- 1State Key Laboratory of Soil and Sustainable Agriculture, Institute of Soil Science, Chinese Academy of Sciences, Nanjing, China
- 2Department of Biological Sciences, University of Toronto, Toronto, ON, Canada
Iron (Fe) is an essential micronutrient for plant growth and development, and is frequently limiting. By contrast, over-accumulation of Fe in plant tissues leads to toxicity. In soils, the distribution of Fe is highly heterogeneous. To cope with this heterogeneity, plant roots engage an array of adaptive responses to adjust their morphology and physiology. In this article, we review root morphological and physiological changes in response to low- and high-Fe conditions and highlight differences between these responses. We especially focus on the role of the root apex in dealing with the stresses resulting from Fe shortage and excess.
Introduction
Iron (Fe) is an essential element, critical to the key primary processes of photosynthesis, respiration, and nitrogen metabolism, and species differ greatly in how much Fe they require for optimal growth. Fe exists in two oxidation states, Fe3+ (ferric) and Fe2+ (ferrous), and rates of absorption and toxicities for the two forms differ significantly, typically with Fe2+ showing higher absorption and toxicity (Becana et al., 1998; Fageria et al., 2008; Brumbarova et al., 2015; Li et al., 2015b). Fe is present in excess in soils of low pH and oxygen tension (Connolly and Guerinot, 2002; Becker and Asch, 2005), while it is frequently limiting in alkaline and aerobic soils (Imsande, 1998; Eroglu et al., 2016). Therefore, knowledge of the mechanisms by which plants maintain Fe homeostasis is of great biological importance and agronomic significance. Root adaptations to Fe limitation and toxicity occur at both morphological and physiological levels. The root apex, the initiator organ of root tropisms, cell polarity, and fate (Baluška et al., 1990), represents the most probable site for sensing Fe limitation and excess. Here, we outline recent progress in our understanding of how plant roots respond to low and high Fe, and especially highlight the mechanisms involving the root apex in these responses.
Morphological Changes
Plants can respond to suboptimal environmental conditions by growth redistribution within their root system architecture (RSA), a process referred to as the stress-induced morphogenic response (Potters et al., 2009; Zolla et al., 2010; De Smet et al., 2015). Nutrient availability can exert a profound impact on RSA by altering the number, length, angle, and diameter of roots and root hairs (for review, see Forde and Lorenzo, 2001; López-Bucio et al., 2003; Osmont et al., 2007; Giehl et al., 2014). By allocating carbon flow to facilitate directional root growth to more favorable soil patches, plants can respond to the heterogeneous availability of nutrients flexibly and rapidly (Zhang and Forde, 1998; Hermans et al., 2006; Giehl et al., 2012a,b; Gruber et al., 2013). Excess Fe has been shown to arrest primary root (PR) growth by decreasing both cell elongation and division, and to inhibit lateral root (LR) initiation in newly grown roots elongating during exposure to excess Fe, sparing proximal roots formed prior to excess-Fe exposure (Yoshida, 1981; Yamauchi and Peng, 1995; Li et al., 2012, 2015a,b; Reyt et al., 2015), resulting from direct contact of the root tip with Fe. Fe2+, the most toxic form, is increasingly present in lower soil strata, where low pH and anoxia prevail (Ratering and Schnell, 2000). Thus, an adjustment of RSA to restrict excessive Fe absorption in lower strata can prevent more serious Fe toxicity, while still allowing for the acquisition of essential nutrients in other parts of the root system. Supportive of this, excess Fe was seen to have no significant effect on LR formation in the proximal roots, with relatively stable LR number and length in this portion of the root system, to which the absorption of other nutrients can be delegated when other components of the root system are under stress, allowing controlled acclimation to nutritional stress (Heil and Baldwin, 2002). Furthermore, root gravitropism can rapidly alter root growth orientation when the root tip is exposed to Fe stress, and this can modify the direction of root growth away from the stress stimulus (Wisniewska et al., 2006; Zou et al., 2012, 2013; Li et al., 2015a). By contrast, to cope with Fe deficiency, plants can increase the exchange surface of the root system, thus enhancing its foraging capacity. A shortage in Fe triggers the formation of ectopic root hairs at positions normally occupied by non-hair cells and leads to bi-furcated root hairs with two tips (Schmidt et al., 2000). This peculiar phenotype plays an important role in Fe uptake by increasing the absorptive surface layer of the root. Similarly, Gruber et al. (2013) suggested that the length of the PR and the number of LRs both increase under moderate Fe deficiency, while they drastically decrease under severe Fe deficiency. Furthermore, Giehl et al. (2012a,b) showed Fe limitation affects RSA by regulating root-tip auxin and Fe content to promote LR elongation in Fe-enriched zones. Thus, at least under moderate Fe deficiency, the plasticity in the root system’s response offers the adaptive advantage of exploiting more distantly located Fe patches in soils; however, under severe Fe deficiency, acclimation proceeds via a growth-dependent pathway that transiently arrests root elongation and number to reduce nutrient demand (Seguela et al., 2008). This is similar to what is observed under phosphate (Pi) deficiency: Pi deprivation also requires continued root growth, whereas an inhibition of cell-cycle activity represses Pi uptake in response to decreased Pi demand (Lai et al., 2007).
