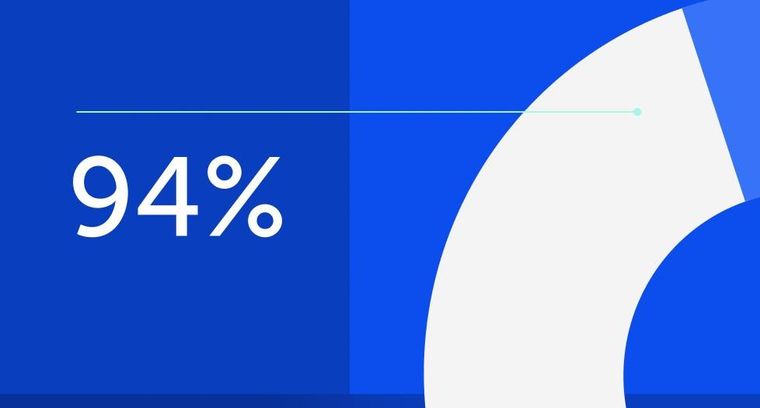
94% of researchers rate our articles as excellent or good
Learn more about the work of our research integrity team to safeguard the quality of each article we publish.
Find out more
MINI REVIEW article
Front. Plant Sci., 29 February 2016
Sec. Plant Physiology
Volume 7 - 2016 | https://doi.org/10.3389/fpls.2016.00218
This article is part of the Research TopicIon Transport in Chloroplast and Mitochondria Physiology in Green OrganismsView all 11 articles
Proton gradients are fundamental to chloroplast function. Across thylakoid membranes, the light induced -proton gradient is essential for ATP synthesis. As a result of proton pumping into the thylakoid lumen, an alkaline stromal pH develops, which is required for full activation of pH-dependent Calvin Benson cycle enzymes. This implies that a pH gradient between the cytosol (pH 7) and the stroma (pH 8) is established upon illumination. To maintain this pH gradient chloroplasts actively extrude protons. More than 30 years ago it was already established that these proton fluxes are electrically counterbalanced by Mg2+, K+, or Cl- fluxes, but only recently the first transport systems that regulate the pH gradient were identified. Notably several (Na+,K+)/H+ antiporter systems where identified, that play a role in pH gradient regulation, ion homeostasis, osmoregulation, or coupling of secondary active transport. The established pH gradients are important to drive uptake of essential ions and solutes, but not many transporters involved have been identified to date. In this mini review we summarize the current status in the field and the open questions that need to be addressed in order to understand how pH gradients are maintained, how this is interconnected with other transport processes and what this means for chloroplast function.
Proton (H+) gradients across biomembranes represent strong driving forces vital for cellular and cell organellar function. In plant cells, several compartments with different pH exist in parallel. While the apoplast and the vacuole maintain fairly acidic pH levels generally between pH 5 and 7 (Grignon and Sentenac, 1991; Martiniere et al., 2013; Shen et al., 2013). The cytosol has to stay neutral (pH 7.2-7.4) to ensure proper biochemical reactions (Schumacher, 2014). Only mitochondria, the chloroplast stroma and some peroxisomes offer alkaline reaction conditions with pH values equal to or higher than 8 (Shen et al., 2013). The pH gradients have to be actively maintained. In the chloroplast thylakoid membrane or in mitochondria this is achieved by H+-transporting electron transfer chains while other organelles depend on ATP fueled H+-pumps (ATPases). Although H+-pumping activities have been detected in several organellar membranes, the molecular identity of all proteins involved is not known. The established pH-gradients are critical to drive secondary active transport by for instance organellar ion/H+ exchange. In recent decades, most research has focused on H+-gradient dependent transporters in endomembrane organelles, but knowledge on mitochondria and chloroplasts lags behind (Bassil et al., 2012; Pittman, 2012). Given the central role of mitochondria and chloroplasts for plant energy metabolism, these systems are urgently awaiting more targeted research activity.
This review focuses on the chloroplast, which harbors the pathway of arguably the most important biochemical process for life on earth, photosynthesis. Eukaryotic photosynthesis depends on the H+ and ion-gradients established between the sub-organellar chloroplast compartments, the thylakoid lumen and the stroma. We will discuss current knowledge on H+-gradients and H+-coupled transport across the chloroplast membranes, and how pH-homeostasis in chloroplast compartments is achieved and maintained.
Light-induced electron transport across the thylakoid membrane gives rise to H+-pumping into the thylakoid lumen. The resulting H+-electrochemical potential difference (pmf) is used for ATP synthesis by the thylakoid ATP synthase. It is generally assumed that both components of the pmf, that is the pH gradient (ΔpH) and the electric field (ΔΨ), generated by the electron transport chain are equally capable of driving ATP synthesis (Kramer et al., 2003). Although the luminal pH was suggested to drop below pH 5 in some circumstances, recent studies show more modest values of 5.7–7.8 while the stromal pH rises to about 7.8–8.0 (Takizawa et al., 2007). Early reports indicated that the H+-accumulation is electrically compensated by K+ or Mg2+ efflux, or Cl- influx (Enz et al., 1993), but only recently, first transporter genes involved in pH- and osmoregulation in the thylakoid were discovered.
