Genomics 12:S5. doi: 10.1186/1471-2164-12-s4-s5
Deng, X.-D., Gu, B., Li, Y.-J., Hu, X.-W., Guo, J.-C., and Fei, X.-W. (2012). The roles of acyl-CoA: Diacylglycerol acyltransferase 2 genes in the biosynthesis of triacylglycerols by the green algae Chlamydomonas reinhardtii. Mol. Plant 5, 945–947. doi: 10.1093/mp/sss040
Depauw, F. A., Rogato, A., d’Alcalá, M., and Falciatore, A. (2012). Exploring the molecular basis of responses to light in marine diatoms. J. Exp. Bot. 63, 1575–1591. doi: 10.1093/jxb/ers005
Dominguez, A. A., Lim, W. A., and Qi, L. S. (2016). Beyond editing: repurposing CRISPR-Cas9 for precision genome regulation and interrogation. Nat. Rev. Mol. Cell Biol. 17, 5–15. doi: 10.1038/nrm.2015.2
Dong, Z., and Chen, Y. (2013). Transcriptomics: advances and approaches. Sci. China Life Sci. 56, 960–967. doi: 10.1007/s11427-013-4557-2
Eberhard, S., Finazzi, G., and Wollman, F.-A. (2008). The dynamics of photosynthesis. Annu. Rev. Genet. 42, 463–515. doi: 10.1146/annurev.genet.42.110807.091452
Finazzi, G., Johnson, G. N., Dall’Osto, L., Zito, F., Bonente, G., Bassi, R., et al. (2006). Nonphotochemical quenching of chlorophyll fluorescence in Chlamydomonas reinhardtii. Biochemistry 45, 1490–1498. doi: 10.1021/bi0521588
Gangl, D., Zedler, J. A. Z., Rajakumar, P. D., Martinez, E. M. R., Riseley, A., Włodarczyk, A., et al. (2015). Biotechnological exploitation of microalgae. J. Exp. Bot. 66, 6975–6990. doi: 10.1093/jxb/erv426
Gao, H., Wright, D. A., Li, T., Wang, Y., Horken, K., Weeks, D. P., et al. (2014). TALE activation of endogenous genes in Chlamydomonas reinhardtii. Algal Res. 5, 52–60. doi: 10.1016/j.algal.2014.05.003
Geider, R. J. (1987). Light and temperature dependence of the carbon to chlorophyll a ratio in microalgae and cyanobacteria: implications for physiology and growth of phytoplankton. New Phytol. 106, 1–34. doi: 10.1111/j.1469-8137.1987.tb04788.x
Genkov, T., Meyer, M., Griffiths, H., and Spreitzer, R. J. (2010). Functional hybrid rubisco enzymes with plant small subunits and algal large subunits: engineered rbcS cDNA for expression in Chlamydomonas. J. Biol. Chem. 285, 19833–19841. doi: 10.1074/jbc.M110.124230
Gimpel, J. A., Henríquez, V., and Mayfield, S. P. (2015). Metabolic engineering of eukaryotic microalgae: potential and challenges come with great diversity. Front. Microbiol. 6:1376. doi: 10.3389/fmicb.2015.01376
Gong, Y., Hu, H., Gao, Y., Xu, X., and Gao, H. (2011). Microalgae as platforms for production of recombinant proteins and valuable compounds: progress and prospects. J. Industrial Microbiol. Biotechnol. 38, 1879–1890. doi: 10.1007/s10295-011-1032-6
Goss, R., and Jakob, T. (2010). Regulation and function of xanthophyll cycle-dependent photoprotection in algae. Photosynth. Res. 106, 103–122. doi: 10.1007/s11120-010-9536-x
Hanagata, N., Takeuchi, T., Fukuju, Y., Barnes, D. J., and Karube, I. (1992). Tolerance of microalgae to high CO2 and high temperature. Phytochemistry 31, 3345–3348. doi: 10.1016/0031-9422(92)83682-O
Hanikenne, M. (2003). Chlamydomonas reinhardtii as a eukaryotic photosynthetic model for studies of heavy metal homeostasis and tolerance. New Phytol. 159, 331–340. doi: 10.1046/j.1469-8137.2003.00788.x