Physiological Changes
To ensure effective Fe acquisition from the rhizosphere under Fe deficiency, plants have developed two principal strategies: Strategy I and Strategy II (Marschner et al., 1986; Romheld and Marschner, 1986; Schmidt, 2003). The initial step in strategy I is to acidify the rhizosphere, through increased proton efflux via plasma-membrane (PM) H+-ATPases (Schmidt, 2006; Santi and Schmidt, 2009). The root tip is an important regulatory region modulating H+ secretion (Haruta and Sussman, 2012; Xu et al., 2012). In Arabidopsis, FRO2 (ferric chelate reductase, FRO) and IRT1 (Fe-regulated transporter, IRT) genes encode a ferric reductase and an Fe transporter engaged in Fe acquisition from acidified media, respectively (Eide et al., 1996; Robinson et al., 1999; Jeong and Guerinot, 2009). An Fe-regulated (Fer)-like Fe-deficiency-induced transcription factor (FIT), heterodimerizes with at least four bHLH transcription factors, and directly binds to a subset of Fe-regulated genes in the root, including FRO2 and IRT1, driving their up-regulation under low Fe (Colangelo and Guerinot, 2004; Walker and Connolly, 2008; Sivitz et al., 2012; Wang et al., 2013). The interplay between hormones [ethylene, auxin, jasmonic acid (JA), cytokinins (CK), brassinosteroids (BR), abscisic acid (ABA)] and nitric oxide (NO) is also critical in Fe-deficiency signaling (see the reviews by Jeong and Guerinot, 2009; Brumbarova et al., 2015; Figure 1). In grasses, a different strategy for Fe uptake has developed, known as Strategy II (Takagi et al., 1984; Marschner et al., 1986; Shi et al., 1988; Zhang et al., 1989; Kobayashi and Nishizawa, 2012). Strategy-II plants synthesize and release compounds such as mugineic acids (MAs) into the rhizosphere to solubilize Fe and chelate ferric Fe (Shi and Liu, 1991; Walker and Connolly, 2008; Kobayashi and Nishizawa, 2012); S-adenosylmethionine is the first substrate in the mugineic acid biosynthesis pathway (Ma et al., 1995; Mori, 1999). Exudation of organic acids from the growing root tip is well documented in other stress-resistance mechanisms, such as Al stress (Kochian et al., 2004; Sivaguru et al., 2013). Recently, several studies have shown that soil microbial activity, influenced by root exudates, can further impact Fe acquisition (Rroco et al., 2003; Jin et al., 2010). Phenolics have also been identified in Fe-deficiency-induced root exudates (Jin et al., 2014), although the precise constituents remain poorly understood. Additional complexities arise when plants are mycorrhizal; in maize, for instance, strong induction of two Fe transporters, OPT8a and OPT8b, bypassing Strategy II, by mycorrhizal colonization was documented (Kobae et al., 2014). Thus, much needs to be learned about rhizospheric microbial partners and their roles in Fe acquisition.
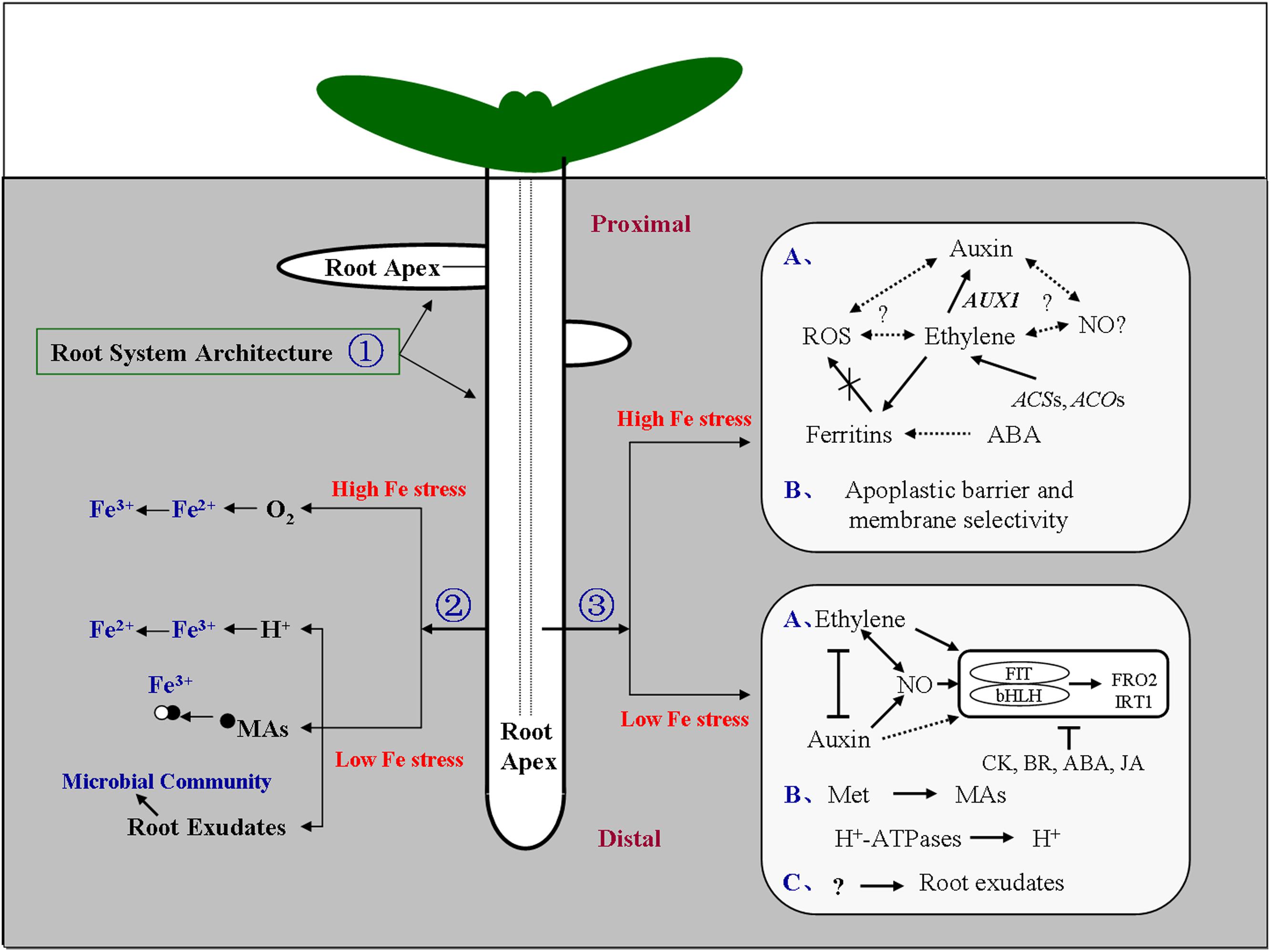
FIGURE 1. Model of the signaling responses under iron (Fe) stress in the root. Signal locations and physiological pathways of Fe stress are shown. The soil distribution of Fe is highly heterogeneous, Fe stress occurs in both low-Fe and high-Fe environments. To cope with this heterogeneity, plant roots, and in particular the root apex, engage an array of adaptive responses to adjust their morphology and physiology. ① Dynamic changes in root system architecture (RSA) could regulate the exchange surface of the root to enhance or restrict Fe absorption in low-Fe and high-Fe environments, respectively, or alter root growth orientation. ② Roots could secrete protons, and acids such as mugineic acids, and regulate the microbial communities in the rhizosphere to enhance Fe available under deficiency, and facilitate diffusion of molecular oxygen to the root medium to oxidize Fe2+ to Fe3+ under Fe2+ excess. ③ Hormones and other molecules could act in concert, and either downstream or upstream of one other, to affect the Fe-uptake gene expression, tissue Fe homeostasis, and cell-wall components, in response to low-Fe or high-Fe environments (Brumbarova et al., 2015; Li et al., 2015a,b). The root apex represents the most probable site for sensing Fe limitation and excess, and plays an important role in perceiving and transducing Fe signals into physiological and developmental responses. Arrows indicate promotion, and perpendicular lines indicate inhibition. Broken arrows indicate a potential effect or interaction. (A–C) represent each response pathway. ABA, abscisic acid; ACO, 1-aminocyclopropane-1-carboxylic acid oxidase; ACS, 1-aminocyclopropane-1-carboxylic acid synthase; AUX1, auxin-resistant 1; bHLH, basic helix-loop-helix; BR, brassinosteroids; CK, cytokinins; FIT, Fer-like Fe-deficiency-induced transcription factor; FRO, ferric chelate reductase; IRT, Fe-regulated transporter; JA, jasmonic acid; MAs, mugineic acid family; Met, methionine; NO, nitric oxide; O2, molecular oxygen; ROS, reactive oxygen species. Proximal, the root portion that existed at the time of transfer; Distal, the root portion that was established after transfer.