First, the two-pore potassium channel TPK3 was confirmed by immunodetection to localize to the stroma lamellae (Carraretto et al., 2013; Figure 1). TPK3-RNAi-silenced Arabidopsis plants revealed stunted growth phenotypes along with anthocyanin accumulation under ambient light conditions (90 μE). TPK3 mutants are compromised in generating ΔpH while increasing ΔΨ by 20% compared to wild-type controls (Carraretto et al., 2013). The channel is in vitro activated by Ca2+ and acidification and releases K+ from the thylakoid membrane to regulate the pmf (Carraretto et al., 2013). Additionally, light-induced Mg2+ release into the stroma (Krause, 1977) and luminal Cl- uptake (Enz et al., 1993) was demonstrated. However, the identity of the Mg2+ transporter at the thylakoid membrane remains hypothetical (Figure 1). T-DNA mutants of the thylakoid membrane channel AtCLCe reveal only subtle effects on photosynthesis (Marmagne et al., 2007), and a role in charge equilibration or Cl- import into the lumen still remains hypothetical as indicated in Figure 1. Thus, K+ appears to be the fundamental coupling ion.
FIGURE 1. Chloroplast with its electron transport chain and selected transporters in the thylakoid membrane. Transport directions are shown for chloroplasts in the light. The K+/H+ antiporter KEA3 and the Potassium Channel TPK3 are confirmed thylakoid membrane K+ transporters. The molecular identity of Ca2+ and Mg2+ transporters has not been determined and the localization and transport activity of the CLCe channel is uncertain. Both is indicated by question marks. The black dashed line indicates linear electron transport in the thylakoid membrane. Ratios and dimensions of the protein complexes involved in the electron transport chain are schematic and do not reflect physiological conditions.
Recently, an electro-neutral K+ efflux antiporter (KEA3) from the Cation Proton Antiporter (CPA2) family was identified in the thylakoid membrane, predominantly in stroma lamellae (Figure 1), of Arabidopsis (Armbruster et al., 2014; Kunz et al., 2014). Independent kea3 T-DNA insertion mutants reveal an increased ΔpH component of pmf partitioning with a 20% diminished ΔΨ, suggesting a role in pmf regulation via K+/H+ exchange across the thylakoid membrane (Kunz et al., 2014). An increase in conductivity to counter ions, for instance by opening of the TPK3 channel, will promote the development of the ΔpH component, but short-circuit the membrane potential, while the activity of KEA3 would only dissipate the ΔpH component, leaving the ΔΨ intact. Armbruster et al. (2014) showed a crucial role for KEA3 in altering the dynamics of the non-photochemical quenching (NPQ) component qE under fluctuating light conditions. qE dissipates excessive excitation energy into heat and is triggered by low lumen pH (Muller et al., 2001). KEA3 was shown to increase photosystem II (PSII) quantum yield [φ(II)] during the transition from high light to low light by accelerating the downregulation of qE via H+-efflux from the lumen (Armbruster et al., 2014). At the same time, reduced luminal acidification in plants with impaired TPK3 function causes increased sensitivity to ambient light intensities, as the mechanisms to dissipate excess light energy cannot be activated (Carraretto et al., 2013). A similar observation was made for cyanobacterial TPK3 counterpart SynK (Checchetto et al., 2012). In a SynK-deficient mutant, the decreased ΔpH component also leads to the inability to trigger NPQ. Thus, it appears that the coordinated activation and deactivation of TPK3 and KEA3 enables the interconversion of ΔΨ and ΔpH in response to environmental stimuli, in order to optimize photosynthesis (Kramer et al., 2003; Armbruster et al., 2014).
Genes with high sequence similarity to KEA3 exist in green algae, moss and higher plants, with one gene copy appearing in both Chlamydomonas and rice, and two gene copies in Physcomitrella (Chanroj et al., 2012). The cyanobacterium Synechocystis sp. expresses a CPA2 gene NhaS3, with some sequence similarity to CHX members and KEA3 from Arabidopsis (Chanroj et al., 2012). NhaS3, as a potential Na+ (K+)/H+ antiporter was shown to be located in the thylakoid membrane and to enhance K+ uptake when expressed in Escherichia coli. The authors therefore concluded that NhaS3 is involved in thylakoid lumen ion-homeostasis (Tsunekawa et al., 2009).
It was reported that the thylakoid ΔpH also energizes Ca2+ import across the thylakoid membrane (Ettinger et al., 1999). Ettinger et al. (1999) suggested that Ca2+ import is driven by a hypothetical Ca2+/H+ antiporter (Figure 1), the gene of which remains elusive.
In summary, the ΔpH component of the pmf contributes to the ATP production, import of proteins, and the uptake of essential ions such as Ca2+. Further, the partitioning of pmf in ΔpH and ΔΨ is regulated via transport activities of KEA3 and TPK3, which has fundamental impact on photosynthetic efficiency and other pH-dependent processes. Finally, thylakoid H+-transport establishes the physiological pH in lumen and stroma, thereby ensuring the activity of the light-dependent photosynthetic reactions and the Calvin Benson cycle enzymes.