Hlavova, M., Turoczy, Z., and Bisova, K. (2015). Improving microalgae for biotechnology - From genetics to synthetic biology. Biotechnol. Adv. 33, 1194–1203. doi: 10.1016/j.biotechadv.2015.01.009
Ho, S.-H., Ye, X., Hasunuma, T., Chang, J.-S., and Kondo, A. (2014). Perspectives on engineering strategies for improving biofuel production from microalgae - a critical review. Biotechnol. Adv. 32, 1448–1459. doi: 10.1016/j.biotechadv.2014.09.002
Hsieh, H.-J., Su, C.-H., and Chien, L.-J. (2012). Accumulation of lipid production in Chlorella minutissima by triacylglycerol biosynthesis-related genes cloned from Saccharomyces cerevisiae and Yarrowia lipolytica. J. Microbiol. 50, 526–534. doi: 10.1007/s12275-012-2041-5
Hu, S., Lau, K. W. K., and Wu, M. (2001). Cadmium sequestration in Chlamydomonas reinhardtii. Plant Sci. 161, 987–996. doi: 10.1016/S0168-9452(01)00501-5
Iwasaki, I., Kurano, N., and Miyachi, S. (1996). Effects of high-CO2 stress on photosystem II in a green alga, Chlorococcum littorale, which has a tolerance to high CO2. J. Photochem. Photobiol. B Biol. 36, 327–332. doi: 10.1016/S1011-1344(96)07385-X
Jiang, H., and Gao, K. (2004). Effects of lowering temperature during culture on the production of polyunsaturated fatty acids in the marine diatom Phaeodactylum tricornutum (Bacillariophyceae). J. Phycol. 40, 651–654. doi: 10.1111/j.1529-8817.2004.03112.x
Jiang, W., Brueggeman, A. J., Horken, K. M., Plucinak, T. M., and Weeks, D. P. (2014). Successful transient expression of Cas9 and single guide RNA genes in Chlamydomonas reinhardtii. Eukaryot. Cell 13, 1465–1469. doi: 10.1128/EC.00213-14
Jones, C. S., and Mayfield, S. P. (2012). Algae biofuels: versatility for the future of bioenergy. Curr. Opin. Biotechnol. 23, 346–351. doi: 10.1016/j.copbio.2011.10.013
Karpowicz, S. J., Prochnik, S. E., Grossman, A. R., and Merchant, S. S. (2011). The GreenCut2 resource, a phylogenomically derived inventory of proteins specific to the plant lineage. J. Biol. Chem. 286, 21427–21439. doi: 10.1074/jbc.M111.233734
Kasai, Y., Oshima, K., Ikeda, F., Abe, J., Yoshimitsu, Y., and Harayama, S. (2015). Construction of a self-cloning system in the unicellular green alga Pseudochoricystis ellipsoidea. Biotechnol. Biofuels 8, 1–12. doi: 10.1186/s13068-015-0277-0
Kaye, Y., Grundman, O., Leu, S., Zarka, A., Zorin, B., Didi-Cohen, S., et al. (2015). Metabolic engineering toward enhanced LC-PUFA biosynthesis in Nannochloropsis oceanica: overexpression of endogenous Δ12 desaturase driven by stress-inducible promoter leads to enhanced deposition of polyunsaturated fatty acids in TAG. Algal Res. 11, 387–398. doi: 10.1016/j.algal.2015.05.003
Kim, E.-J., Ma, X., and Cerutti, H. (2015). Gene silencing in microalgae: mechanisms and biological roles. Bioresour. Technol. 184, 23–32. doi: 10.1016/j.biortech.2014.10.119
Kirst, G. O. (1990). Salinity tolerance of eukaryotic marine algae. Annu. Rev. Plant Physiol. Plant Mol. Biol. 41, 21–53. doi: 10.1146/annurev.pp.41.060190.000321
