- 1Department of Plant Pathology and the Stakman-Borlaug Center for Sustainable Plant Health, University of Minnesota, St. Paul, MN, USA
- 2Agriculture, Commonwealth Scientific and Industrial Research Organisation, Canberra, ACT, Australia
- 3Agriculture, Centre for Environment and Life Sciences, Commonwealth Scientific and Industrial Research Organisation, Perth, WA, Australia
- 4Faculty of Agriculture and Environment, Plant Breeding Institute, The University of Sydney, Narellan, NSW, Australia
- 5Cereal Disease Laboratory, United States Department of Agriculture-Agricultural Research Service, St. Paul, MN, USA
The recent resurgence of wheat stem rust caused by new virulent races of Puccinia graminis f. sp. tritici (Pgt) poses a threat to food security. These concerns have catalyzed an extensive global effort toward controlling this disease. Substantial research and breeding programs target the identification and introduction of new stem rust resistance (Sr) genes in cultivars for genetic protection against the disease. Such resistance genes typically encode immune receptor proteins that recognize specific components of the pathogen, known as avirulence (Avr) proteins. A significant drawback to deploying cultivars with single Sr genes is that they are often overcome by evolution of the pathogen to escape recognition through alterations in Avr genes. Thus, a key element in achieving durable rust control is the deployment of multiple effective Sr genes in combination, either through conventional breeding or transgenic approaches, to minimize the risk of resistance breakdown. In this situation, evolution of pathogen virulence would require changes in multiple Avr genes in order to bypass recognition. However, choosing the optimal Sr gene combinations to deploy is a challenge that requires detailed knowledge of the pathogen Avr genes with which they interact and the virulence phenotypes of Pgt existing in nature. Identifying specific Avr genes from Pgt will provide screening tools to enhance pathogen virulence monitoring, assess heterozygosity and propensity for mutation in pathogen populations, and confirm individual Sr gene functions in crop varieties carrying multiple effective resistance genes. Toward this goal, much progress has been made in assembling a high quality reference genome sequence for Pgt, as well as a Pan-genome encompassing variation between multiple field isolates with diverse virulence spectra. In turn this has allowed prediction of Pgt effector gene candidates based on known features of Avr genes in other plant pathogens, including the related flax rust fungus. Upregulation of gene expression in haustoria and evidence for diversifying selection are two useful parameters to identify candidate Avr genes. Recently, we have also applied machine learning approaches to agnostically predict candidate effectors. Here, we review progress in stem rust pathogenomics and approaches currently underway to identify Avr genes recognized by wheat Sr genes.
Introduction
The basidiomycete fungus Puccinia graminis f. sp. tritici (Pgt) causes stem rust or black rust, one of the most devastating diseases affecting common and durum wheat, barley, and triticale (Leonard and Szabo, 2005; Park, 2007). While both barley and triticale are valuable dietary and industrial crops, the menace that stem rust poses to wheat production is the most feared. Wheat provides one fifth of the calories and protein intake for human consumption across the globe. The world's wheat production for 2015 is estimated to be more than 733 million tons (FAO, 2015), a number that will need to be more than doubled to meet supply demands projected by the year 2050 (Wheat, 2014). Pgt has a wide geographical distribution, and it can destroy a wheat field entirely in as little as 3 weeks (Leonard and Szabo, 2005). For these reasons, Pgt has been ranked as one of the most economically important plant pathogens and threats to global food security (Dean et al., 2012).
In 1998, a new highly virulent race of Pgt, referred to as TTKSK (synonym Ug99), was detected in Uganda (Pretorius et al., 2000). This race overcame the long term protection that had been provided by the stem rust resistance gene Sr31, used widely in many parts of the world. Since then, at least eight variants of the Ug99 race group have appeared, rendering additional resistance genes ineffective (Singh et al., 2011, 2015). The steady geographical spread and rapid evolution of the Ug99 race group poses a significant threat, with 80 and 95% of global wheat and barley cultivars respectively considered susceptible (Singh et al., 2011; Steffenson et al., 2013). This threat has led to a major worldwide investment in improving genetic resistance to wheat stem rust through identifying and incorporating new sources of resistance effective against this race group. However, in 2013-2014, Ethiopia suffered a devastating stem rust outbreak caused by an unrelated Pgt race (TKTTF) which defeated the resistance gene SrTmp in the widely cultivated wheat variety “Digalu,” introduced to provide protection against the Ug99 race group (Olivera et al., 2015). Similarly, in 2014 Kenya experienced significant losses in fields of the wheat variety “Robin,” which also carries SrTmp, in this case caused by a new race in the Ug99 lineage (Singh et al., 2015; Patpour et al., 2016). Thus, there is an ongoing need to not only diversify sources of resistance with a broader focus beyond the Ug99 lineage, but also to ensure that multiple Sr genes are deployed in combination to guard against future stem rust epidemics.
Currently the best strategy for achieving long-lasting resistance involves the use of several different stem rust resistance (Sr) genes with complementary race specificity, in combination with non-specific partial resistance genes (Ellis et al., 2014). However, there is a challenge in identifying the optimal genes and gene combinations to minimize the chance of new virulent races arising. Because most Sr genes confer race-specific resistance based on recognition of pathogen avirulence (Avr) genes (McIntosh et al., 1995; Zambino et al., 2000), knowledge of the specific genes underlying virulence and their population genetics is critical to informing resistance deployment strategies. What are the pathogen Avr genes recognized by important Sr genes? How variable are they in local and global Pgt populations? What is the level of homozygosity/heterozygosity at these loci and thus their propensity to mutate to virulence? Some Sr genes from different sources cannot be distinguished because they provide resistance to all known pathotypes of Pgt, but do they in fact recognize different Avr genes? Lastly, the recent cloning of several Sr genes, including Sr33 (Periyannan et al., 2013), Sr35 (Saintenac et al., 2013), Sr50 (Mago et al., 2015), Sr55 (Moore et al., 2015), and Sr57 (Krattinger et al., 2009) brings the possibility of developing multigene transgenic stacks that segregate as a single locus (Ellis et al., 2007; Wulff et al., 2011; McDonald, 2014). Because pyramids of multiple broadly effective resistance genes confound the confirmation of individual gene function through pathogenicity assays, it will be important to generate tools allowing their independent assay based on knowledge of their corresponding Avr genes. Here, we discuss the implications of recent advances in Pgt molecular genetics and genomics in the context of these research priorities and the underlying biology and history of stem rust disease.
Biology of the Stem Rust Fungus: Mastering Complexity to Survive
Pgt is a biotrophic fungus with a complex life cycle that includes asexual (clonal) reproduction on a cereal host (Poaceae), such as wheat, and sexual recombination on an alternate host (Berberidaceae; Leonard and Szabo, 2005). During the asexual phase, the fungus produces multitudes of single-celled dikaryotic urediniospores, which can mediate new cycles of infection approximately every 2 weeks. Urediniospores germinate on the leaf surface after exposure to moisture (Staples and Macko, 2004; Leonard and Szabo, 2005). The topology of the leaf surface helps the germ tube to locate a stomate where a specialized penetration structure, known as an appressorium, forms. The appressorium remains quiescent over the stomatal aperture until the formation of a penetration peg is triggered by exposure of the plant to light (Yirgou and Caldwell, 1968). Subsequently, the fungus forms a substomatal vesicle that gives rise to the primary infection hypha (Rowell et al., 1958). As the mycelium grows and comes into contact with a mesophyll cell, the fungus produces a specialized feeding structure, known as a haustorium (Harder and Chong, 1984; Staples and Macko, 2004). After formation of the first haustorium, the fungus branches to generate secondary infection hyphae and form additional haustoria. The haustorium penetrates the plant cell wall, and invaginates the plasma membrane creating a sealed compartment, referred to as the extrahaustorial matrix (Harder and Chong, 1984). In addition to nutrient uptake, these structures enable the delivery of effector proteins into the host cell that are thought to manipulate cellular processes to facilitate infection (Garnica et al., 2014). These elaborate morphological and molecular events take place during the first days of Pgt infection and macroscopic symptoms of disease usually manifest after 8–10 days, as urediniospore pustules erupt through the leaf or stem surface (Figures 1A–C; Leonard and Szabo, 2005). Yield reduction associated with stem rust infection in cereals is attributed to plant lodging, poor nutrient mobilization to support grain development, and decrease of photosynthetic capacity as the plant foliar area is severely damaged (Figure 1D; Leonard and Szabo, 2005).