In the dark the cytoplasmic and stromal pH are close to 7, but upon illumination the stroma becomes alkaline, as a consequence of H+-pumping across the thylakoid membrane. An alkaline stroma pH is a prerequisite for the full activation of pH-dependent Calvin Benson cycle enzymes (Heldt et al., 1973; Werdan et al., 1975). This implies that a pH gradient between the cytosol (pH 7) and the stroma (pH 8) is established upon illumination. It is assumed, that in order to maintain this pH gradient, an active H+-export mechanism exists in the envelope membrane to compensate for passive H+-diffusion from cytosol to stroma. Evidence for an active H+-extrusion is based on the observation that illumination of isolated chloroplasts induces a small transitory acidification in slightly buffered solutions (Heber and Krause, 1971; Heldt et al., 1973; Heber and Heldt, 1981; Demmig and Gimmler, 1983). Experiments on equilibration of lipophilic cations and tracer studies in isolated chloroplasts indicate that a membrane potential of around -100 mV (negative inside, Figure 2) exists across the envelope membrane due to a Gibbs Donan equilibrium, in low salt solutions. At higher KCl concentrations, similar to the situation inside the cell, this membrane potential becomes very small (Demmig and Gimmler, 1983). In illuminated chloroplasts the membrane potential increases by about 10 mV, concomitant with light induced H+-extrusion and coupled K+ uptake (Demmig and Gimmler, 1983; Wu et al., 1991). The K+/H+ exchange is not abolished by the K+ ionophore valinomycin, indicating that K+ and H+-fluxes are electrically and thus indirectly coupled (Demmig and Gimmler, 1983; Wu and Berkowitz, 1992; Wang et al., 1993). Several mechanisms have been proposed to explain the H+-efflux, but the molecular identities of the transporters involved are still elusive.
FIGURE 2. Selected transporters in the chloroplast envelope membrane. Transporter activities are shown for chloroplasts in the light. The nature of the H+ efflux mechanism and the molecular identity of K+ channels is still unknown and the Cl- substrate specificity of the MSL2/3 channels is not confirmed. Both is indicated by question marks.
H+-extrusion was suggested to depend on a K+-stimulated vanadate sensitive envelope P-type H+-ATPase (Scherer et al., 1986; Berkowitz and Peters, 1993; Mi et al., 1994; Shingles and McCarty, 1994) but the Arabidopsis genome does not encode for chloroplast predicted P-type H+-pumps (Axelsen and Palmgren, 2001). Other reports found that the envelope ATPase activity is sensitive to oligomycin, which is not a P-type ATPase inhibitor but a Fo subunit inhibitor of the F1Fo ATP Synthase. However, no ATPases were identified in chloroplast proteomics studies of envelope membranes. In conclusion the existence of an envelope H+-ATPase is hypothetical. Alternatively, an electron-transfer chain may be responsible for H+-extrusion. Although proteins that could fulfill such functions are found in the envelope membrane, their function in H+-extrusion has never been demonstrated (Jäger-Vottero et al., 1997; Murata and Takahashi, 1999; Kovacs-Bogdan et al., 2010). In algae, the plastome-encoded, putative heme-binding, inner envelope protein YCF10 from Chlamydomonas is essential for enhancing carbon fixation and inorganic carbon uptake (Rolland et al., 1997). The YCF10 protein was suggested to be involved in plastid pH regulation and to be part of a redox chain in the envelope membrane (Jäger-Vottero et al., 1997; Rolland et al., 1997). In ycf10 deficient mutants of Chlamydomonas the CO2 or HCO3- uptake was compromised and cells failed to grow photoautotrophically (Rolland et al., 1997). It was hypothesized that YCF10 is crucial for acidification of the intermembrane space by H+-translocation to allow for the conversion of HCO3- to CO2, which in turn could diffuse into the chloroplast (Rolland et al., 1997). A similar observation was made for the cyanobacterial homolog PcxA (CotA) that was shown to be involved in light-induced Na+-dependent H+-extrusion. The cotA insertion mutant MA29 of the cyanobacterium Synechocystis sp. shows no H+-export leading to a diminished CO2 uptake (Katoh et al., 1996).
Lastly, it was speculated that an alkaline stromal pH could be maintained by direct H+-export from the thylakoid lumen to the cytosol by some unknown temporary or spatial connection (Heber and Heldt, 1981). In summary, the mechanism by which H+ move across the envelope membrane and if this involves an ATP-dependent H+-pump or electron transport coupled H+-export into the intermembrane space or some other mechanism remains unknown (Figure 2).
Although the mechanism by which the envelope pH-gradient is generated and maintained during the day remains unclear, it represents the driving force for secondary active H+-coupled transport processes across the inner envelope membrane. Thus, it is worthwhile to focus at the characterized and putative H+-dependent transport mechanisms and their transport direction across the inner envelope membrane.
Electrogenic coupling of K+ uptake to H+-extrusion in chloroplasts has been demonstrated many times, and the presence of a K+ channel in the envelope membrane was hypothesized (Figure 2) based on biochemical studies with membrane vesicles (Wang et al., 1993) as well as patch clamp studies (Heibert et al., 1995). The chloroplast inner envelope is also reported to contain non-selective cation channel activities (Pottosin et al., 2005). However, up to today the molecular identity of these channels is unknown. Instead several, most likely electroneutral, K+/H+ or Na+/H+ antiporters were identified. The envelope pH-gradient dictates antiport activity via such antiporters toward K+-efflux, which can be an important feature for plastid osmoregulation (Shabala and Pottosin, 2010). Light induced shrinkage of chloroplasts was observed more than 40 years ago (Nobel et al., 1969). K+/H+ antiporters could be involved in this response, together with mechanosensitive channels MSL2 and MSL3 for which Cl- efflux activity was hypothesized (Veley et al., 2012; Figure 2).