Kobayashi, Y., Harada, N., Nishimura, Y., Saito, T., Nakamura, M., Fujiwara, T., et al. (2014). Algae sense exact temperatures: small heat shock proteins are expressed at the survival threshold temperature in Cyanidioschyzon merolae and Chlamydomonas reinhardtii. Genome Biol. Evol. 6, 2731–2740. doi: 10.1093/gbe/evu216
Lamers, P. P., Janssen, M., De Vos, R. C. H., Bino, R. J., and Wijffels, R. H. (2012). Carotenoid and fatty acid metabolism in nitrogen-starved Dunaliella salina, a unicellular green microalga. J. Biotechnol. 162, 21–27. doi: 10.1016/j.jbiotec.2012.04.018
Lee, D.-J., Chang, J.-S., and Lai, J.-Y. (2015). Microalgae–microbial fuel cell: a mini review. Bioresour. Technol. 198, 891–895. doi: 10.1016/j.biortech.2015.09.061
Lemaire, S., Keryer, E., Stein, M., Schepens, I., Issakidis-Bourguet, E., Gérard-Hirne, C., et al. (1999). Heavy-metal regulation of thioredoxin gene expression in Chlamydomonas reinhardtii. Plant Physiol. 120, 773–778. doi: 10.1104/pp.120.3.773
Lemoine, Y., and Schoefs, B. (2010). Secondary ketocarotenoid astaxanthin biosynthesis in algae: a multifunctional response to stress. Photosynth. Res. 106, 155–177. doi: 10.1007/s11120-010-9583-3
Li, X., Zhang, R., Patena, W., Gang, S. S., Blum, S. R., Ivanova, N., et al. (2016). An indexed, mapped mutant library enables reverse genetics studies of biological processes in Chlamydomonas reinhardtii. Plant Cell 28, 367–387. doi: 10.1105/tpc.15.00465
Li, Y., Huang, J., Sandmann, G., and Chen, F. (2009). High-light and sodium chloride stress differentially regulate the biosynthesis of astaxanthin in Chlorella zofingiensis (Chlorophyceae). J. Phycol. 45, 635–641. doi: 10.1111/j.1529-8817.2009.00689.x
Liska, A. J., Shevchenko, A., Pick, U., and Katz, A. (2004). Enhanced photosynthesis and redox energy production contribute to salinity tolerance in Dunaliella as revealed by homology-based proteomics. Plant Physiol. 136, 2806–2817. doi: 10.1104/pp.104.039438
Lopez, D., Casero, D., Cokus, S. J., Merchant, S. S., and Pellegrini, M. (2011). Algal functional annotation tool: a web-based analysis suite to functionally interpret large gene lists using integrated annotation and expression data. BMC Bioinform. 12:282. doi: 10.1186/1471-2105-12-282
Lusser, M., Parisi, C., Plan, D., and Rodríguez-Cerezo, E. (2012). Deployment of new biotechnologies in plant breeding. Nat. Biotechnol. 30, 231–239. doi: 10.1038/nbt.2142
Markou, G., and Nerantzis, E. (2013). Microalgae for high-value compounds and biofuels production: a review with focus on cultivation under stress conditions. Biotechnol. Adv. 31, 1532–1542. doi: 10.1016/j.biotechadv.2013.07.011
Maruyama, S., Tokutsu, R., and Minagawa, J. (2014). Transcriptional regulation of the stress-responsive light harvesting complex genes in Chlamydomonas reinhardtii. Plant Cell Physiol. 55, 1304–1310. doi: 10.1093/pcp/pcu068
Mayank, A., Venkatesh, J., and Tran, L. S. P. (2015). Regulation of photosynthesis during abiotic stress-induced photoinhibition. Mol. Plant 8, 1304–1320. doi: 10.1016/j.molp.2015.05.005
Mayfield, S., and Golden, S. (2015). Photosynthetic bio-manufacturing: food, fuel, and medicine for the 21st century. Photosynth. Res. 123, 225–226. doi: 10.1007/s11120-014-0063-z