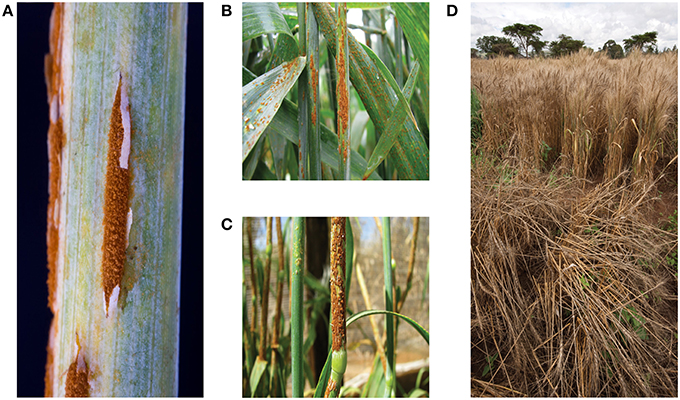
Figure 1. P. graminis f. sp. tritici (Pgt) infected wheat and barley. (A) Close-up of a Pgt uredinium after eruption through the epidermis of a barley stem. (B) Sporulation of African Pgt race PTKST on wheat (C) Sporulation of African Pgt race PTKST on barley. (D) Severe straw breakage of a susceptible wheat line (front) caused by heavy stem rust infection in comparison with a resistant wheat line (back) growing in a disease screening nursery in Kenya in 2008. Images (A,D) were taken by David Hansen/Brian Steffenson and (B,C) by Zacharias Pretorius.
During the asexual stage described above Pgt is dikaryotic (n + n), with each cell containing two haploid nuclei dikaryotic genetics. However, toward the end of the growing season, as the cereal host starts to senesce, the fungus may initiate the sexual stage through formation of diploid (2n) teliospores. These thick-walled, two-celled spores can remain dormant until the spring and then complete meiosis to generate haploid basidiospores that can infect the alternate host Berberis (barberry) or Mahonia (Roelfs, 1985; Roelfs and Groth, 1988; Boehm et al., 1992). The haploid infection stage culminates in the production pycnia, which contain two types of haploid gametes (Roelfs, 1985). Haploid pycniospores cross fertilize with receptive hyphae to re-establish dikaryotic mycelia that give rise to aeciospores, which can then infect the cereal host and re-initiate the asexual phase (Harder, 1984). Thus, in areas where the alternate host exists, the sexual cycle of the fungus can yield novel virulence phenotypes due to re-assortment and segregation of genetic variation.
KEY CONCEPT 1. Dikaryotic genetics
Pgt is a dikaryotic pathogen with an asexual cycle on wheat and barley, and a sexual cycle on an alternate host. Pgt therefore exhibits diploid genetics, with a virulent phenotype requiring homozygosity at the Avr locus.
Genetic Resistance to Stem Rust Disease: Racing Evolution
Race-specific resistance (R) genes, often known as “major” or “seedling” resistance genes in the wheat and barley context, are one of the fundamental resources utilized in disease resistance breeding programs. These R genes generally conform to the “gene-for-gene” model, and confer resistance to pathogen races carrying a corresponding Avr gene (Flor, 1971) race-specific resistance. Most known R genes encode intracellular immune receptor proteins belonging to the nucleotide-binding leucine-rich-repeat (NB-LRR) class (Dangl and Jones, 2001; Dodds and Rathjen, 2010). These receptors can recognize specific pathogen effector proteins (known as Avr proteins) that are translocated into the plant cell (Hogenhout et al., 2009; Koeck et al., 2011).
KEY CONCEPT 2. Race-specific resistance
Race-specific resistance to Pgt is mediated by ‘gene-for-gene’ type interactions that involve recognition of pathogen avirulence proteins by host resistance proteins.
The flax rust disease system provides the best defined model for rust disease resistance, with over 30 corresponding R and Avr genes defined genetically in the host (Linum usitatissimum) and pathogen (Melampsora lini), respectively, many of which are now cloned (Ravensdale et al., 2011). In this system, all the known R genes encode NB-LRR intracellular receptors, while the corresponding Avr genes encode small secreted proteins that are expressed in haustoria and delivered into host cells. There are over 50 race-specific stem rust resistance (Sr) genes described in wheat and its close relatives (McIntosh et al., 1995), a number of which have been cloned recently (Periyannan et al., 2013; Saintenac et al., 2013; Mago et al., 2015). As in flax, these all encode NB-LRR immune receptors, suggesting that the corresponding Pgt Avr proteins are likely to be secreted effectors delivered to host cells. Likewise in barley, the Rpg4/5 stem rust resistance locus encodes a pair of NB-LRR receptors that probably function in tandem (Brueggeman et al., 2008; Wang et al., 2013). Although R genes encoding cell surface immune receptors that recognize apoplastic effectors have been identified in some disease systems (Tax and Kemmerling, 2012; Du et al., 2015), such receptors have not been observed in rust resistance. However, the barley Rpg1 resistance gene is unusual in encoding an intracellular kinase (Brueggeman et al., 2002) and appears to respond to components present in the urediniospore that may be released early in infection (Nirmala et al., 2011).
Avr genes in flax rust are characterized by high levels of polymorphism between races and display signatures of diversifying selection (Catanzariti et al., 2006; Dodds et al., 2006; Barrett et al., 2009) as a result of the strong pressure to escape host immune recognition avirulence proteins. For instance, 12 variants of AvrL567 are known with different recognition properties determined by polymorphic residues exposed on the protein surface that interact with host immune receptors (Wang et al., 2007; Moore et al., 2015). Likewise, differences in recognition of AvrM variants by the corresponding M resistance protein are governed by surface-exposed polymorphic residues (Catanzariti et al., 2010; Ve et al., 2013). Effectors are probably also under selection to adapt to host proteins targeted by their pathogenicity functions. The only other basidiomycete avirulence gene identified to date is UhAvr1 from Ustilago hordei (causal agent of barley smut) and in this case virulence arose from a transposable element insertion in the promoter of the gene (Ali et al., 2014).
KEY CONCEPT 3. Avirulence proteins
Known Avr proteins of rust fungi are secreted from haustoria and delivered into host cells where they are recognized by NB-LRR immune receptors. Thus, there is strong selection pressure for Avr sequence diversification to allow the pathogen to escape recognition.
Additional genetic protection against rust pathogens can also occur through the action of race non-specific resistance genes. In wheat, some of these genes provide broad-spectrum but partial resistance against multiple pathogens and probably operate by physiological mechanisms independently of immune recognition. For instance Lr34 (syn. Sr57, Yr18, Pm38) confers resistance against stem, leaf and stripe, rust fungi as well as barley yellow dwarf virus and powdery mildew and encodes a putative adenosine triphosphate-binding cassette (ABC) transporter protein (Dyck et al., 1966; Singh, 1993; Krattinger et al., 2009; Risk et al., 2013). Likewise, Lr67 (syn. Sr55, Yr46, Pm46) also confers multi-pathogen resistance and encodes a hexose transporter (Moore et al., 2015). Because these non-specific genes may also enhance the effectiveness of race-specific genes, a desirable strategy to enhance genetic resistance is to employ combinations of both resistance gene classes (Ellis et al., 2014). Indeed North America, Mexico and Australia have been largely protected from stem rust epidemics since the 1950s, probably due to the continuous deployment of rust resistant wheat cultivars often with multiple resistance genes in the absence of significant sexual recombination in the pathogen populations (Leonard and Szabo, 2005; Park, 2007; Ellis et al., 2014).