Studies in rice growing under flooded conditions suggest a development of oxidic mechanisms to cope with adverse Fe-toxic conditions. Rice roots diffuse molecular oxygen to the root medium, rendering the rhizosphere significantly more oxidative than bulk soil (Chen et al., 1980a,b). Rhizospheric oxidation to Fe3+ ensues, which helps keep root-medium Fe2+ low and is instrumental to the formation of an oxidized ‘iron plaque’ layer on the root surface to limit Fe uptake. Furthermore, limiting excessive tissue Fe accumulation under excess Fe supply engages apoplastic and symplastic mechanisms. About 90% of Fe2+ is typically intercepted by the root apoplast, although the mechanism of interception is only partially understood (McLaughlin et al., 1985). One explanation is that Fe2+ availability in the apoplast is reduced via alkalinization of apoplastic pH (Kosegarten et al., 2004), which affects both Fe2+ mobility and chemical stability, and this alkalinization can be affected by ethylene (Staal et al., 2011). Another possible hypothesis relates to the cation-exchange capacity of the cell wall. The cell wall acts as a major pool of calcium, aluminum, cadmium, and other cations, cell-wall components with negative surface charges (pectin and hemicelluloses) possess cation-adsorption capacities, and cations compete for these surface charges with variable potency (Kronzucker et al., 1995). It has been reported that ethylene, auxin, and NO play roles in the cell-wall adjustment to stress (Xiong et al., 2009; Tsang et al., 2011; Zhu et al., 2013). Fe2+ can also be excluded at root-cell membranes (Tadano, 1975). Additionally, plants can sequester Fe2+ in root-cell vacuoles and in the multimeric protein ferritin (Connolly and Guerinot, 2002; Majerus et al., 2007, 2009). In maize (Zea mays L.) and Arabidopsis, regulation of ferritin gene expression in response to Fe excess occurs at the transcriptional level (Briat et al., 2010; Li et al., 2015b), and involves regulatory pathways mediated by ABA, reactive oxygen species (ROS), and ethylene. However, Majerus et al. (2009) showed that a signaling pathway leading to the induction of ferritin synthesis depended neither on ABA nor oxidative stress in African rice.
Response Mechanism of the Root Apex
Identifying the stress-sensing site is as critical under Fe stress as under other stresses. As the root apex is the first part of the plant to come into contact with previously unexplored soil regions, the tip represents the most probable candidate (Dat et al., 2004; Baluška et al., 2010). Growth-inhibitory effects of low phosphate (Svistoonoff et al., 2007; Ward et al., 2008) or elevated aluminum (Ryan et al., 1993; Jones and Kochian, 1995; Baluška and Mancuso, 2013; Sivaguru et al., 2013) are sensed by the root tip. The root tip transition zone was also suggested as a primary site in sensing and transducing nitrate signals (Trevisan et al., 2015). Some receptors for environmental signals have been reported to be strongly expressed in the root tip, such as, LPR1, which senses low Pi (Svistoonoff et al., 2007; Müller et al., 2015). The root tip has received much attention devoted to the action of hormones and other molecules. Plant hormones and other molecules could act in concert, and either downstream or upstream of one other. With more evidence accumulating supporting the involvement of different molecules in Fe signaling, a necessary task will be to determine how they integrate into the larger signaling network. This will also contribute to our understanding of exactly how the root tip can act as a signaling-response nexus. Fe excess leads to a significant decrease in PR length, and recent results have shown that contact of the PR tip with Fe is both necessary and sufficient for PR inhibition (Zhang et al., 2011, 2012; Li et al., 2015b). Fe excess could modulate the H2O2/O2⋅- balance, decreasing O2⋅- in the root tip proliferation zone and increasing H2O2 production in the transition zone, to arrest PR growth (Reyt et al., 2015), as suggested by the model by Tsukagoshi et al. (2010), which correlates PR growth with the relative distribution of O2⋅- and H2O2 in the tip. Meanwhile, ethylene evolution is enhanced by upregulating expression of ACS and ACO genes in the root tip and protects root growth under Fe toxicity by regulating tissue Fe homeostasis (Harahap et al., 2014; Li et al., 2015b). Despite the well-known functions of ethylene and ROS signaling during a variety of abiotic stresses, whether ethylene acts alone or in conjunction with ROS in root-tip acclimation to Fe excess remains to be elucidated. It has been reported that enhanced NO generation in the root transition zone is required for maintaining root growth under cadmium stress (Alemayehu et al., 2015). However, a clear role for root-tip NO in regulating root growth under Fe excess has not yet bee established (see below). The root tip is also the primary sensing site for the LR formation response to excess Fe, and reduced LR formation in response to excess Fe was found to be partially related to auxin levels, while root-tip PIN2 protein expression and ethylene-related AUX1 functions were shown to play a positive role on LR formation under Fe excess (Li et al., 2015a). Furthermore, LR development also requires ROS signaling (Manzano et al., 2014). Arabidopsis seedlings exposed to oxidative stress-inducing agents display clear modifications in auxin homeostasis, suggesting a possible crosstalk between ROS and auxin signals (Cheng et al., 2011; Yuan et al., 2013). This may constitute a signaling intersection point within the root tip to meditate intelligent growth responses to Fe excess. The PIN2 gene, critical to routing signals to either the root or shoot apex, is seen as a general stress target due to its strong sensitivity to a variety of environmental stresses, such as cold, salt, and aluminum, supporting root stress avoidance (Baluška et al., 2010). Auxin distribution within the root under Fe stress responds to alterations in PIN2 gene expression in the root tip (Li et al., 2015a), and this may modify the direction of root growth away from the stress stimulus (Sun et al., 2008). Furthermore, ROS and NO signaling in the root apex are also implicated as an early response in gravitropic acclimation (Mugnai et al., 2014). Fe2+ presence is increased by hypoxic or anoxic conditions (Mongon et al., 2014), and the transition zone seems to be the most sensitive region of the root to oxygen deprivation (McLamore et al., 2010; Larsen et al., 2015). Moreover, local NO peaks in the transition zone are essential to the successful acclimation of the entire root to oxygen deprivation (Mugnai et al., 2012)
Similarly, under Fe deficiency, Giehl et al. (2012a,b) found that locally supplied Fe evokes RSA modifications and affects the local symplastic Fe gradient in LRs, upregulating the AUX1 gene to accumulate auxin in LR tips as a prerequisite for LR elongation. Thus, AUX1 may represent a check-point at which systemic and local nutritional signals are integrated into the overall root developmental program (Giehl et al., 2014). Proton secretion is regulated in the response to Fe deficiency and maintains or promotes PR elongation and root hair development (Santi and Schmidt, 2009; Yang et al., 2010; Xu et al., 2013). Several studies have shown that the root tip plays an important role in the response to Fe stress by mediating proton and organic acids secretion ((López-Millán et al., 2000; Abadia et al., 2002). In addition, NO levels are altered in response to Fe availability in the root tip and have been invoked in signal transmission (Chen et al., 2010; Romera et al., 2011). Although NO interacts with ethylene and auxin (Freschi, 2013), the details of this mechanism remain largely unknown. Reporter gene studies have shown that ethylene and auxin can act antagonistically in regulating the topology of IRT1 gene expression along the root (Blum et al., 2014). These suggest that the root apex serves as the sensing site initiating the growth response to both low and excess Fe, shortly after the root apex reaches the heterogeneous Fe zone, triggering a sequence of signaling events. To further understand the interactions of the various signaling pathways and of the gene-regulatory network in the root-tip response to Fe, future work will need to combine genomic and genetic approaches. For example, Trevisan et al. (2015) confirmed the root tip transition zone as a critical zone in sensing nitrate using genome-wide studies and postulated the contribution of NO to the nitrate-induced transcriptional response in the transition zone. Moreover, Satbhai and Busch (2014) used a set of 450 natural accessions of Arabidopsis to identify genes that quantitatively regulate root growth responses to Fe deficiency using genome-wide association mapping, and more than 20 genomic loci were found to be significantly associated with changes in root growth rate upon Fe deficiency.
Conclusion
Recently, important progress has been made in our understanding of how plants maintain Fe homeostasis in response to heterogeneous Fe supply in soils. Many of the morphological and physiological responses are now understood, and various regulatory factors have been shown to take part in the sensing of soil-Fe stress, both in low-Fe and in high-Fe environments, and many of the components of the signaling pathways engaged have been mapped out (Figure 1). This working model should provide important new insight into plant responses to heterogeneous Fe supply. Our knowledge of the molecular components involved in the Fe-stress response is in its infancy, and details of signal transduction, such as the precise identification of sensors and transcription factors, remain a challenge. The root apex has emerged as the primary sensing site for Fe stress, and it is hoped that future work will elucidate how apex sensing integrates into whole-plant signaling and translates into an intelligent root-system-architecture response to Fe stress.
Author Contributions
GL drafted the manuscript. GL, HK, and WS revised the manuscript.
Conflict of Interest Statement
The authors declare that the research was conducted in the absence of any commercial or financial relationships that could be construed as a potential conflict of interest.
Acknowledgments
This work was supported by the Strategic Priority Research Program (B) - “Soil-Microbial System Function and Regulation” of the Chinese Academy of Sciences (XDB15030100), National Natural Science Foundation of China (31430095 and 31300210) and the Natural Sciences and Engineering Research Council of Canada (NSERC, Discovery Grant 217277-2009).
References
Abadia, J., Lopez-Millan, A. F., Rombol, A., and Abadia, A. (2002). Organic acids and Fe deficiency: a review. Plant Soil 241, 75–86. doi: 10.1023/A:1016093317898
Alemayehu, A., Zelinová, V., Bočová, B., Huttová, J., Mistrík, I., and Tamás, L. (2015). Enhanced nitric oxide generation in root transition zone during the early stage of cadmium stress is required for maintaining root growth in barley. Plant Soil 390, 213–222. doi: 10.1007/s11104-015-2397-5