Two members of the K+/H+ antiporter family KEA, KEA1 and KEA2 were confirmed to reside in the envelope membrane of Arabidopsis (Kunz et al., 2014). A short version of the KEA2 protein comprising the antiport domain and the regulatory C-terminal KTN domain, but not the long N-terminal domain of unknown function (Chanroj et al., 2012) was purified and reconstituted in liposomes containing the pH indicator pyranine to demonstrate ΔpH-dependent K+/H+ antiport (Aranda-Sicilia et al., 2012). KEA1 and KEA2 represent very close homologs, most likely due to a gene-duplication event and thus are expected to facilitate the same transport function. Moreover, single T-DNA insertion kea1 or kea2 mutants do not show visible phenotypes indicating functional redundancy in Arabidopsis (Kunz et al., 2014). kea1kea2 double mutant plants revealed stunted growth, pale green leaves and low photosynthetic efficiency (Kunz et al., 2014). Chloroplasts from double mutant plants have a swollen appearance, suggesting they suffer from excessive K+ accumulation. Interestingly, this phenotype depends on the leaf developmental stage, i.e., young leaves were found to be most strongly compromised (Kunz et al., 2014). Additionally, since the pmf across the thylakoid membrane depends not only on light but also on the stromal pH (Hauser et al., 1995), the lack of K+/H+-exchange across the envelope membrane in kea1kea2 mutants probably causes downstream effects that trigger changes in pmf partitioning with lower ΔpH and in turn lower qE (Kunz et al., 2014). This further emphasizes the linkage between plastid ion and pH homeostasis, and their importance for efficient photosynthesis.
The rice chloroplast envelope KEA homolog is encoded by a single locus named AM1, (albino midrib mutant1). The am1 mutant was isolated in an EMS screen and displays green- and white leaf variegations (Sheng et al., 2014). Surprisingly, no photosynthetic alterations were found in am1 mutants. However, mutants did reveal altered chloroplast ultrastructure along with increased drought tolerance (Sheng et al., 2014). The authors attribute AM1 function in rice to chloroplast development and drought stress (Sheng et al., 2014). Interestingly, the growth and photosynthesis defects in Arabidopsis kea1kea2 double mutant plants could be rescued partially in high NaCl concentrations. Salt or drought stress are known to increase cytoplasmic and vacuolar osmotic values due to accumulation of salt or synthesis of osmolytes. Possibly, this counteracts the increased osmotic values of the chloroplast stroma in absence of these KEA antiporters (Kunz et al., 2014).
In addition to the KEA K+/H+ antiporters, another cation exchanger was identified in the plastid envelope membrane of Arabidopsis, the Na+/H+ exchanger NHD1 (Furumoto et al., 2011; Muller et al., 2014; Figure 2). NHD1 is an NHAD type carrier that is also found in algae, mosses and other plant species (Barrero-Gil et al., 2007; Cosentino et al., 2010). AtNHD1 and homologs from Mesembryanthemum crystallinum and Physcomitrella were shown to be localized in the envelope membrane (Barrero-Gil et al., 2007; Cosentino et al., 2010; Muller et al., 2014). Investigation of the Arabidopsis T-DNA insertion mutant nhd1-1 showed compromised φ(II) and higher NPQ in response to a NaCl shock treatment (Muller et al., 2014). Proteomics studies on isolated Arabidopsis thylakoid membrane fractions also detected NHD1 in the stroma lamellae (Tomizioli et al., 2014). Therefore, a dual localization of the protein within the chloroplast cannot be excluded at the moment.
H+-gradient driven chloroplast Na+-efflux by NHD1 could prevent Na+-accumulation in the organelle during salt stress. Alternatively, this activity could be coupled to the action of Na+-dependent transporters in the envelope membrane. As such, AtNHD1 was suggested to operate as a two-translocator system together with the sodium:pyruvate cotransporter BASS2 (Furumoto et al., 2011; Figure 2). Several more Na+-dependent carriers in the envelope membrane have been described that facilitate ascorbate import (PHT4;4; Miyaji et al., 2015) or 2-keto acids transport (BASS5; Gigolashvili et al., 2009; Sawada et al., 2009; Figure 2). For PHT4;4 and BASS2 experimental evidence from in vitro transport studies have revealed the Na+-dependency. Furthermore, transporters of the PHT2 and PHT4 family, mediate H+ and/or Na+-dependent transport of phosphate into chloroplasts and are localized in the envelope membrane (Ferro et al., 2002; Versaw and Harrison, 2002; Roth et al., 2004; Guo et al., 2008).