Genetic Variability of Pgt: the Code for Success
A significant challenge for resistance breeding is the “boom and bust” cycle resulting from the emergence of new pathogen variants or races that overcome single major resistance genes. Although many Sr genes have been deployed in the field, resistance breakdown occurs frequently due to genetic changes in existing local Pgt races or the migration of new races, as exemplified by the Ug99 race group (Stokstad, 2007) sources of genetic variation. Indeed, Pgt is genetically diverse and many different physiological races have been described by systematic classification systems based on the disease phenotypes (infection types) induced in a standard set of differential lines containing known Sr genes (Roelfs and Martens, 1988; Jin et al., 2008). The differential host set has often been modified or extended in different regions based on locally important Sr genotypes (Park, 2008). These classification systems reflect the genetic make-up of the Avr gene repertoires within isolates, and have been a very useful tool to monitor pathogen types in field surveys and detect changes in virulence.
KEY CONCEPT 4. Sources of genetic variation
Genetic variability in Pgt can occur via multiple mechanisms, such as random mutations in clonal lineages, sexual recombination in geographical regions where the alternate host is present, and somatic hybridization.
Stem rust epidemics occur intermittently in regions with warm and humid weather conditions and references to recurrent disease outbreaks date back to ancient Roman times (Dubin and Brennan, 2009). Stem rust epidemics have impacted wheat production in the United States (US) from the late 1800's. The two most devastating epidemics occurred in 1935 and 1953-1954. In the epidemic of 1935 yield losses recorded in the upper Midwest (Minnesota and North Dakota) reached more than 50% (Roelfs, 1978). The 1953-1954 epidemics were responsible for the loss of more than 75% of the US durum wheat (Stakman and Harrar, 1957). In response to disease outbreaks in the early 1900s, a barberry eradication program was initiated throughout the northern Great Plains and lasted for nearly a half of century. As a result the number of epidemics and diversity of the Pgt population has been dramatically reduced (Roelfs, 1982). Since then, there has been only one epidemic reported to the US and Canada in 1974 (Roelfs, 1978; Leonard and Szabo, 2005). Currently, in the primary wheat growing regions of the United States (east of the Rocky Mountains) the Pgt population is primarily composed of a single race and stem rust is rarely observed in commercial wheat production fields (Jin et al., 2014). Another important factor that has helped reduce the complexity of the Pgt population in Great Plains is the inability of the stem rust pathogen to survive the winter season in the colder regions, except for along the Gulf Coast. Due to this genetic bottleneck, the development of stem rust in the central and northern Great Plains is dependent on the transport of fresh inoculum from the Gulf Coast region. This annual northward movement of fungal spores has been referred to as the Puccinia Pathway. The use of rust resistant varieties in Mexico by CIMMYT in the 1950's and 1960's, led by Norman Borlaug (Borlaug, 1968) may have decreased the amount of inoculum migrating from Northern Mexico into the Puccinia Pathway, and thus helped to reducing the severity and incidence of the disease.
Similarly, Australia has also struggled with the economic losses caused by Pgt. Some of the significant epidemics of stem rust in Australia occurred in the late 1800's, followed by the 1947-1948 epidemic when the state of New South Wales lost ~12% of grain yield (Stakman and Harrar, 1957; Dubin and Brennan, 2009) and finally in 1973 when the country faced the worst outbreak leading to AU$100-200 million losses (Watson, 1981; McIntosh et al., 1995; Park, 2007). Surveys of Pgt have indicated that it was introduced into the Australian continent on at least three occasions since 1921 (Park, 2007). Because barberry is not present in Australia, the pathogen has evolved largely clonally with three or four independent lineages recognized, each derived from an independent introduction. The annual surveys of Pgt races across the Australian wheat belt for over 90 years provide a detailed history of changes in virulence in these lineages over time (Park, 2007). The predominant evolutionary mechanism involves changes in which single virulence phenotypes are acquired sequentially by new races within a Pgt lineage. This is most likely due to mutations occurring within single Avr genes. For example, the detection of virulence for the resistant wheat cultivars Eureka (Sr6), Gabo (Sr11), Spica (Sr17), and Festival (Sr9b) soon after their release was considered to be the result of mutation (Waterhouse, 1952; Watson, 1958). Experiments under controlled conditions demonstrated the acquisition of virulence for resistance genes following exposure to the chemical mutagen Ethyl Methane Sulphonate (Luig, 1978; Gates and Loegering, 1991). Luig (1978) showed that Avr genes vary in their mutability, with mutations to virulence for the resistance genes Sr5, Sr9e, and Sr21 being relatively common, while mutations to virulence for Sr26 were not observed. It was suggested that the Avr genes present in Pgt have intrinsic differences. Because Pgt is dikaryotic, the probability of virulence arising should differ according to whether a single mutation (in a race heterozygous for avirulence) or two mutations (in a homozygote) are required. This may explain some of the observed differences in the durability of Sr genes in particular environments with varying pathogen populations. Alternatively, some virulence mutations may impose fitness costs.
Somatic hybridization, which involves fusion of dikaryotic hyphae from different isolates and either nuclear exchange or parasexual recombination and exchange of chromosomes, is another mechanisms that may introduce variability in the absence of sexual recombination (Park and Wellings, 2012). For instance, the Australian pathotype Pgt 34-2-11 probably emerged as the result of somatic hybridization between isolates belonging to race 126 and race 21 (Watson, 1981). Wang and McCallum found that germinating urediniospores of the wheat leaf fungus, Puccinia triticina, can undergo anastomosis and nuclear migration (Wang and McCallum, 2009). The genomics tools now available will help to determine the frequency and role of somatic hybridization in the evolution of Pgt populations.
Clearly, identifying Avr genes from Pgt is a priority to enhance crop protection strategies. However, the biotrophic lifestyle of rust fungi has posed experimental constraints on development of genetic tools, such as transformation and gene-knockout strategies, that are used in other fungi. Sequencing advances have greatly enhanced the amenability of these pathogens for experimental analysis, with genome assemblies now available for several rust fungi, including those causing wheat stem, leaf and stripe rust, poplar leaf rust, and flax rust (Duplessis et al., 2011; Cantu et al., 2013; Zheng et al., 2013; Nemri et al., 2014) (http://www.broadinstitute.org/annotation/genome/puccinia_group/MultiHome.html).
A high quality reference genome sequence for a North American Pgt isolate, CDL 75-36-700-3, was assembled based on Sanger sequencing of plasmid and fosmid clones (Duplessis et al., 2011). This approach resulted in a highly connected assembly spanning 88.6 Mbp in just 392 scaffolds of 4557 contigs (81.5 Mbp sequence length). A total of 15,979 genes were annotated based on a combination of in silico predictions, EST sequences and RNAseq transcript analysis. Resequencing of the Pgt reference isolate using Illumina 76b paired-end reads identified about 129,000 single nucleotide polymorphisms (SNPs) between the two haploid genomes in this isolate. Subsequent analysis of global isolates has led to the development of a high throughput SNP genotyping array. The PgtSNP 1.5 k chip has been used to genotype Pgt isolates from Northeast Africa to examine the genetic relationship between races of Pgt (Olivera et al., 2015). This analysis clearly demonstrated that the Pgt race TKTTF, which caused the 2013-14 wheat stem rust epidemic in Ethiopia, was not derived from Ug99 lineage or other common races in the region. In addition, the Ethiopian isolates of race TKTTF were found to represent two distinct subclades, indicating that evolution of this race was not a recent event. Current genotyping of Pgt isolates from Europe, Middle East and Central Asia supports this hypothesis. Thus, SNP genotyping provides a rapid tool for surveillance of critical Pgt race groups in regions where populations are primarily asexual. In addition, this tool allows population genetics studies to examine Pgt population structure and evolution and is currently being use to analyze the Ug99 lineage. An important question to address is whether sexual recombination contributes to Pgt population diversity in Africa.
The reference sequence has also provided an excellent resource to facilitate broader genomic analyses of Pgt. Upadhyaya et al. (2015) used this reference in combination with Illumina sequencing to build a pan-genome of Australian Pgt isolates. This was based on sequences of isolates representing founder races for the four clonal lineages present in Australia. In addition to the reference-based assembly, a de novo assembly of unaligned reads was also used to give a final 95 Mbp pan-genome. The additional ~15 Mbp de novo assembled sequence in this genome is not race specific, because it was present in all four independent Australian Pgt isolates, and indeed also in unassembled reads from CDL 75-36-700-3. Only low amounts of race-specific sequence, < 0.5 Mbp, represented by numerous short contigs, were observed. This suggests that there are few large scale genome structural differences between Pgt races, unlike observations in other pathogenic fungi such as Fusarium (Ma et al., 2010) and Magnaporthe (Yoshida et al., 2009). However, very high rates of heterozygosity were observed, with around a million SNPs detected within each race representing diversity between the two haploid nuclei (Upadhyaya et al., 2015).