Baluška, F., Kubica,Š., and Hauskrecht, M. (1990). Postmitotic ‘isodiametric’ cell growth in the maize root apex. Planta 181, 269–274. doi: 10.1007/BF00195876
Baluška, F., and Mancuso, S. (2013). Root apex transition zone as oscillatory zone. Front. Plant Sci. 4:354. doi: 10.3389/fpls.2013.00354
Baluška, F., Mancuso, S., Volkmann, D., and Barlow, P. W. (2010). Root apex transition zone: a signalling-response nexus in the root. Trends Plant Sci. 15, 402–408. doi: 10.1016/j.tplants.2010.04.007
Becana, M., Moran, J. F., and Iturbe-Ormaetxe, I. (1998). Iron-dependent oxygen free radical generation in plants subjected to environmental stress: toxicity and antioxidant protection. Plant Soil 201, 137–147. doi: 10.1023/A:1004375732137
Becker, M., and Asch, F. (2005). Iron toxicity in rice-conditions and management concepts. J. Plant Nutr. Soil Sci. 168, 558–573. doi: 10.1002/jpln.200520504
Blum, A., Brumbarova, T., Bauer, P., and Ivanov, R. (2014). Hormone influence on the spatial regulation of IRT1 expression in iron-deficient Arabidopsis thaliana roots. Plant Signal. Behav. 9:e2878. doi: 10.4161/psb.28787
Brumbarova, T., Bauer, P., and Ivanov, R. (2015). Molecular mechanisms governing Arabidopsis iron uptake. Trends Plant Sci. 20, 1360–1385. doi: 10.1016/j.tplants.2014.11.004
Briat, J. F., Ravet, K., Arnaud, N., Duc, C., Boucherez, J., Touraine, B., et al. (2010). New insights into ferritin synthesis and function highlight a link between iron homeostasis and oxidative stress in plants. Ann. Bot. 10, 811–822. doi: 10.1093/aob/mcp128
Chen, C. C., Dixon, J. B., and Turner, F. T. (1980a). Iron coatings on rice roots: morphology and models of development. Soil Sci. Soc. Am. J. 44, 1113–1119. doi: 10.2136/sssaj1980.03615995004400050046x
Chen, C. C., Dixon, J. B., and Turner, F. T. (1980b). Iron coatings on rice roots: mineralogy and quantity influencing factors. Soil Sci. Soc. Am. J. 44, 635–639. doi: 10.2136/sssaj1980.03615995004400030041x
Chen, W. W., Yang, J. L., Qin, C., Jin, C. W., Mo, J. H., Ye, T., et al. (2010). Nitric oxide acts downstream of auxin to trigger root ferric-chelate reductase activity in response to iron deficiency in Arabidopsis. Pant Physiol. 154, 810–819. doi: 10.1104/pp110.161109
Cheng, N. H., Liu, J. Z., Liu, X., Wu, Q., Thompson, S. M., Lin, J., et al. (2011). Arabidopsis monothiol glutaredoxin, AtGRXS17, is critical for temperature-dependent postembryonic growth and development via modulating auxin response. J. Biol. Chem. 286, 20398–20406. doi: 10.1074/jbc.M110.201707
Colangelo, E. P., and Guerinot, M. L. (2004). The essential basic helix-loop-helix protein FIT1 is required for the iron deficiency response. Plant Cell 12, 3400–3412. doi: 10.1105/tpc.104.024315
Connolly, E. L., and Guerinot, M. L. (2002). Iron stress in plants. Genome. Biol. 3, 1–6. doi: 10.1186/gb-2002-3-8-reviews1024
Dat, J. F., Capelli, N., Folzer, H., Bourgeade, P., and Badot, P. M. (2004). Sensing and signalling during plant flooding. Plant Physiol. Biochem. 42, 273–282. doi: 10.1016/j.plaphy.2004.02.003
De Smet, S., Cuypers, A., Vangronsveld, J., and Remans, T. (2015). Gene networks involved in hormonal control of root development in Arabidopsis thaliana: a framework for studying its disturbance by metal stress. Int. J. Mol. Sci. 16, 19195–19224. doi: 10.3390/ijms160819195
Eide, D., Broderius, M., Fett, J., and Guerinot, M. L. (1996). A novel iron-regulated metal transporter from plants identified by functional expression in yeast. Proc. Natl. Acad. Sci. U.S.A. 93, 5624–5628.
Eroglu, S., Meier, B., von Wirén, N., and Peiter, E. (2016). The vacuolar manganese transporter MTP8 determines tolerance to iron deficiency-induced chlorosis in Arabidopsis. Plant Physiol. 170, 1030–1045. doi: 10.1104/pp.15.01194
Fageria, N. K., Santos, A. B., Barbosa Filho, M. P., and Guimarães, C. M. (2008). Iron toxicity in lowland rice. J. Plant Nutr. 31, 1676–1697. doi: 10.1080/01904160802244902
Forde, B., and Lorenzo, H. (2001). The nutritional control of root development. Plant Soil 232, 51–68. doi: 10.1023/A:1010329902165
Freschi, L. (2013). Nitric oxide and phytohormone interactions: current status and perspectives. Front. Plant Sci. 4:398. doi: 10.3389/fpls.2013.00398
Giehl, R. F. H., Gruber, B. D., and von Wirén, N. (2014). It’s time to make changes: modulation of root system architecture by nutrient signals. J. Exp. Bot. 65, 769–778. doi: 10.1093/jxb/ert421
Giehl, R. F. H., Lima, J. E., and von Wirén, N. (2012a). Localized iron supply triggers lateral root elongation in Arabidopsis by altering the AUX1-mediated auxin distribution. Plant Cell 24, 33–49. doi: 10.1105/tpc.111.092973
Giehl, R. F. H., Lima, J. E., and von Wirén, N. (2012b). Regulatory components involved in altering lateral root development in response to localized iron: evidence for natural genetic variation. Plant Signal. Behav. 7, 711–713. doi: 10.4161/psb.20337
Gruber, B. D., Giehl, R. F. H., Friedel, S., and von Wirén, N. (2013). Plasticity of the Arabidopsis root system under nutrient deficiencies. Plant Physiol. 163, 161–179. doi: 10.1104/pp.113.218453
Harahap, S. M., Ghulamahdi, M., Aziz, S. A., Sutandi, A., and Miftahudin. (2014). Relationship of ethylene production and aerenchyme formation on oxidation ability and root surfaced-iron (Fe2+) accumulation under different iron concentrations and rice genotypes. Int. J. Appl. Mat. Com. 4, 186–194.