In diatoms that evolved by secondary endosymbiosis, plastids possess four envelope membranes. To supply the plastid gene expression machinery with nucleotides and provide energy-rich components during the absence of photosynthesis, diatoms utilize nucleotide carriers that efficiently shuttle the compounds from the cytosol, where they are synthesized, into the stroma across the four membranes. Unlike the plastid nucleotide transporters (NTTs) from plant and algae, NTT1 from the diatoms Thalassiosira pseudonana and Phaeodactylum tricornutum is suggested to enter the innermost plastid envelope and function as H+-dependent symporter, which implies a vital function for an envelope pH-gradient in the diatoms (Ast et al., 2009).
Biochemical studies on intact isolated chloroplasts have suggested further H+-dependent transport processes across the envelope membrane. Measurements on inner envelope membrane vesicles revealed that Ca2+-uptake was stimulated by an electrochemical H+-gradient across the membrane. Further, Ca2+-movement across the membrane could be shown in the presence of a K+-diffusion potential gradient (Roh et al., 1998). Two potential candidates were suggested as membrane potential driven Ca2+-transporters in the chloroplast envelope. However, for neither of them the Ca2+ transport activity or the substrate specificity could be proven and thus the genetic loci encoding for an H+/Ca2+ uptake mechanism remains unknown. In summary, there is little doubt that Ca2+ is crucial for photosynthesis per se and therefore fine-tuned delivery systems into stroma and thylakoid lumen have to exist, reviewed in (Hochmal et al., 2015). Because of the significance of Ca2+ transporters for chloroplast function this issue needs more detailed research in the future.
It is of note that further transporters functionally important for chloroplast ion homeostasis exist in the envelope membrane. However, since these transporters are not driven by ΔpH, they are not listed here, but are reviewed comprehensively in a recent article (Finazzi et al., 2015).
All authors listed, have made substantial, direct and intellectual contribution to the work, and approved it for publication.
Funded by ERDF-co-financed grants from the Ministry of Economy and Competitiveness (BIO2012-33655) and Junta de Andalucía (CVI-7558) to KV. HHK and RH were funded by an NSF Career Grant IOS- 1553506, the School of Biological Sciences and the College of Arts and Sciences at Washington State University. AA acknowledges the receipt of a grant for his PhD studies from the Agricultural Research for Development Fund (ARDF, Egypt).
The authors declare that the research was conducted in the absence of any commercial or financial relationships that could be construed as a potential conflict of interest.
Aranda-Sicilia, M. N., Cagnac, O., Chanroj, S., Sze, H., Rodriguez-Rosales, M. P., and Venema, K. (2012). Arabidopsis KEA2, a homolog of bacterial KefC, encodes a K(+)/H(+) antiporter with a chloroplast transit peptide. Biochim. Biophys. Acta 1818, 2362–2371. doi: 10.1016/j.bbamem.2012.04.011
Armbruster, U., Carrillo, L. R., Venema, K., Pavlovic, L., Schmidtmann, E., Kornfeld, A., et al. (2014). Ion antiport accelerates photosynthetic acclimation in fluctuating light environments. Nat. Commun. 5, 5439. doi: 10.1038/ncomms6439
Ast, M., Gruber, A., Schmitz-Esser, S., Neuhaus, H. E., Kroth, P. G., Horn, M., et al. (2009). Diatom plastids depend on nucleotide import from the cytosol. Proc. Natl. Acad. Sci. U.S.A. 106, 3621–3626. doi: 10.1073/pnas.0808862106
Axelsen, K. B., and Palmgren, M. G. (2001). Inventory of the superfamily of P-type ion pumps in Arabidopsis. Plant Physiol. 126, 696–706. doi: 10.1104/pp.126.2.696
Barrero-Gil, J., Rodríguez-Navarro, A., and Benito, B. (2007). Cloning of the PpNHAD1 transporter of Physcomitrella patens, a chloroplast transporter highly conserved in photosynthetic eukaryotic organisms. J. Exp. Bot. 58, 2839–2849. doi: 10.1093/jxb/erm094
Bassil, E., Coku, A., and Blumwald, E. (2012). Cellular ion homeostasis: emerging roles of intracellular NHX Na+/H+ antiporters in plant growth and development. J. Exp. Bot. 63, 5727–5740. doi: 10.1093/jxb/ers250
Berkowitz, G. A., and Peters, J. S. (1993). Chloroplast inner-envelope ATPase acts as a primary H+ pump. Plant Physiol. 102, 261–267. doi: 10.1104/pp.102.1.261
Carraretto, L., Formentin, E., Teardo, E., Checchetto, V., Tomizioli, M., Morosinotto, T., et al. (2013). A thylakoid-located two-pore K+ channel controls photosynthetic light utilization in plants. Science 342, 114–118. doi: 10.1126/science.1242113