Identifying putative Avr genes in the Pgt genome is not simple. The key criteria that can be extrapolated from the known flax rust Avr genes are quite broad; they should encode secreted proteins and should be expressed in haustoria effector candidates. In contrast to oomycetes (Bozkurt et al., 2012), there are no conserved sequence motifs uniting these genes into a readily identified set (Saunders et al., 2012; Garnica et al., 2014; Nemri et al., 2014). Upadhyaya et al. (2015) annotated a total of 22,391 expressed gene sequences in the pan-genome assembly based on RNAseq transcripts from isolated haustoria and germinated spores. About 20% of the genes showed differential expression between haustoria and germinated spores, reflecting different biological processes in these cell types. A total of 520 effector candidates were predicted based on the criteria of encoding secreted proteins without additional membrane spanning domains (1924 in total in the genome) and showing either greater than two-fold upregulation or high expression (>100 RPKM) in haustoria. Similarly, genomic and transcriptomic studies on other rust fungi have revealed large sets (500–1500) of potential effector genes (Cantu et al., 2011, 2013; Duplessis et al., 2011; Hacquard et al., 2012; Garnica et al., 2013; Link et al., 2014; Nemri et al., 2014).
KEY CONCEPT 5. Effector candidates
The Pgt genome contains over 500 genes predicted to encode secreted proteins that are preferentially expressed in haustoria. These genes comprise a set of most likely Avr gene candidates.
The large numbers of putative effectors in rust genomes make it difficult to prioritize candidates for functional studies. To address this challenge, other unbiased effector prediction approaches have been developed. Saunders et al. (2012) used a method to rank predicted gene families (“tribes”) according to their likelihood of containing effectors based on a combination of properties associated with known effectors such as a predicted secretion signal, small size, high number of cysteines, in planta induction, and their genomic location. Whilst this method is powerful for finding expanded effector gene families in rust fungi, it does not consider effector genes that are not part of such families. Sperschneider et al. (2014) used comparative genomics to classify proteins into those that are generally conserved across fungi and those that are specifically associated with pathogenic fungi, including those that are specific to rust fungi. This analysis revealed that in planta up-regulated genes that are under diversifying selection are found almost exclusively in pathogen-associated gene families. In particular, a small set of 42 high-confidence effectors was identified that are rapidly evolving within the Puccinia genus, consistent with co-evolution with host R genes or virulence targets. While adaptive evolution analysis is a powerful approach for prioritizing effector candidates, it can only be applied to genes for which distantly diverged sequences or population genomics data are available. More recently, a machine learning approach has been developed to make effector predictions in fungi without making assumptions on their protein properties, and can complement other approaches to identify novel rust effectors (Sperschneider et al., 2015).
Genetic and Functional Tools: Associating Genotype and Phenotype
Genome sequencing and effector predictions have provided a critical resource for Pgt virulence studies, but the determination of Avr gene function will depend on genetic and functional evidence to associate these genes with avirulence/virulence phenotypes identifying Avr genes. In the flax rust system, a mapping family segregating for multiple Avr loci was essential for identification of Avr genes (Dodds et al., 2004; Catanzariti et al., 2006). A similar resource is available in Pgt, with an F2 family population of 81 individuals developed from a cross between two North American strains, including CDL 75-36-700-3, (Zambino et al., 2000). This family segregates for avirulence/virulence on 10 Sr genes, eight of which segregate as a single dominant genes. Genome-wide mapping of SNPs is currently being undertaken to anchor these onto genome scaffolds and thereby identify linked effector candidates, and additional segregating populations are also being generated.
KEY CONCEPT 6. Identifying Avr genes
Genetic variation for virulence in segregating populations, clonal lineages and derived mutants allows association of sequence variation in gene candidates with avirulence phenotypes.
The Australian Pgt collection also provides an excellent genetic resource for Avr gene identification. This asexual pathogen population falls into clonal lineages that have largely evolved by sequential mutation to overcome specific Sr genes. The extensive pathotype analysis from the University of Sydney annual pathogen surveys provides the necessary phenotypic data to correlate gene sequences with specific virulence changes. Upadhyaya et al. (2015) initiated an analysis of this material focusing on two field isolates within the race 21 lineage that had acquired virulence on four additional resistance genes (Sr5, Sr11, Sr27, SrSatu). Comparison of these genome sequences with the original 21-0 progenitor pathotype identified a total of 25 effector candidates containing sequence changes that could be responsible for conferring these virulence phenotypes.
Both of these resources are however limited by the genetic variation that occurs within a single segregating family or collection of field isolates, which mostly represents Sr genes that have been used historically and subsequently overcome. Identifying Avr genes corresponding to important new Sr genes will require a different approach. A promising strategy at this time is to use a mutagenesis approach to generate genetic diversity that can then be associated with sequence and virulence changes. The feasibility of this approach was demonstrated in early mutagenesis experiments (Luig, 1978). Because Pgt is dikaryotic, the genotype of the parental race (homozygous or heterozygous) will affect the ease of identifying virulence mutations. Indeed, spontaneous virulence mutants provided a valuable resource in cloning Avr genes from M. lini, but were only detected for heterozygous Avr loci (Dodds et al., 2004; Catanzariti et al., 2006). Thus the use of genetically diverse Pgt isolates in mutagenesis experiments is advantageous because the mutability of different races is likely to differ on specific Sr genes. Recently, a spontaneous mutant of Pgt virulent to the broadly effective and now cloned Sr50 was identified (Mago et al., 2015). Sequencing experiments are underway to determine the nature of the genetic alteration in this race. We are also conducting new screens for both spontaneous and EMS-induced virulent Pgt mutants against a range of Sr genes to identify new Avr gene candidates.
Lastly, confirmation of the identity of genetically defined Avr genes will require demonstration of activity through functional assays. In many disease systems, this can be achieved by either transformation of a virulent pathogen to test for an avirulent phenotype, or by transient expression of the candidate effector in a resistant host to test for defense induction. Although transformation of flax rust is possible, it relies on silencing a previously identified Avr gene for selection (Lawrence et al., 2010) and no transformation system has yet been developed for Pgt or other rust fungi. Likewise Agrobacterium-mediated transient protein expression is not available for wheat. Other potential assays include bacterial systems developed in Pseudomonas and Xanthomonas species that can deliver effector candidates via Type 3 Secretion (Sohn et al., 2007; Thomas et al., 2009). Recently wheat compatible versions of these delivery systems have become available (Yin and Hulbert, 2011; Upadhyaya et al., 2014). Other possibilities include generating transgenic plants expressing Avr candidates and crossing these to the Sr resistant line to test for cell death defense induction and transient expression by biolistic transformation (Jones et al., 1994; Leister et al., 1996; Jia et al., 2000). Host induced gene silencing of candidate genes may also provide a useful functional assay (Panwar et al., 2013; Yin et al., 2015). Where specific Sr genes have been cloned, these may be co-expressed with the Avr candidates in a heterologous system, such as Nicotiana benthamiana. This approach was used recently to confirm the identity of AvrPm3f from wheat powdery mildew as it triggered cell death when recognized by the corresponding wheat Pm3f resistance gene (Bourras et al., 2015).