Haruta, M., and Sussman, M. R. (2012). The effect of a genetically reduced plasma membrane protonmotive force on vegetative growth of Arabidopsis. Plant Physiol. 158, 1158–1171. doi: 10.1104/pp.111.189167
Heil, M., and Baldwin, I. T. (2002). Fitness costs of induced resistance: emerging experimental support for a slippery concept. Trends Plant Sci. 7, 61–67. doi: 10.1016/S1360-1385(01)02186-0
Hermans, C., Hammond, J. P., White, P. J., and Verbruggen, N. (2006). How do plants respond to nutrient shortage by biomass allocation? Trends Plant Sci. 11, 610–617. doi: 10.1016/j.tplants.2006.10.007
Imsande, J. (1998). Iron, sulfur, and chlorophyll deficiencies: a need for an integrative approach in plant physiology. Physiol. Plant 103, 139–144. doi: 10.1034/j.1399-3054.1998.1030117.x
Jeong, J., and Guerinot, M. L. (2009). Homing in on iron homeostasis in plants. Trends Plant Sci. 14, 280–285. doi: 10.1016/j.tplants.2009.02.006
Jin, C. W., Li, G. X., Yu, X. H., and Zheng, S. J. (2010). Plant Fe status affects the composition of siderophore-secreting microorganisms in the rhizosphere. Ann. Bot. 105, 835–841. doi: 10.1093/aob/mcq071
Jin, C. W., Ye, E. Q., and Zheng, S. J. (2014). An underground tale: contribution of microbial activity to plant iron acquisition via ecological processes. Ann. Bot. 113, 7–18. doi: 10.1093/aob/mct249
Jones, D. L., and Kochian, L. V. (1995). Aluminum inhibition of the 1,4,5-trisphosphate signal transduction pathway in wheat roots: a role in aluminum toxicity? Plant Cell 7, 1913–1922. doi: 10.1105/tpc.7.11.1913
Kobae, Y., Tomioka, R., Tanoi, K., Kobayashi, N. I., Ohmori, Y., Nishida, S., et al. (2014). Selective induction of putative iron transporters, OPT8a and OPT8b, in maize by mycorrhizal colonization. Soil Sci. Plant Nutr. 60, 843–847. doi: 10.1080/00380768.2014.949854
Kobayashi, T., and Nishizawa, N. K. (2012). Iron uptake, translocation, and regulation in higher plants. Annu. Rev. Plant Biol. 63, 131–152. doi: 10.1146/annurev-arplant-042811-105522
Kochian, L. V., Hoekenga, O. A., and Pineros, M. A. (2004). How do crop plants tolerate acid soils? Mechanisms of aluminum tolerance and phosphorous efficiency. Annu. Rev. Plant Biol. 55, 459–493. doi: 10.1146/annurev.arplant.55.031903.141655
Kosegarten, H., Hoffmann, B., and Rroco, E. (2004). Apoplastic pH and Fe-III reduction in young sunflower (Helianthus annuus) roots. Physiol. Plant 122, 95–106. doi: 10.1111/j.1399-3054.2004.00377.x
Kronzucker, H. J., Siddiqi, M. Y., and Glass, A. D. M. (1995). Kinetics of NO3- influx in spruce. Plant Physiol. 109, 319–326. doi: 10.1104/pp109.1.319
Lai, F., Thacker, J., Li, Y. Y., and Doerner, P. (2007). Cell division activity determines the magnitude of phosphate starvation responses in Arabidopsis. Plant J. 50, 545–556. doi: 10.1111/j.1365-313X.2007.03070.x
Larsen, M., Santner, J., Oburger, E., Wenzel, W. W., and Glud, R. N. (2015). O2 dynamics in the rhizosphere of young rice plants (Oryza sativa L.) as studied by planar optodes. Plant Soil 390, 279–292. doi: 10.1007/s11104-015-2382-z
Li, G. J., Song, H., Li, B., Kronzucker, H. J., and Shi, W. M. (2015a). Auxin resistant1 and PIN-FORMED2 protect lateral root formation in Arabidopsis under iron stress. Plant Physiol. 169, 2608–2623. doi: 10.1104/pp.15.00904
Li, G. J., Xu, W., Kronzucker, H. J., and Shi, W. M. (2015b). Ethylene is critical to the maintenance of primary root growth and Fe homeostasis under Fe stress in Arabidopsis. J. Exp. Bot. 66, 2041–2054. doi: 10.1093/jxb/erv005
Li, X. N., Ma, H. Z., Jia, P. X., Wang, J., Jia, L. Y., Zhang, T. G., et al. (2012). Responses of seedling growth and antioxidant activity to excess iron and copper in Triticum aestivum L. Ecotox. Environ. Saf. 86, 47–53. doi: 10.1016/j.ecoenv.2012.09.010
López-Bucio, J., Cruz-Ramírez, A., and Herrera-Estrella, L. (2003). The role of nutrient availability in regulating root architecture. Curr. Opin. Plant Biol. 6, 280–287. doi: 10.1016/S1369-5266(03)00035-9
López-Millán, A. F., Morales, F., Andaluz, A., Gogorcena, Y., Abadía, A., and de las Rivas, J. (2000). Protective mechanisms in roots of iron deficient sugar beet: changes in carbon assimilation and oxygen use. Plant Physiol. 124, 885–897. doi: 10.1104/pp.124.2.885
Ma, J. F., Shinada, T., Matsuda, C., and Nomoto, K. (1995). Biosynthesis of phytosiderophores, mugineic acids, associated with methionine cycling. J. Biol. Chem. 270, 16549–16554. doi: 10.1074/jbc.270.28.16549
Majerus, V., Bertin, P., and Lutts, S. (2009). Abscisic acid and oxidative stress implications in overall ferritin synthesis by African rice (Oryza glaberrima Steud.) seedlings exposed to short term iron toxicity. Plant Soil 324, 253–265. doi: 10.1007/s11104-009-9952-x
Majerus, V., Bertin, P., and Lutts, S. (2007). Effects of iron toxicity on osmotic potential, osmolytes and polyamines concentration in the African rice (Oryza glaberrima Steud). Plant Sci. 173, 96–105. doi: 10.1016/j.plantsci.2007.04.003
Manzano, C., Pallero-Baena, M., Casimiro, I., De Rybel, B., OrmanLigeza, B., Van Isterdael, G., et al. (2014). The emerging role of ROS signaling during lateral root development. Plant Physiol. 165, 1105–1119. doi: 10.1104/pp.114.238873
Marschner, H., Romheld, V., and Kissel, M. (1986). Different strategies in higher plants in mobilization and uptake of iron. J. Plant Nutr. 9, 3–7. doi: 10.1080/01904168609363475
McLamore, E. S., Jaroch, D., Chatni, M. R., and Porterfield, D. M. (2010). Self-referencing optrodes for measuring spatially resolved, real-time metabolic oxygen flux in plant systems. Planta 232, 1087–1099. doi: 10.1007/s00425-010-1234-6
McLaughlin, B. E., Loon, G. W., and Crowder, A. A. (1985). Comparison of selected washing treatments on Agrostis giganteansamples from mine tailings near Copper Cliff, Ontario, before analysis for Cu, Ni, Fe and K content. Plant Soil 85, 433–436.