Chanroj, S., Wang, G., Venema, K., Zhang, M. W., Delwiche, C. F., and Sze, H. (2012). Conserved and diversified gene families of monovalent cation/H+ antiporters from algae to flowering plants. Front. Plant Sci. 3:25. doi: 10.3389/fpls.2012.00025
Checchetto, V., Segalla, A., Allorent, G., La Rocca, N., Leanza, L., Giacometti, G. M., et al. (2012). Thylakoid potassium channel is required for efficient photosynthesis in cyanobacteria. Proc. Natl. Acad. Sci. U.S.A. 109, 11043–11048. doi: 10.1073/pnas.1205960109
Cosentino, C., Fischer-Schliebs, E., Bertl, A., Thiel, G., and Homann, U. (2010). Na(+)/H(+) antiporters are differentially regulated in response to NaCl stress in leaves and roots of Mesembryanthemum crystallinum. New Phytol. 186, 669–680. doi: 10.1111/j.1469-8137.2010.03208.x
Demmig, B., and Gimmler, H. (1983). Properties of the isolated intact chloroplast at cytoplasmic K(+) concentrations: I. Light-induced cation uptake into intact chloroplasts is driven by an electrical potential difference. Plant Physiol. 73, 169–174. doi: 10.1104/pp.73.1.169
Enz, C., Steinkamp, T., and Wagner, R. (1993). Ion channels in the thylakoid membrane (a patch-clamp study). Biochim. Biophys. Acta 1143, 67–76. doi: 10.1016/0005-2728(93)90217-4
Ettinger, W. F., Clear, A. M., Fanning, K. J., and Peck, M. L. (1999). Identification of a Ca2+/H+ antiport in the plant chloroplast thylakoid membrane. Plant Physiol. 119, 1379–1386. doi: 10.1104/pp.119.4.1379
Ferro, M., Salvi, D., Riviere-Rolland, H., Vermat, T., Seigneurin-Berny, D., Grunwald, D., et al. (2002). Integral membrane proteins of the chloroplast envelope: identification and subcellular localization of new transporters. Proc. Natl. Acad. Sci. U.S.A. 99, 11487–11492. doi: 10.1073/pnas.172390399
Finazzi, G., Petroutsos, D., Tomizioli, M., Flori, S., Sautron, E., Villanova, V., et al. (2015). Ions channels/transporters and chloroplast regulation. Cell Calcium 58, 86–97. doi: 10.1016/j.ceca.2014.10.002
Furumoto, T., Yamaguchi, T., Ohshima-Ichie, Y., Nakamura, M., Tsuchida-Iwata, Y., Shimamura, M., et al. (2011). A plastidial sodium-dependent pyruvate transporter. Nature 476, 472–475. doi: 10.1038/nature10250
Gigolashvili, T., Yatusevich, R., Rollwitz, I., Humphry, M., Gershenzon, J., and Flugge, U. I. (2009). The plastidic bile acid transporter 5 is required for the biosynthesis of methionine-derived glucosinolates in Arabidopsis thaliana. Plant Cell 21, 1813–1829. doi: 10.1105/tpc.109.066399
Grignon, C., and Sentenac, H. (1991). pH and ionic conditions in the apoplast. Annu. Rev. Plant Physiol. Plant Mol. Biol. 42, 103–128. doi: 10.1146/annurev.pp.42.060191.000535
Guo, B., Jin, Y., Wussler, C., Blancaflor, E. B., Motes, C. M., and Versaw, W. K. (2008). Functional analysis of the Arabidopsis PHT4 family of intracellular phosphate transporters. New Phytol. 177, 889–898. doi: 10.1111/j.1469-8137.2007.02331.x
Hauser, M., Eichelmann, H., Oja, V., Heber, U., and Laisk, A. (1995). Stimulation by light of rapid pH regulation in the chloroplast stroma in vivo as indicated by CO2 solubilization in leaves. Plant Physiol. 108, 1059–1066.
Heber, U., and Heldt, H. W. (1981). The chloroplast envelope: structure, function, and role in leaf metabolism. Annu. Rev. Plant Physiol. 32, 139–168. doi: 10.1146/annurev.pp.32.060181.001035
Heber, U., and Krause, G. H. (1971). “Hydrogen and proton transfer across the chloroplast envelope,” in Proceedings of the 2nd International Congress on Photosynthesis (The Hague: N. V. Junk), 1023–1033.
Heibert, T., Steinkamp, T., Hinnah, S., Schwarz, M., Flueggel, U.-I., Weber, A., et al. (1995). Ion channels in the chloroplast envelope membrane. Biochemistry 34, 15906–15917. doi: 10.1021/bi00049a005
Heldt, H. W., Werdan, K., Milovancev, M., and Geller, G. (1973). Alkalization of the chloroplast stroma caused by light dependent proton flux into the thylakoid space. Biochim. Biophys. Acta 314, 224–241. doi: 10.1016/0005-2728(73)90137-0
Hochmal, A. K., Schulze, S., Trompelt, K., and Hippler, M. (2015). Calcium-dependent regulation of photosynthesis. Biochim. Biophys. Acta 1847, 993–1003. doi: 10.1016/j.bbabio.2015.02.010
Jäger-Vottero, P., Dorne, A.-J., Jordanov, J., Douce, R., and Joyard, J. (1997). Redox chains in chloroplast envelope membranes: spectroscopic evidence for the presence of electron carriers, including iron–sulfurcenters. Proc. Natl. Acad. Sci. U.S.A. 94, 1597–1602. doi: 10.1073/pnas.94.4.1597
Katoh, A., Sonoda, M., Katoh, H., and Ogawa, T. (1996). Absence of light-induced proton extrusion in a cotA-less mutant of Synechocystis sp. strain PCC6803. J. Bacteriol. 178, 5452–5455.