Future Perspectives
Keeping stem rust at bay has not been an easy task; however, for over 80 years breeding for disease resistance and the deployment of resistant cultivars has been a powerful strategy to lessen the frequency of stem rust disease outbreaks (Leonard and Szabo, 2005). Nonetheless, stem rust continues to threaten grain production and is a serious problem in regions of the world where small-scale farming plays a pivotal role in agriculture and economic resources are limited. One lesson we have learned is that the durability of genetic resistance depends on the manner in which it is deployed. Employing multiple resistance genes is essential to reduce the likelihood of new virulent variants emerging. This strategy will require careful selection of Sr genes to be deployed in new wheat cultivars, informed by knowledge of the corresponding Avr genes and their population genetics. In light of this, the identification of effectors in Pgt and understanding the underlying mechanisms that generate genetic variability in Pgt are two research priorities. The coordinated international surveillance efforts led by the Borlaug Global Rust Initiative and the Durable Rust Resistance in Wheat Project during the last 8 years have created a platform to enable pathogenomics studies in recently emerged virulent isolates from multiple points of origin. In combination with historical collections of Pgt isolates these resources provide a valuable experimental system to address these research priorities. Recent breakthroughs in cloning Sr genes from wheat have provided tools such as perfect molecular markers to facilitate the production of gene pyramids or stacks. In parallel, these advances also provide materials for generating transgenic events with multiple Sr genes at a single location which will greatly simplify the breeding constraints of multiple unlinked genes. Cloned Avr genes will provide a means to verify the function of individual Sr genes in such combinations. The ability to rotate between stacks with different R gene components would also contribute to resistance durability (McDonald, 2014). Finding new stem rust resistance genes means continuing laborious germplasm screens in crop varieties or related species (Rouse M. et al., 2011; Rouse M. N. et al., 2011). Additionally, non-host systems such as the grass Brachypodium distachyon may provide novel sources of resistance genes from outside the wheat and barley gene pool (Ayliffe et al., 2013; Figueroa et al., 2013, 2015). Overall, the existing genetic and genomic resources available to study Pgt (Zambino et al., 2000; Duplessis et al., 2011; Upadhyaya et al., 2015), wheat (Mayer et al., 2014), barley (The International Barley Genome Consortium, 2012), and B. distachyon (The International Brachypodium Initiative, 2010; Gordon et al., 2013) hold significant promise to strengthen genetic resistance approaches to battle stem rust.
Author Contributions
All authors listed, have made substantial, direct and intellectual contribution to the work, and approved it for publication.
Conflict of Interest Statement
The authors declare that the research was conducted in the absence of any commercial or financial relationships that could be construed as a potential conflict of interest.
Acknowledgments
Work in the authors' laboratories has been supported by funds provided through the Two Blades Foundation, the Grains Research and Development Corporation (US00063), start-up funds provided by the University of Minnesota, the Durable Rust Resistance in Wheat Project administered by Cornell University and supported by the Bill & Melinda Gates Foundation and the UK Department for International Development, Lieberman- Okinow Endowment at the University of Minnesota, and USDA-ARS.
Author Biography
References
Ali, S., Laurie, J. D., Linning, R., Cervantes-Chávez, J. A., Gaudet, D., and Baakeren, G. (2014). An immunity-triggering effector from the barley smut fungus Ustilgao hordei resides in an Ustilaginaceae-specific cluster bearing signs of transposable element-assisted evolution. PLoS Pathog. 10:e1004223. doi: 10.1371/journal.ppat.1004223
Ayliffe, M., Singh, D., Park, R., Moscou, M. J., and Pryor, T. (2013). The infection of Brachypodium distachyon with selected grass rust pathogens. Mol. Plant Microbe Interact. 26, 946–957. doi: 10.1094/MPMI-01-13-0017-R
Barrett, L. G., Thrall, P. H., Dodds, P. N., van der Merwe, M., Linde, C. C., Lawrence, G. J., et al. (2009). Diversity and evolution of effector loci in natural populations of the plant pathogen Melampsora lini. Mol. Biol. Evol. 26, 2499–2513. doi: 10.1093/molbev/msp166
Boehm, E., Wenstrom, J., McLaughlin, D., Szabo, L., Roelfs, A., and Bushnell, W. (1992). An ultrastructural pachytene karyotype for Puccinia graminis f. sp. tritici. Can. J. Bot. 70, 401–413. doi: 10.1139/b92-054
Borlaug, N. E. (1968). Wheat Breeding and its Impact on World Food Supply. Canberra: Australian Academy of Science.
Bourras, S., McNally, K. E., Ben-David, R., Parlange, F., Roffler, S., Praz, C. R., et al. (2015). Multiple avirulence loci and allele-specific effector recognition control the Pm3 race-specific resistance of wheat to powdery mildew. Plant Cell 27, 2991–3012. doi: 10.1105/tpc.15.00171
Bozkurt, T. O., Schornack, S., Banfield, M. J., and Kamoun, S. (2012). Oomycetes, effectors, and all that jazz. Curr. Opin. Plant Biol. 15, 483–492. doi: 10.1016/j.pbi.2012.03.008
Brueggeman, R., Druka, A., Nirmala, J., Cavileer, T., Drader, T., Rostoks, N., et al. (2008). The stem rust resistance gene Rpg5 encodes a protein with nucleotide-binding-site, leucine-rich, and protein kinase domains. Proc. Natl. Acad. Sci. U.S.A. 105, 14970–14975. doi: 10.1073/pnas.0807270105
Brueggeman, R., Rostoks, N., Kudrna, D., Kilian, A., Han, F., Chen, J., et al. (2002). The barley stem rust-resistance gene Rpg1 is a novel disease-resistance gene with homology to receptor kinases. Proc. Natl. Acad. Sci. U.S.A. 99, 9328–9333. doi: 10.1073/pnas.142284999
Cantu, D., Govindarajulu, M., Kozik, A., Wang, M., Chen, X., Kojima, K. K., et al. (2011). Next generation sequencing provides rapid access to the genome of Puccinia striiformis f. sp. tritici, the causal agent of wheat stripe rust. PLoS ONE 6:e24230. doi: 10.1371/journal.pone.0024230
Cantu, D., Segovia, V., MacLean, D., Bayles, R., Chen, X., Kamoun, S., et al. (2013). Genome analyses of the wheat yellow (stripe) rust pathogen Puccinia striiformis f. sp. tritici reveal polymorphic and haustorial expressed secreted proteins as candidate effectors. BMC Genomics 14:270. doi: 10.1186/1471-2164-14-270
Catanzariti, A. M., Dodds, P. N., Lawrence, G. J., Ayliffe, M. A., and Ellis, J. G. (2006). Haustorially expressed secreted proteins from flax rust are highly enriched for avirulence elicitors. Plant Cell 18, 243–256. doi: 10.1105/tpc.105.035980
Catanzariti, A.-M., Dodds, P. N., Ve, T., Kobe, B., Ellis, J. G., and Staskawicz, B. J. (2010). The AvrM effector from flax rust has a structured C-terminal domain and interacts directly with the M resistance protein. Mol. Plant Microbe Interact. 23, 49–57. doi: 10.1094/MPMI-23-1-0049
Consortium, I. B. G. S. (2012). A physical, genetic and functional sequence assembly of the barley genome. Nature 491, 711–716. doi: 10.1038/nature11543
Dangl, J. L., and Jones, J. D. (2001). Plant pathogens and integrated defence responses to infection. Nature 411, 826–833. doi: 10.1038/35081161
Dean, R., Van Kan, J. A., Pretorius, Z. A., Hammond-Kosack, K. E., Di Pietro, A., Spanu, P. D., et al. (2012). The Top 10 fungal pathogens in molecular plant pathology. Mol. Plant Pathol. 13, 414–430. doi: 10.1111/j.1364-3703.2011.00783.x
Dodds, P. N., Lawrence, G. J., Catanzariti, A. M., Ayliffe, M. A., and Ellis, J. G. (2004). The Melampsora lini AvrL567 avirulence genes are expressed in haustoria and their products are recognized inside plant cells. Plant Cell 16, 755–768. doi: 10.1105/tpc.020040
Dodds, P. N., Lawrence, G. J., Catanzariti, A. M., Teh, T., Wang, C. I., Ayliffe, M. A., et al. (2006). Direct protein interaction underlies gene-for-gene specificity and coevolution of the flax resistance genes and flax rust avirulence genes. Proc. Natl. Acad. Sci. U.S.A. 103, 8888–8893. doi: 10.1073/pnas.0602577103
Dodds, P. N., and Rathjen, J. P. (2010). Plant immunity: towards an integrated view of plant-pathogen interactions. Nat. Rev. Genet. 11, 539–548. doi: 10.1038/nrg2812
Du, J., Verzaux, E., Chaparro-Garcia, A., Bijsterbosch, G., Keizer, L. P., Zhou, J., et al. (2015). Elicitin recognition confers enhanced resistance to Phytophthora infestans in potato. Nat. Plants 1:15034. doi: 10.1038/nplants.2015.34
Dubin, H., and Brennan, J. P. (2009). Combating Stem and Leaf Rust of Wheat: Historical Perspective, Impacts, and Lessons Learned. International Food Policy Research Institute. IFPRI Discussion Paper No. 00910. 2020 Vision Initiative. Available online at: http://www.ifpri.org/millionsfed.