Mongon, J., Konnerup, D., Colmer, T. D., and Rerkasem, B. (2014). Responses of rice to Fe2+ in aerated and stagnant conditions: growth, root porosity and radial oxygen loss barrier. Funct. Plant Biol. 41, 922–929. doi: 10.1071/FP13359
Mugnai, S., Azzarello, E., Baluška, F., and Mancuso, S. (2012). Local root apex hypoxia induces NO-mediated hypoxic acclimation of the entire root. Plant Cell Physiol. 53, 912–920. doi: 10.1093/pcp/pcs034
Mugnai, S., Pandolfi, C., Masi, E., Azzarello, E., Monetti, E., Comparini, D., et al. (2014). Oxidative stress and NO signalling in the root apex as an early response to changes in gravity conditions. Biomed Res. Int. 2014, 1–10. doi: 10.1155/2014/834134
Müller, J., Toev, T., Heisters, M., Teller, J., Moore, K. L., Hause, G., et al. (2015). Iron-dependent callose deposition adjusts root meristem maintenance to phosphate availability. Dev. Cell 33, 216–230. doi: 10.1016/j.devcel.2015.02.007
Osmont, K. S., Sibout, R., and Hardtke, C. S. (2007). Hidden branches: developments in root system architecture. Annu. Rev. Plant Biol. 58, 93–113. doi: 10.1146/annurev.arplant.58.032806.104006
Potters, G., Pasternak, T. P., Guisez, Y., and Jansen, M. A. K. (2009). Different stresses, similar morphogenic responses: integrating a plethora of pathways. Plant Cell Environ. 32, 158–169. doi: 10.1111/j.1365-3040.2008.01908.x
Ratering, S., and Schnell, S. (2000). Localization of iron-reducing activity in paddy soil by profile studies. Biochem 48, 341–365.
Reyt, G., Boudouf, S., Boucherez, J., Gaymard, F., and Briat, J. F. (2015). Iron and ferritin dependent ROS distribution impact Arabidopsis root system architecture. Mol. Plant 8, 439–453. doi: 10.1016/j.molp.2014.11.014
Robinson, N. J., Procter, C. M., Connolly, E. L., and Guerinot, M. L. (1999). A ferric-chelate reductase for iron uptake from soils. Nature 397, 694–697. doi: 10.1038/17800
Romera, F. J., García, M., Alcántara, E., and Pérez-Vicente, R. (2011). Latest findings about the interplay of auxin, ethylene and nitric oxide in the regulation of Fe deficiency responses by Strategy I plants. Plant Signal. Behav. 6, 167–170. doi: 10.4161/psb.6.1.14111
Romheld, V., and Marschner, H. (1986). Evidence for a specific uptake system for iron phytosiderophores in roots of grasses. Plant Physiol. 80, 175–180. doi: 10.1104/pp.80.1.175
Rroco, E., Kosegarten, H., Harizaj, F., Imani, J., and Mengel, K. (2003). The importance of soil microbial activity for the supply of iron to sorghum and rape. Euro. J. Agron. 19, 487–493. doi: 10.1016/S1161-0301(02)00185-5
Ryan, P. R., DiTomaso, J. M., and Kochian, L. V. (1993). Aluminum toxicity in roots: an investigation of spatial sensitivity and the role of the root cap. J. Exp. Bot. 44, 437–446. doi: 10.1093/jxb/44.2.437
Santi, S., and Schmidt, W. (2009). Dissecting iron deficiency-induced proton extrusion in Arabidopsis roots. New Phytol. 183, 1072–1084. doi: 10.1111/j.1469-8137.2009.02908.x
Satbhai, S., and Busch, W. (2014). A set of LRR-RLK genes quantitatively regulate root growth under iron-limited conditions in Arabidopsis. FEBS J. 281, 316. doi: 10.1111/febs.12919
Schmidt, W. (2003). Iron solutions: acquisition strategies and signaling pathways in plants. Trends Plant Sci. 8, 188–193. doi: 10.1016/S1360-1385(03)00048-7
Schmidt, W. (2006). “Iron stress response in roots of strategy I plants,” in Iron Nutrition in Plants and Rhizospheric Microorganisms, eds L. L. Barton and J. Abadia (Amsterdam: Springer).
Schmidt, W., Tittel, J., and Schikora, A. (2000). Role of hormones in the induction of iron deficiency responses in Arabidopsis roots. Plant Physiol. 122, 1109–1118. doi: 10.1104/pp.122.4.1109
Seguela, M., Briat, J. F., Vert, G., and Curie, C. (2008). Cytokinins negatively regulate the root iron uptake machinery in Arabidopsis through a growth-dependent pathway. Plant J. 55, 289–300. doi: 10.1111/j.1365-313X.2008.03502.x
Shi, W. M., Chino, M., Youssef, R. A., Mori, S., and Takagi, S. (1988). The occurrence of mugineic acid in the rhizosphere soil of barley plant. Soil Sci. Plant Nutr. 34, 585–592. doi: 10.1080/00380768.1988.10416473
Shi, W. M., and Liu, Z. Y. (1991). Secretion of phytosiderophore and its effects on soil Fe availability. Pedosphere 1, 73–81.
Sivaguru, M., Liu, J., and Kochian, L. V. (2013). Targeted expression of SbMATE in the root distal transition zone is responsible for sorghum aluminum resistance. Plant J. 76, 297–307. doi: 10.1111/tpj.12290
Sivitz, A. B., Hermand, V., Curie, C., and Vert, G. (2012). Arabidopsis bHLH100 and bHLH101 control iron homeostasis via a FIT-independent pathway. PLoS ONE 7:e44843. doi: 10.1371/journal.pone.0044843
Staal, M., Cnodder, T. D., Simon, D., Vandenbussche, F., Straeten, D. V. D., Verbelen, J. P., et al. (2011). Apoplastic alkalinization is instrumental for the inhibition of cell elongation in the Arabidopsis root by the ethylene precursor 1-aminocyclopropane-1-carboxylic acid. Plant Physiol. 155, 2049–2055. doi: 10.1104/pp110.168476
Sun, F., Zhang, W., Hu, H., Li, B., Wang, Y., Zhao, Y., et al. (2008). Salt modulates gravity signaling pathway to regulate growth direction of primary roots in Arabidopsis. Plant Physiol. 146, 178–188. doi: 10.1104/pp.107.109413
Svistoonoff, S., Creff, A., Reymond, M., Sigoillot-Claude, C., Ricaud, L., Blanchet, A., et al. (2007). Root tip contact with low-phosphate media reprograms plant root architecture. Nature Gen. 39, 792–796. doi: 10.1038/ng2041
Tadano, T. (1975). Devices of rice roots to tolerated high iron concentration in growth media. Jpn. Agr. Res. Quart. 9, 34–39.