Kovacs-Bogdan, E., Soll, J., and Bolter, B. (2010). Protein import into chloroplasts: the Tic complex and its regulation. Biochim. Biophys. Acta 1803, 740–747. doi: 10.1016/j.bbamcr.2010.01.015
Kramer, D. M., Cruz, J. A., and Kanazawa, A. (2003). Balancing the central roles of the thylakoid proton gradient. Trends Plant Sci. 8, 27–32. doi: 10.1016/S1360-1385(02)00010-9
Krause, G. H. (1977). Light-induced movement of magnesium ions in intact chloroplasts. Spectroscopic determination with Eriochrome Blue SE. Biochim. Biophys. Acta 460, 500–510. doi: 10.1016/0005-2728(77)90088-3
Kunz, H.-H., Gierth, M., Herdean, A., Satoh-Cruz, M., Kramer, D. M., Spetea, C., et al. (2014). Plastidial transporters KEA1, -2, and -3 are essential for chloroplast osmoregulation, integrity, and pH regulation in Arabidopsis. Proc. Natl. Acad. Sci. U.S.A. 111, 7480–7485. doi: 10.1073/pnas.1323899111
Marmagne, A., Vinauger-Douard, M., Monachello, D., de Longevialle, A. F., Charon, C., Allot, M., et al. (2007). Two members of the Arabidopsis CLC (chloride channel) family, AtCLCe and AtCLCf, are associated with thylakoid and Golgi membranes, respectively. J. Exp. Bot. 58, 3385–3393. doi: 10.1093/jxb/erm187
Martiniere, A., Bassil, E., Jublanc, E., Alcon, C., Reguera, M., Sentenac, H., et al. (2013). In vivo intracellular pH measurements in tobacco and Arabidopsis reveal an unexpected pH gradient in the endomembrane system. Plant Cell 25, 4028–4043. doi: 10.1105/tpc.113.116897
Mi, F., Peters, J. S., and Berkowitz, G. A. (1994). Characterization of a chloroplast inner envelope K+ channel. Plant Physiol. 105, 955–964. doi: 10.1104/pp.105.3.955
Miyaji, T., Kuromori, T., Takeuchi, Y., Yamaji, N., Yokosho, K., Shimazawa, A., et al. (2015). AtPHT4;4 is a chloroplast-localized ascorbate transporter in Arabidopsis. Nat. Commun. 6, 5928. doi: 10.1038/ncomms6928
Muller, M., Kunz, H. H., Schroeder, J. I., Kemp, G., Young, H. S., and Neuhaus, H. E. (2014). Decreased capacity for sodium export out of Arabidopsis chloroplasts impairs salt tolerance, photosynthesis and plant performance. Plant J. 78, 646–658. doi: 10.1111/tpj.12501
Muller, P., Li, X. P., and Niyogi, K. K. (2001). Non-photochemical quenching. A response to excess light energy. Plant Physiol. 125, 1558–1566. doi: 10.1104/pp.125.4.1558
Murata, Y., and Takahashi, M. (1999). An alternative electron transfer pathway mediated by chloroplast envelope. Plant Cell Physiol. 40, 1007–1013. doi: 10.1093/oxfordjournals.pcp.a029481
Nobel, P. S., Chang, D. T., Wang, C. T., Smith, S. S., and Barcus, D. E. (1969). Initial ATP formation, NADP reduction, CO(2) fixation, and chloroplast flattening upon illuminating pea leaves. Plant Physiol. 44, 655–661. doi: 10.1104/pp.44.5.655
Pittman, J. K. (2012). Multiple transport pathways for mediating intracellular pH homeostasis: the contribution of H(+)/ion exchangers. Front. Plant Sci. 3:11. doi: 10.3389/fpls.2012.00011
Pottosin, I. I., Muniz, J., and Shabala, S. (2005). Fast-activating channel controls cation fluxes across the native chloroplast envelope. J. Membr. Biol. 204, 145–156. doi: 10.1007/s00232-005-0758-3
Roh, M. H., Shingles, R., Cleveland, M. J., and McCarty, R. E. (1998). Direct measurement of calcium transport across chloroplast inner-envelope vesicles. Plant Physiol. 118, 1447–1454. doi: 10.1104/pp.118.4.1447
Rolland, N., Dorne, A. J., Amoroso, G., Sultemeyer, D. F., Joyard, J., and Rochaix, J. D. (1997). Disruption of the plastid ycf10 open reading frame affects uptake of inorganic carbon in the chloroplast of Chlamydomonas. EMBO J. 16, 6713–6726. doi: 10.1093/emboj/16.22.6713
Roth, C., Menzel, G., Petétot, J.-C., Rochat-Hacker, S., and Poirier, Y. (2004). Characterization of a protein of the plastid inner envelope having homology to animal inorganic phosphate, chloride and organic-anion transporters. Planta 218, 406–416. doi: 10.1007/s00425-003-1121-5
Sawada, Y., Toyooka, K., Kuwahara, A., Sakata, A., Nagano, M., Saito, K., et al. (2009). Arabidopsis bile acid:sodium symporter family protein 5 is involved in methionine-derived glucosinolate biosynthesis. Plant Cell Physiol. 50, 1579–1586. doi: 10.1093/pcp/pcp110
Scherer, S., Hinrichs, I., and Boger, P. (1986). Effect of monochromatic light on proton efflux of the blue-green alga Anabaena variabilis. Plant Physiol. 81, 939–941. doi: 10.1104/pp.81.3.939
Schumacher, K. (2014). pH in the plant endomembrane system-an import and export business. Curr. Opin. Plant Biol. 22, 71–76. doi: 10.1016/j.pbi.2014.09.005
Shabala, S., and Pottosin, I. (2010). “Potassium and potassium-permeable channels in plant salt tolerance,” in Ion Channels and Plant Stress Responses, eds V. Demidchik and F. Maathuis (Heidelberg: Springer Berlin), 87–110.