Duplessis, S., Cuomo, C. A., Lin, Y. C., Aerts, A., Tisserant, E., Veneault-Fourrey, C., et al. (2011). Obligate biotrophy features unraveled by the genomic analysis of rust fungi. Proc. Natl. Acad. Sci. U.S.A. 108, 9166–9171. doi: 10.1073/pnas.1019315108
Dyck, P., Samborski, D., and Anderson, R. (1966). Inheritance of adult-plant leaf rust resistance derived from the common wheat varieties Exchange and Frontana. Can. J. Genet. Cytol. 8, 665–671. doi: 10.1139/g66-082
Ellis, J., Mago, R., Kota, R., Dodds, P., McFadden, H., Lawrence, G., et al. (2007). Wheat rust resistance research at CSIRO in the 21st century - how will we do better? Aust. J. Agric. Res. 58, 507–511. doi: 10.1071/AR06151
Ellis, J. G., Lagudah, E. S., Spielmeyer, W., and Dodds, P. N. (2014). The past, present and future of breeding rust resistant wheat. Front. Plant Sci. 5:641. doi: 10.3389/fpls.2014.00641
FAO (2015). World Food Situation. Available online at: http://www.fao.org/worldfoodsituation/csdb/en/
Figueroa, M., Alderman, S., Garvin, D. F., and Pfender, W. F. (2013). Infection of Brachypodium distachyon by formae speciales of Puccinia graminis: early infection events and host-pathogen incompatibility. PLoS ONE 8:e56857. doi: 10.1371/journal.pone.0056857
Figueroa, M., Castell-Miller, C. V., Li, F., Hulbert, S. H., and Bradeen, J. M. (2015). Pushing the boundaries of resistance: insights from brachypodium-rust interactions. Front. Plant Sci. 6:558. doi: 10.3389/fpls.2015.00558
Flor, H. (1971). Current status of the gene-for-gene concept. Annu. Rev. Phytopathol. 9, 275–296. doi: 10.1146/annurev.py.09.090171.001423
Garnica, D. P., Nemri, A., Upadhyaya, N. M., Rathjen, J. P., and Dodds, P. N. (2014). The ins and outs of rust haustoria. PLoS Pathog. 10:e1004329. doi: 10.1371/journal.ppat.1004329
Garnica, D. P., Upadhyaya, N. M., Dodds, P. N., and Rathjen, J. P. (2013). Strategies for wheat stripe rust pathogenicity identified by transcriptome sequencing. PLoS ONE 8:e67150. doi: 10.1371/journal.pone.0067150
Gates, J. E., and Loegering, W. Q. (1991). Mutation to wider virulence in Puccinia graminis f. sp. tritici: evidence for the existence of loci which allow the fungus to overcome several host stem rust resistance genes simultaneously. Appl. Environ. Microbiol. 57, 2332–2336.
Gordon, S., Priest, H., Des Marais, D., Schackwitz, W., Figueroa, M., Martin, J., et al. (2013). Genome diversity in Brachypodium distachyon: deep sequencing of highly diverse natural accessions. Plant J. 79, 361–374. doi: 10.1111/tpj.12569
Hacquard, S., Joly, D. L., Lin, Y. C., Tisserant, E., Feau, N., Delaruelle, C., et al. (2012). A Comprehensive Analysis of Genes Encoding Small Secreted Proteins Identifies Candidate Effectors in Melampsora larici-populina (Poplar Leaf Rust). Mol. Plant-Microbe Interact. 25, 279–293. doi: 10.1094/MPMI-09-11-0238
Harder, D. (1984). “Developmental ultrastructure of hyphae and spores,” in The Cereal Rusts, Vol. 1, eds W. R. Bushnell and A. P. Roelfs (Orlando, FL: Academic Press, Inc.), 333–373.
Harder, D. E., and Chong, J. (1984). “Structure and physiology of haustoria,” in The Cereal Rusts, Vol. 1, eds W. R. Bushnell and A. P. Roelfs (Orlando, FL: Academic Press, Inc.), 416–460.
Hogenhout, S. A., van Der Hoorn, R. A., Terauchi, R., and Kamoun, S. (2009). Emerging concepts in effector biology of plant-associated organisms. Mol. Plant Microbe Interact. 22, 115–122. doi: 10.1094/MPMI-22-2-0115
Initiative, T. I. B. (2010). Genome sequencing and analysis of the model grass Brachypodium distachyon. Nature 463, 763–768. doi: 10.1038/nature08747
Jia, Y., McAdams, S. A., Bryan, G. T., Hershey, H. P., and Valent, B. (2000). Direct interaction of resistance gene and avirulence gene products confers rice blast resistance. EMBO J. 19, 4004–4014. doi: 10.1093/emboj/19.15.4004
Jin, Y., Rouse, M., and Groth, J. (2014). Population diversity of Puccinia graminis is sustained through sexual cycle on alternate hosts. J. Integr. Agric. 13, 262–264. doi: 10.1016/S2095-3119(13)60647-4
Jin, Y., Szabo, L., Pretorius, Z., Singh, R., Ward, R., and Fetch, T. Jr. (2008). Detection of virulence to resistance gene Sr24 within race TTKS of Puccinia graminis f. sp. tritici. Plant Dis. 92, 923–926. doi: 10.1094/PDIS-92-6-0923
Jones, D. A., Thomas, C. M., Hammond-Kosack, K. E., Balint-Kurti, P. J., and Jones, J. (1994). Isolation of the tomato Cf-9 gene for resistance to Cladosporium fulvum by transposon tagging. Science 266, 789–793. doi: 10.1126/science.7973631
Koeck, M., Hardham, A. R., and Dodds, P. N. (2011). The role of effectors of biotrophic and hemibiotrophic fungi in infection. Cell. Microbiol. 13, 1849–1857. doi: 10.1111/j.1462-5822.2011.01665.x
Krattinger, S. G., Lagudah, E. S., Spielmeyer, W., Singh, R. P., Huerta-Espino, J., McFadden, H., et al. (2009). A putative ABC transporter confers durable resistance to multiple fungal pathogens in wheat. Science 323, 1360–1363. doi: 10.1126/science.1166453
Lawrence, G. J., Dodds, P. N., and Ellis, J. G. (2010). TECHNICAL ADVANCE: transformation of the flax rust fungus, Melampsora lini: selection via silencing of an avirulence gene. Plant J. 61, 364–369. doi: 10.1111/j.1365-313X.2009.04052.x
Leister, R. T., Ausubel, F. M., and Katagiri, F. (1996). Molecular recognition of pathogen attack occurs inside of plant cells in plant disease resistance specified by the Arabidopsis genes RPS2 and RPM1. Proc. Natl. Acad. Sci. U.S.A. 93, 15497–15502. doi: 10.1073/pnas.93.26.15497
Leonard, K. J., and Szabo, L. J. (2005). Pathogen profile. Stem rust of small grains and grasses caused by Puccinia graminis. Mol. Plant Pathol. 6, 489–489. doi: 10.1111/j.1364-3703.2005.00273.x
Link, T. I., Lang, P., Scheffler, B. E., Duke, M. V., Graham, M. A., Cooper, B., et al. (2014). The haustorial transcriptomes of Uromyces appendiculatus and Phakopsora pachyrhizi and their candidate effector families. Mol. Plant Pathol. 15, 379–393. doi: 10.1111/mpp.12099
Luig, N. H. (1978). “Mutation studies in Puccinia graminis tritici,” in Proceedings of the 5th International Wheat Genetics Symposium, Vol. 1, ed S. Ramanujam (New Delhi: Indian Society of Genetics and Plant Breeding), 533–539.