Miyachi, S., Iwasaki, I., and Shiraiwa, Y. (2003). Historical perspective on microalgal and cyanobacterial acclimation to low- and extremely high-CO2 conditions. Photosynth. Res. 77, 139–153. doi: 10.1023/a:1025817616865
Morozova, O., and Marra, M. A. (2008). Applications of next-generation sequencing technologies in functional genomics. Genomics 92, 255–264. doi: 10.1016/j.ygeno.2008.07.001
Müller, P., Li, X.-P., and Niyogi, K. K. (2001). Non-photochemical quenching. A response to excess light energy. Plant Physiol. 125, 1558–1566. doi: 10.1104/pp.125.4.1558
Mussgnug, J. H., Thomas-Hall, S., Rupprecht, J., Foo, A., Klassen, V., McDowall, A., et al. (2007). Engineering photosynthetic light capture: impacts on improved solar energy to biomass conversion. Plant Biotechnol. J. 5, 802–814. doi: 10.1111/j.1467-7652.2007.00285.x
Noor-Mohammadi, S., Pourmir, A., and Johannes, T. W. (2012). Method to assemble and integrate biochemical pathways into the chloroplast genome of Chlamydomonas reinhardtii. Biotechnol. Bioeng. 109, 2896–2903. doi: 10.1002/bit.24569
Perales-Vela, H. V., Peña-Castro, J. M., and Cañizares-Villanueva, R. O. (2006). Heavy metal detoxification in eukaryotic microalgae. Chemosphere 64, 1–10. doi: 10.1016/j.chemosphere.2005.11.024
Qin, S., Lin, H., and Jiang, P. (2012). Advances in genetic engineering of marine algae. Biotechnol. Adv. 30, 1602–1613. doi: 10.1016/j.biotechadv.2012.05.004
Quaas, T., Berteotti, S., Ballottari, M., Flieger, K., Bassi, R., Wilhelm, C., et al. (2015). Non-photochemical quenching and xanthophyll cycle activities in six green algal species suggest mechanistic differences in the process of excess energy dissipation. J. Plant Physiol. 172, 92–103. doi: 10.1016/j.jplph.2014.07.023
Rajamani, S., Siripornadulsil, S., Falcao, V., Torres, M., Colepicolo, P., and Sayre, R. (2007). “Phycoremediation of heavy metals using transgenic microalgae,” in Transgenic Microalgae as Green Cell Factories, eds R. León, A. Galván, and E. Fernández (New York, NY: Springer), 99–109.
Rasala, B. A., Chao, S.-S., Pier, M., Barrera, D. J., and Mayfield, S. P. (2014). Enhanced genetic tools for engineering multigene traits into green algae. PLoS ONE 9:e94028. doi: 10.1371/journal.pone.0094028
Rasala, B. A., Muto, M., Sullivan, J., and Mayfield, S. P. (2011). Improved heterologous protein expression in the chloroplast of Chlamydomonas reinhardtii through promoter and 5’ untranslated region optimization. Plant Biotechnol. J. 9, 674–683. doi: 10.1111/j.1467-7652.2011.00620.x
Raven, J. A. (2010). Inorganic carbon acquisition by eukaryotic algae: four current questions. Photosynth. Res. 106, 123–134. doi: 10.1007/s11120-010-9563-7
Renaud, S. M., Thinh, L.-V., Lambrinidis, G., and Parry, D. L. (2002). Effect of temperature on growth, chemical composition and fatty acid composition of tropical Australian microalgae grown in batch cultures. Aquaculture 211, 195–214. doi: 10.1016/S0044-8486(01)00875-4
Roháček, K., Bertrand, M., Moreau, B., Jacquette, B., Caplat, C., Morant-Manceau, A., et al. (2014). Relaxation of the non-photochemical chlorophyll fluorescence quenching in diatoms: kinetics, components and mechanisms. Philos. Trans. R. Soc. Lond. B Biol. Sci. 369:20130241. doi: 10.1098/rstb.2013.0241