Shen, J., Zeng, Y., Zhuang, X., Sun, L., Yao, X., Pimpl, P., et al. (2013). Organelle pH in the Arabidopsis endomembrane system. Mol. Plant 6, 1419–1437. doi: 10.1093/mp/sst079
Sheng, P., Tan, J., Jin, M., Wu, F., Zhou, K., Ma, W., et al. (2014). Albino midrib 1, encoding a putative potassium efflux antiporter, affects chloroplast development and drought tolerance in rice. Plant Cell Rep. 33, 1581–1594. doi: 10.1007/s00299-014-1639-y
Shingles, R., and McCarty, R. E. (1994). Direct measurement of ATP-dependent proton concentration changes and characterization of a K+-stimulated ATPase in pea chloroplast inner envelope vesicles. Plant Physiol. 106, 731–737.
Takizawa, K., Cruz, J. A., Kanazawa, A., and Kramer, D. M. (2007). The thylakoid proton motive force in vivo. Quantitative, non-invasive probes, energetics, and regulatory consequences of light-induced pmf. Biochim. Biophys. Acta 1767, 1233–1244. doi: 10.1016/j.bbabio.2007.07.006
Tomizioli, M., Lazar, C., Brugiere, S., Burger, T., Salvi, D., Gatto, L., et al. (2014). Deciphering thylakoid sub-compartments using a mass spectrometry-based approach. Mol. Cell. Proteomics 13, 2147–2167. doi: 10.1074/mcp.M114.040923
Tsunekawa, K., Shijuku, T., Hayashimoto, M., Kojima, Y., Onai, K., Morishita, M., et al. (2009). Identification and characterization of the Na+/H+ antiporter NhaS3 from the thylakoid membrane of Synechocystis sp. PCC 6803. J. Biol. Chem. 284, 16513–16521. doi: 10.1074/jbc.M109.001875
Veley, K. M., Marshburn, S., Clure, C. E., and Haswell, E. S. (2012). Mechanosensitive channels protect plastids from hypoosmotic stress during normal plant growth. Curr. Biol. 22, 408–413. doi: 10.1016/j.cub.2012.01.027
Versaw, W. K., and Harrison, M. J. (2002). A chloroplast phosphate transporter, PHT2;1, influences allocation of phosphate within the plant and phosphate-starvation responses. Plant Cell 14, 1751–1766. doi: 10.1105/tpc.002220
Wang, X., Berkowitz, G. A., and Peters, J. S. (1993). K+-conducting ion channel of the chloroplast inner envelope: functional reconstitution into liposomes. Proc. Natl. Acad. Sci. U.S.A. 90, 4981–4985. doi: 10.1073/pnas.90.11.4981
Werdan, K., Heldt, H. W., and Milovancev, M. (1975). The role of pH in the regulation of carbon fixation in the chloroplast stroma. Studies on CO2 fixation in the light and dark. Biochim. Biophys. Acta 396, 276–292. doi: 10.1016/0005-2728(75)90041-9
Wu, W., and Berkowitz, G. A. (1992). Stromal pH and photosynthesis are affected by electroneutral K and H exchange through chloroplast envelope ion channels. Plant Physiol. 98, 666–672. doi: 10.1104/pp.98.2.666
Keywords: proton gradient, chloroplast, thylakoid, envelope, cation/H+ exchanger
Citation: Höhner R, Aboukila A, Kunz H-H and Venema K (2016) Proton Gradients and Proton-Dependent Transport Processes in the Chloroplast. Front. Plant Sci. 7:218. doi: 10.3389/fpls.2016.00218
Received: 03 November 2015; Accepted: 08 February 2016;
Published: 29 February 2016.
Edited by:
Irene Murgia, Università degli Studi di Milano, ItalyReviewed by:
Ralf Bernd Klösgen, Martin Luther University of Halle-Wittenberg, GermanyCopyright © 2016 Höhner, Aboukila, Kunz and Venema. This is an open-access article distributed under the terms of the Creative Commons Attribution License (CC BY). The use, distribution or reproduction in other forums is permitted, provided the original author(s) or licensor are credited and that the original publication in this journal is cited, in accordance with accepted academic practice. No use, distribution or reproduction is permitted which does not comply with these terms.
*Correspondence: Hans-Henning Kunz, aGVubmluZy5rdW56QHdzdS5lZHU= Kees Venema, a2V2QGVlei5jc2ljLmVz
Disclaimer: All claims expressed in this article are solely those of the authors and do not necessarily represent those of their affiliated organizations, or those of the publisher, the editors and the reviewers. Any product that may be evaluated in this article or claim that may be made by its manufacturer is not guaranteed or endorsed by the publisher.
Research integrity at Frontiers
Learn more about the work of our research integrity team to safeguard the quality of each article we publish.