Ma, L.-J., van der Does, H. C., Borkovich, K. A., Coleman, J. J., Daboussi, M.-J., Di Pietro, A., et al. (2010). Comparative genomics reveals mobile pathogenicity chromosomes in Fusarium. Nature 464, 367–373. doi: 10.1038/nature08850
Mago, R., Zhang, P., Vautrin, S., Simkova, H., Bansal, U., Luo, M.-C., et al. (2015). The wheat Sr50 reveals a rich diversity at a cereal disease resistance locus. Nat. Plants. 1:15186. doi: 10.1038/nplants.2015.186
Mayer, K. F., Rogers, J., DoleŽel, J., Pozniak, C., Eversole, K., Feuillet, C., et al. (2014). A chromosome-based draft sequence of the hexaploid bread wheat (Triticum aestivum) genome. Science 345, 1251788. doi: 10.1126/science.1251788
McDonald, B. A. (2014). Using dynamic diversity to achieve durable disease resistance in agricultural ecosystems. Trop. Plant Pathol. 39, 191–196. doi: 10.1590/S1982-56762014000300001
McIntosh, R. A., Wellings, C. R., and Park, R. F. (1995). Wheat Rusts: an Atlas of Resistance Genes. Melbourne: CSIRO Publishing.
Moore, J. W., Herrera-Foessel, S., Lan, C., Schnippenkoetter, W., Ayliffe, M., Huerta-Espino, J., et al. (2015). A recently evolved hexose transporter variant confers resistance to multiple pathogens in wheat. Nat. Genet. 47, 1494–1498. doi: 10.1038/ng.3439
Nemri, A., Saunders, D. G., Anderson, C., Upadhyaya, N. M., Win, J., Lawrence, G. J., et al. (2014). The genome sequence and effector complement of the flax rust pathogen Melampsora lini. Front. Plant Sci. 5:98. doi: 10.3389/fpls.2014.00098
Nirmala, J., Drader, T., Lawrence, P. K., Yin, C., Hulbert, S., Steber, C. M., et al. (2011). Concerted action of two avirulent spore effectors activates Reaction to Puccinia graminis 1 (Rpg1)-mediated cereal stem rust resistance. Proc. Natl. Acad. Sci. U.S.A. 108, 14676–14681. doi: 10.1073/pnas.1111771108
Olivera, P., Newcomb, M., Szabo, L., Rouse, M., Johnson, J., Gale, S., et al. (2015). Phenotypic and genotypic characterization of race TKTTF of Puccinia graminis f. sp. tritici that caused a wheat stem rust epidemic in southern Ethiopia in 2013/14. Phytopathology 105, 917–928. doi: 10.1094/PHYTO-11-14-0302-FI
Panwar, V., McCallum, B., and Bakkeren, G. (2013). Endogenous silencing of Puccinia triticina pathogenicity genes through in pant-expressed sequences leads to the suppression of rust disease. Plant J. 73, 521–532. doi: 10.1111/tpj.12047
Park, R. (2007). Stem rust of wheat in Australia. Crop Pasture Sci. 58, 558–566. doi: 10.1071/AR07117
Park, R. (2008). Breeding cereals for rust resistance in Australia. Plant Pathol. 57, 591–602. doi: 10.1111/j.1365-3059.2008.01836.x
Park, R. F., and Wellings, C. R. (2012). Somatic hybridization in the Uredinales. Annu. Rev. Phytopathol. 50, 219–239. doi: 10.1146/annurev-phyto-072910-095405
Patpour, M., Hovmoller, M., Justesen, A. F., Newcomb, M., Olivera Firpo, P. D., Jin, Y., et al. (2016). Emergence of virulence to SrTmp in the Ug99 race group of wheat stem rust, Puccinia graminis f. sp. tritici, in Africa. Plant Dis. 100, 522. doi: 10.1094/PDIS-06-15-0668-PDN
Periyannan, S., Moore, J., Ayliffe, M., Bansal, U., Wang, X., Huang, L., et al. (2013). The gene Sr33, an ortholog of barley Mla genes, encodes resistance to wheat stem rust race Ug99. Science 341, 786–788. doi: 10.1126/science.1239028
Pretorius, Z. A., Singh, R. P., Wagoire, W. W., and Payne, T. S. (2000). Detection of Virulence to Wheat Stem Rust Resistance Gene Sr31 in Puccinia graminis. f. sp. tritici in Uganda. Plant Dis. 84:203. doi: 10.1094/PDIS.2000.84.2.203B
Ravensdale, M., Nemri, A., Thrall, P. H., Ellis, J. G., and Dodds, P. N. (2011). Co−evolutionary interactions between host resistance and pathogen effector genes in flax rust disease. Mol. Plant Pathol. 12, 93–102. doi: 10.1111/j.1364-3703.2010.00657.x
Risk, J. M., Selter, L. L., Chauhan, H., Krattinger, S. G., Kumlehn, J., Hensel, G., et al. (2013). The wheat Lr34 gene provides resistance against multiple fungal pathogens in barley. Plant Biotech. J. 11, 847–854. doi: 10.1111/pbi.12077
Roelfs, A., and Martens, J. (1988). An International System of Nomenclature for Puccinia graminis f. sp. tritici. Phytopathology 78, 526–533.
Roelfs, A. P. (1978). Estimated Losses Caused by Rust in Small Grain Cereals in the United States, 1918-76. Washington, DC: Dept. of Agriculture, Agricultural Research Service: for sale by the Supt. of Docs., US Govt. Print. Off.
Roelfs, A. P. (1982). Effects of barberry eradication on stem rust in the United States. Plant Dis. 66:177. doi: 10.1094/PD-66-177
Roelfs, A. P., and Groth, J. V. (1988). Puccinia graminis f.sp. tritici, Black Stem Rust of Triticum spp. London: Academic Press.
Rouse, M., Wanyera, R., Njau, P., and Jin, Y. (2011). Sources of resistance to stem rust race Ug99 in spring wheat germplasm. Plant Dis. 95, 762–766. doi: 10.1094/PDIS-12-10-0940
Rouse, M. N., Olson, E. L., Gill, B. S., Pumphrey, M. O., and Jin, Y. (2011). Stem rust resistance in germplasm. Crop Sci. 51, 2074–2078. doi: 10.2135/cropsci2010.12.0719
Rowell, J. B., Olien, C. R., and Wilcoxson, R. D. (1958). Effect of certain environmental conditions on infection of wheat by Puccinia graminis. Phytopathology 48, 751–761.
Saintenac, C., Zhang, W., Salcedo, A., Rouse, M. N., Trick, H. N., Akhunov, E., et al. (2013). Identification of wheat gene Sr35 that confers resistance to Ug99 stem rust race group. Science 341, 783–786. doi: 10.1126/science.1239022
Saunders, D. G., Win, J., Cano, L. M., Szabo, L. J., Kamoun, S., and Raffaele, S. (2012). Using hierarchical clustering of secreted protein families to classify and rank candidate effectors of rust fungi. PLoS ONE 7:e29847. doi: 10.1371/journal.pone.0029847
Singh, R. (1993). Genetic association of gene Bdv1 for tolerance to barley yellow dwarf virus with genes Lr 34 and Yr18 for adult plant resistance to rusts in bread wheat. Plant Dis. 77, 1103–1106. doi: 10.1094/PD-77-1103
Singh, R. P., Hodson, D. P., Huerta-Espino, J., Jin, Y., Bhavani, S., Njau, P., et al. (2011). The emergence of Ug99 races of the stem rust fungus is a threat to world wheat production. Annu. Rev. Phytopathol. 49, 465–481. doi: 10.1146/annurev-phyto-072910-095423
Singh, R. P., Hodson, D. P., Jin, Y., Lagudah, E. S., Ayliffe, M. A., Bhavani, S., et al. (2015). Emergence and spread of new races of wheat stem rust fungus: continued threat to food security and prospects of genetic control. Phytopathology 105, 872–884. doi: 10.1094/PHYTO-01-15-0030-FI
Sohn, K. H., Lei, R., Nemri, A., and Jones, J. D. (2007). The downy mildew effector proteins ATR1 and ATR13 promote disease susceptibility in Arabidopsis thaliana. Plant Cell 19, 4077–4090. doi: 10.1105/tpc.107.054262
Sperschneider, J., Gardiner, D. M., Dodds, P. N., Tini, F., Covarelli, L., Singh, K. B., et al. (2015). EffectorP: predicting fungal effector proteins from secretomes using machine learning. New Phytol. doi: 10.1111/nph.13794. [Epub ahead of print].