Sakai, N., Sakamoto, Y., Kishimoto, N., Chihara, M., and Karube, I. (1995). Chlorella strains from hot springs tolerant to high temperature and high CO2. Energy Convers. Manag. 36, 693–696. doi: 10.1016/0196-8904(95)00100-R
Scibilia, L., Girolomoni, L., Berteotti, S., Alboresi, A., and Ballottari, M. (2015). Photosynthetic response to nitrogen starvation and high light in Haematococcus pluvialis. Algal Res. 12, 170–181. doi: 10.1016/j.algal.2015.08.024
Scranton, M. A., Ostrand, J. T., Fields, F. J., and Mayfield, S. P. (2015). Chlamydomonas as a model for biofuels and bio-products production. Plant J. 82, 523–531. doi: 10.1111/tpj.12780
Scranton, M. A., Ostrand, J. T., Georgianna, D. R., Lofgren, S. M., Li, D., Ellis, R. C., et al. (2016). Synthetic promoters capable of driving robust nuclear gene expression in the green alga Chlamydomonas reinhardtii. Algal Res. 15, 135–142. doi: 10.1016/j.algal.2016.02.011
Siripornadulsil, S., Traina, S., Verma, D. P. S., and Sayre, R. T. (2002). Molecular mechanisms of Proline-mediated tolerance to toxic heavy metals in transgenic microalgae. Plant Cell 14, 2837–2847. doi: 10.1105/tpc.004853
Sizova, I., Greiner, A., Awasthi, M., Kateriya, S., and Hegemann, P. (2013). Nuclear gene targeting in Chlamydomonas using engineered zinc-finger nucleases. Plant J. 73, 873–882. doi: 10.1111/tpj.12066
Solovchenko, A., and Khozin-Goldberg, I. (2013). High-CO2 tolerance in microalgae: possible mechanisms and implications for biotechnology and bioremediation. Biotechnol. Lett. 35, 1745–1752. doi: 10.1007/s10529-013-1274-7
Steinbrenner, J., and Sandmann, G. (2006). Transformation of the green alga Haematococcus pluvialis with a phytoene desaturase for accelerated astaxanthin biosynthesis. Appl. Environ. Microbiol. 72, 7477–7484. doi: 10.1128/AEM.01461-06
Stengel, D. B., Connan, S., and Popper, Z. A. (2011). Algal chemodiversity and bioactivity: sources of natural variability and implications for commercial application. Biotechnol. Adv. 29, 483–501. doi: 10.1016/j.biotechadv.2011.05.016
Sun, G., Zhang, X., Sui, Z., and Mao, Y. (2008). Inhibition of pds gene expression via the RNA interference approach in Dunaliella salina (Chlorophyta). Mar. Biotechnol. 10, 219–226. doi: 10.1007/s10126-007-9056-7
Sung, K.-D., Lee, J.-S., Shin, C.-S., Park, S.-C., and Choi, M.-J. (1999). CO2 fixation by Chlorella sp. KR-1 and its cultural characteristics. Bioresour. Technol. 68, 269–273. doi: 10.1016/S0960-8524(98)00152-7
Suresh Kumar, K., Dahms, H.-U., Won, E.-J., Lee, J.-S., and Shin, K.-H. (2015). Microalgae – A promising tool for heavy metal remediation. Ecotoxicol. Environ. Saf. 113, 329–352. doi: 10.1016/j.ecoenv.2014.12.019
Tardif, M., Atteia, A., Specht, M., Cogne, G., Rolland, N., Brugière, S., et al. (2012). PredAlgo: a new subcellular localization prediction tool dedicated to green algae. Mol. Biol. Evol. 29, 3625–3639. doi: 10.1093/molbev/mss178
Tissot-Lecuelle, G., Purton, S., Dubald, M., and Goldschmidt-Clermont, M. (2014). “Synthesis of recombinant products in the chloroplast,” in Plastid Biology, eds S. M. Theg and F.-A. Wollman (New York, NY: Springer), 517–557.