Takagi, S., Nomoto, K., and Takemoto, T. (1984). Physiological aspect of mugineic acid, a possible phytosiderophore of graminaceous plants. J. Plant Nutr. 7, 469–477. doi: 10.1080/01904168409363213
Trevisan, S., Manoli, A., Ravazzolo, L., Botton, A., Pivato, M., Masi, A., et al. (2015). Nitrate sensing by the maize root apex transition zone: a merged transcriptomic and proteomic survey. J. Exp. Bot. 66, 3699–3715. doi: 10.1093/jxb/erv165
Tsang, D. L., Edmond, C., Harrington, J. L., and Nuhse, T. S. (2011). Cell wall integrity controls root elongation via a general 1-aminocyclopropane-1-carboxylic acid-dependent, ethylene-independent pathway. Plant Physiol. 156, 596–604. doi: 10.1104/pp.111.175372
Tsukagoshi, H., Busch, W., and Benfey, P. N. (2010). Transcriptional regulation of ROS controls transition from proliferation to differentiation in the root. Cell 143, 606–616. doi: 10.1016/j.cell.2010.10.020
Walker, E. L., and Connolly, E. L. (2008). Time to pump iron: irondeficiency-signaling mechanisms of higher plants. Curr. Opin. Plant Biol. 11, 530–535. doi: 10.1016/j.pbi.2008.06.013
Wang, N., Cui, Y., Liu, Y., Fan, H., Du, J., Huang, Z., et al. (2013). Requirement and functional redundancy of Ib subgroup bHLH proteins for iron deficiency responses and uptake in Arabidopsis thaliana. Mol. Plant 6, 503–513. doi: 10.1093/mp/sss089
Ward, J. T., Lahner, B., Yakubova, E., Salt, D. E., and Raghothama, K. G. (2008). The effect of iron on the primary root elongation of Arabidopsis during phosphate deficiency. Plant Physiol. 147, 1181–1191. doi: 10.1104/pp.108.1185621110
Wisniewska, J., Xu, J., Seifertová, D., Brewer, P. B., Ruzicka, K., Blilou, I., et al. (2006). Polar PIN localization directs auxin flow in plants. Science 312:883. doi: 10.1126/science.1121356
Xiong, J., An, L., Lu, H., and Zhu, C. (2009). Exogenous nitric oxide enhances cadmium tolerance of rice by increasing pectin and hemicellulose contents in root cell wall. Planta 230, 755–765. doi: 10.1007/s00425-009-0984-5
Xu, W. F., Jia, L., Baluška, F., Ding, G., Shi, W., Ye, N., et al. (2012). PIN2 is required for the adaptation of Arabidopsis roots to alkaline stress by modulating proton secretion. J. Exp. Bot. 63, 6105–6114. doi: 10.1093/jxb/ers259
Xu, W. F., Jia, L. G., Shi, W. M., Liang, J., Zhou, F., Li, Q., et al. (2013). Abscisic acid accumulation modulates auxintransport in the root tip to enhance proton secretion for maintaining root growth under moderate water stress. New Phytol. 197, 139–150. doi: 10.1111/nph.12004
Yamauchi, M., and Peng, X. X. (1995). Iron toxicity and stress-induced ethylene production in rice leaves. Plant Soil 173, 21–28.
Yang, Y., Qin, Y., Xie, C., Zhao, F., Zhao, J., Liu, D., et al. (2010). The Arabidopsis chaper one J3 regulates the plasma membrane H+-ATPase through interaction with the PKS5 kinase. Plant Cell 22, 1313–1332. doi: 10.1105/tpc.109.069609
Yoshida, S. (1981). Fundamentals of Rice Crop Science. Manila: The International Rice Research Institute.
Yuan, H., Liu, W., Jin, Y., and Lu, Y. (2013). Role of ROS and auxin in plant response to metal mediated stress. Plant Signal. Behav. 8:e24671. doi: 10.4161/psb.24671
Zhang, F. S., Romheld, V., and Marschner, H. (1989). Effect of zinc deficiency in wheat on the release of zinc and iron mobilizing root exudates. J. Plant Nutr. Soil Sci. 152, 205–210. doi: 10.1002/jpln.19891520211
Zhang, H., and Forde, B. G. (1998). An Arabidopsis MADS box gene that controls nutrient-induced changes in root architecture. Science 279, 407–409. doi: 10.1126/science.279.5349.407
Zhang, Y., Wang, Y. P., Liu, P., Song, J. M., Xu, G. D., and Zheng, G. H. (2012). Effect of toxic Fe2+ level on the biological characteristics of rice root border cell. Russ. J. Plant Physiol. 59, 766–771. doi: 10.1134/S1021443712060209
Zhang, Y., Zheng, G. H., Liu, P., Song, J. M., Xu, G. D., and Cai, M. Z. (2011). Morphological and physiological responses of root tip cells to Fe2+ toxicity in rice. Acta Physiol. Plant. 33, 683–689. doi: 10.1007/s11738-010-0590-y
Zhu, X., Wang, Z., Dong, F., Lei, G., Shi, Y., Li, G., et al. (2013). Exogenous auxin alleviates cadmium toxicity in Arabidopsis thaliana by stimulating synthesis of hemicellulose 1 and increasing the cadmium fixation capacity of root cell walls. J. Hazard Mater. 263, 398–403. doi: 10.1016/j.jhazmat.2013.09.018
Zolla, G., Heimer, Y. M., and Barak, S. (2010). Mild salinity stimulates a stress-induced morphogenic response in Arabidopsis thaliana roots. J. Exp. Bot. 61, 211–224. doi: 10.1093/jxb/erp290
Zou, N., Li, B. H., Chen, H., Su, Y. H., Kronzucker, H. J., Xiong, L. M., et al. (2013). GSA-1/ARG1 protects root gravitropism in Arabidopsis under ammonium stress. New Phytol. 200, 97–111. doi: 10.1111/nph.12365
Keywords: iron, root apex, morphology, physiology, plant
Citation: Li G, Kronzucker HJ and Shi W (2016) The Response of the Root Apex in Plant Adaptation to Iron Heterogeneity in Soil. Front. Plant Sci. 7:344. doi: 10.3389/fpls.2016.00344
Received: 11 December 2015; Accepted: 07 March 2016;
Published: 21 March 2016.
Edited by:
Frantisek Baluska, University of Bonn, GermanyReviewed by:
Autar Krishen Mattoo, United States Department of Agriculture, USASilvia Quaggiotti, Università degli Studi di Padova, Italy
Copyright © 2016 Li, Kronzucker and Shi. This is an open-access article distributed under the terms of the Creative Commons Attribution License (CC BY). The use, distribution or reproduction in other forums is permitted, provided the original author(s) or licensor are credited and that the original publication in this journal is cited, in accordance with accepted academic practice. No use, distribution or reproduction is permitted which does not comply with these terms.
*Correspondence: Weiming Shi, d21zaGlAaXNzYXMuYWMuY24=