Sperschneider, J., Ying, H., Dodds, P. N., Gardiner, D. M., Upadhyaya, N. M., Singh, K. B., et al. (2014). Diversifying selection in the wheat stem rust fungus acts predominantly on pathogen-associated gene families and reveals candidate effectors. Front. Plant Sci. 5:372. doi: 10.3389/fpls.2014.00372
Stakman, E. C., and Harrar, J. G. (1957). Principles of Plant Pathology. New York, NY: Ronald Press.
Staples, R. C., and Macko, V. (2004). “Germination of urediospores and differentiation of infection structures,” in The Cereal Rusts, Vol. 1, eds W. R. Bushell and A. P. Roelfs (Orlando, FL: Academic Press, Inc.), 250–282.
Steffenson, B. J., Zhou, H., Chai, Y., and Grando, S. (2013). “Vulnerability of cultivated and wild barley to African stem rust race TTKSK,” in Advance in Barley Sciences (Dordrecht: Springer), 243–255.
Stokstad, E. (2007). Deadly wheat fungus threatens world's breadbaskets. Science 315, 1786–1787. doi: 10.1126/science.315.5820.1786
Tax, F., and Kemmerling, B. (2012). Receptor-Like Kinases in Plants: From Development to Defense. New York; Berlin; Heidelberg: Springer-Verlag.
Thomas, W. J., Thireault, C. A., Kimbrel, J. A., and Chang, J. H. (2009). Recombineering and stable integration of the Pseudomonas syringae pv. syringae 61 hrp/hrc cluster into the genome of the soil bacterium Pseudomonas fluorescens Pf0−1. Plant J. 60, 919–928. doi: 10.1111/j.1365-313X.2009.03998.x
Upadhyaya, N. M., Garnica, D. P., Karaoglu, H., Sperschneider, J., Nemri, A., Xu, B., et al. (2015). Comparative genomics of Australian isolates of the wheat stem rust pathogen Puccinia graminis f. sp. tritici reveals extensive polymorphism in candidate effector genes. Front. Plant Sci. 5:759. doi: 10.3389/fpls.2014.00759
Upadhyaya, N. M., Mago, R., Staskawicz, B. J., Ayliffe, M. A., Ellis, J. G., and Dodds, P. N. (2014). A bacterial type III secretion assay for delivery of fungal effector proteins into wheat. Mol. Plant-Microbe Interact. 27, 255–264. doi: 10.1094/MPMI-07-13-0187-FI
Ve, T., Williams, S. J., Catanzariti, A.-M., Rafiqi, M., Rahman, M., Ellis, J. G., et al. (2013). Structures of the flax-rust effector AvrM reveal insights into the molecular basis of plant-cell entry and effector-triggered immunity. Proc. Natl. Acad. Sci. U.S.A. 110, 17594–17599. doi: 10.1073/pnas.1307614110
Wang, C.-I. A., Gunèar, G., Forwood, J. K., Teh, T., Catanzariti, A.-M., Lawrence, G. J., et al. (2007). Crystal structures of flax rust avirulence proteins AvrL567-A and-D reveal details of the structural basis for flax disease resistance specificity. Plant Cell 19, 2898–2912. doi: 10.1105/tpc.107.053611
Wang, X., and McCallum, B. (2009). Fusion body formation, germ tube anastomosis, and nuclear migration during the germination of urediniospores of the wheat leaf rust fungus, Puccinia triticina. Phytopathology 99, 1355–1364. doi: 10.1094/PHYTO-99-12-1355
Wang, X., Richards, J., Gross, T., Druka, A., Kleinhofs, A., Steffenson, B., et al. (2013). The Rpg4-mediated resistance to wheat stem rust (Puccinia graminis) in barley (Hordeum vulgare) requires Rpg5, a second NBS-LRR gene, and an actin depolymerization factor. Mol. Plant-Microbe Interact. 26, 407–418. doi: 10.1094/MPMI-06-12-0146-R
Waterhouse, W. L. (1952). “Australian rust studies. IX. Physiological race determinations and surveys of cereal rusts,” in Proceedings of the Linnean Society of New South Wales (New South Wales), 209–258.
Watson, I. (1981). “Wheat and its rust parasites in Australia,” Wheat Science-Today and Tomorrow eds L. T. Evans and W. J. Peacock (Cambridge, UK: Cambridge University Press), 129–147.
Watson, I. A. (1958). The Present Status of Breeding Disease Resistant Wheats in Australia. NSW Department of Agriculture.
Wheat (2014). “Wheat: the vital grain of civilization and food security,” in CGIAR Research Program on Wheat (Mexico).
Wulff, B. B. H., Horvath, D. M., and Ward, E. R. (2011). Improving immunity in crops: new tactics in an old game. Curr. Opin. Plant Biol. 14, 468–476. doi: 10.1016/j.pbi.2011.04.002
Yin, C., Downey, S. I., Klages-Mundt, N. L., Ramachandran, S., Chen, X., Szabo, L. J., et al. (2015). Identification of promising host-induced silencing targets among genes preferentially transcribed in haustoria of Puccinia. BMC Genomics 16:579. doi: 10.1186/s12864-015-1791-y
Yin, C., and Hulbert, S. (2011). Prospects for functional analysis of effectors from cereal rust fungi. Euphytica 179, 57–67. doi: 10.1007/s10681-010-0285-x
Yirgou, D., and Caldwell, R. M. (1968). Stomatal penetration of wheat seedlings by stem and leaf rusts in relation to effects of carbon dioxide light and stomatal aperture. Phytopathology 58, 500–507.
Yoshida, K., Saitoh, H., Fujisawa, S., Kanzaki, H., Matsumura, H., Yoshida, K., et al. (2009). Association genetics reveals three novel avirulence genes from the rice blast fungal pathogen Magnaporthe oryzae. Plant Cell 21, 1573–1591. doi: 10.1105/tpc.109.066324
Zambino, P. J., Kubelik, A. R., and Szabo, L. (2000). Gene action and linkage of avirulence genes to DNA markers in the rust fungus Puccinia graminis. Phytopathology 90, 819–826. doi: 10.1094/PHYTO.2000.90.8.819
Keywords: Puccinia, effectors, resistance, stem rust, virulence, avirulence, wheat, barley
Citation: Figueroa M, Upadhyaya NM, Sperschneider J, Park RF, Szabo LJ, Steffenson B, Ellis JG and Dodds PN (2016) Changing the Game: Using Integrative Genomics to Probe Virulence Mechanisms of the Stem Rust Pathogen Puccinia graminis f. sp. tritici. Front. Plant Sci. 7:205. doi: 10.3389/fpls.2016.00205
Received: 25 November 2015; Accepted: 06 February 2016;
Published: 24 February 2016.
Edited by:
Guus Bakkeren, Agriculture and Agri-Food Canada, CanadaReviewed by:
Mark Gijzen, Agriculture and Agri-Food Canada, CanadaBruce A. McDonald, ETH Zürich, Switzerland
Copyright © 2016 Figueroa, Upadhyaya, Sperschneider, Park, Szabo, Steffenson, Ellis and Dodds. This is an open-access article distributed under the terms of the Creative Commons Attribution License (CC BY). The use, distribution or reproduction in other forums is permitted, provided the original author(s) or licensor are credited and that the original publication in this journal is cited, in accordance with accepted academic practice. No use, distribution or reproduction is permitted which does not comply with these terms.
*Correspondence: cGV0ZXIuZG9kZHNAY3Npcm8uYXU=