Torricelli, E., Gorbi, G., Pawlik-Skowronska, B., di Toppi, L. S., and Corradi, M. G. (2004). Cadmium tolerance, cysteine and thiol peptide levels in wild type and chromium-tolerant strains of Scenedesmus acutus (Chlorophyceae). Aquat. Toxicol. 68, 315–323. doi: 10.1016/j.aquatox.2004.03.020
Tsai, C.-H., Warakanont, J., Takeuchi, T., Sears, B. B., Moellering, E. R., and Benning, C. (2014). The protein compromised hydrolysis of triacylglycerols 7 (CHT7) acts as a repressor of cellular quiescence in Chlamydomonas. Proc. Natl. Acad. Sci. U.S.A. 111, 15833–15838. doi: 10.1073/pnas.1414567111
Tsuji, N., Hirayanagi, N., Okada, M., Miyasaka, H., Hirata, K., Zenk, M. H., et al. (2002). Enhancement of tolerance to heavy metals and oxidative stress in Dunaliella tertiolecta by Zn-induced phytochelatin synthesis. Biochem. Biophys. Res. Commun. 293, 653–659. doi: 10.1016/S0006-291X(02)00265-6
USDA Japan Reports (2014). United States Department of Agriculture. Japan takes step Forward to Improve its GE Product Review Process. Available at: usdajapan.org/en/reports/reports2014.html
Waters, E., and Rioflorido, I. (2007). Evolutionary analysis of the small heat shock proteins in five complete algal genomes. J. Mol. Evol. 65, 162–174. doi: 10.1007/s00239-006-0223-7
Whitney, S. M., Houtz, R. L., and Alonso, H. (2011). Advancing our understanding and capacity to engineer nature’s CO2-sequestering enzyme. Rubisco. Plant Physiol. 155, 27–35. doi: 10.1104/pp.110.164814
Wobbe, L., Bassi, R., and Kruse, O. (2016). Multi-level light capture control in plants and green algae. Trends Plant Sci. 21, 55–68. doi: 10.1016/j.tplants.2015.10.004
Wu, S., Xu, L., Huang, R., and Wang, Q. (2011). Improved biohydrogen production with an expression of codon-optimized hemH and lba genes in the chloroplast of Chlamydomonas reinhardtii. Bioresour. Technol. 102, 2610–2616. doi: 10.1016/j.biortech.2010.09.123
Yao, L., Tan, T. W., Ng, Y.-K., Ban, K. H. K., Shen, H., Lin, H., et al. (2015). RNA-Seq transcriptomic analysis with Bag2D software identifies key pathways enhancing lipid yield in a high lipid-producing mutant of the non-model green alga Dunaliella tertiolecta. Biotechnol. Biofuels 8, 1–16. doi: 10.1186/s13068-015-0382-0
Young, R. E. B., and Purton, S. (2015). Codon reassignment to facilitate genetic engineering and biocontainment in the chloroplast of Chlamydomonas reinhardtii. Plant Biotechnol. J. doi: 10.1111/pbi.12490 [Epub ahead of print].
Zhang, J., Hao, Q., Bai, L., Xu, J., Yin, W., Song, L., et al. (2014). Overexpression of the soybean transcription factor GmDof4 significantly enhances the lipid content of Chlorella ellipsoidea. Biotechnol. Biofuels 7, 1–16. doi: 10.1186/s13068-014-0128-4
Keywords: microalgae, abiotic stresses, genetic engineering, strain improvement, potential applications
Citation: Guihéneuf F, Khan A and Tran L-SP (2016) Genetic Engineering: A Promising Tool to Engender Physiological, Biochemical, and Molecular Stress Resilience in Green Microalgae. Front. Plant Sci. 7:400. doi: 10.3389/fpls.2016.00400
Received: 08 February 2016; Accepted: 14 March 2016;
Published: 31 March 2016.
Edited by:
Mohammad Anwar Hossain, Bangladesh Agricultural University, BangladeshReviewed by:
Benoit Schoefs, University of Maine, FranceBoris Zorin, Ben-Gurion University of the Negev, Israel
Copyright © 2016 Guihéneuf, Khan and Tran. This is an open-access article distributed under the terms of the Creative Commons Attribution License (CC BY). The use, distribution or reproduction in other forums is permitted, provided the original author(s) or licensor are credited and that the original publication in this journal is cited, in accordance with accepted academic practice. No use, distribution or reproduction is permitted which does not comply with these terms.
*Correspondence: Lam-Son P. Tran, c29udHJhbkB0ZHQuZWR1LnZu; c29uLnRyYW5AcmlrZW4uanA=
†These authors have contributed equally to this work.