- 1Department of Natural Products, Plant Biology and Soil Science, Laboratory of Plant Physiology, Faculty of Pharmacy, University of Barcelona, Barcelona, Spain
- 2Max-Planck-Institut für Molekulare Pflanzenphysiologie, Potsdam-Golm, Germany
- 3Department of Agricultural Sciences, Biotechnology and Food Science, Cyprus University of Technology, Limassol, Cyprus
Early and more recent studies have suggested that some polyamines (PAs), and particularly spermine (Spm), exhibit anti-senescence properties in plants. In this work, we have investigated the role of Arabidopsis Polyamine Oxidase 4 (PAO4), encoding a PA back-conversion oxidase, during dark-induced senescence. Two independent PAO4 (pao4-1 and pao4-2) loss-of-function mutants have been found that accumulate 10-fold higher Spm, and this associated with delayed entry into senescence under dark conditions. Mechanisms underlying pao4 delayed senescence have been studied using global metabolic profiling by GC-TOF/MS. pao4 mutants exhibit constitutively higher levels of important metabolites involved in redox regulation, central metabolism and signaling that support a priming status against oxidative stress. During senescence, interactions between PAs and oxidative, sugar and nitrogen metabolism have been detected that additively contribute to delayed entry into senescence. Our results indicate the occurrence of metabolic interactions between PAs, particularly Spm, with cell oxidative balance and transport/biosynthesis of amino acids as a strategy to cope with oxidative damage produced during senescence.
Introduction
Polyamines (PAs) putrescine (Put), spermidine (Spd), and spermine (Spm) are nitrogen-containing compounds of low molecular weight known to participate in stress responses (Alcázar et al., 2010; Takahashi and Kakehi, 2010; Minocha et al., 2014; Tiburcio et al., 2014). The polycationic nature of PAs enables their participation in the modulation of cell ion balance as well as in the interaction with negatively charged molecules such as membrane lipids, proteins, and nucleic acids (Schuber, 1989; Cai et al., 2014). Protection of plant cell membranes by PAs has been documented and this might underlie some of the anti-senescence properties reported (He et al., 2002; Liu et al., 2007; Del Duca et al., 2014). However, PAs cannot only be considered as mere polycations stabilizing macromolecules. Evidence indicate that PAs have intrinsic properties and some act as signaling molecules (Minocha et al., 2014; Moschou and Roubelakis-Angelakis, 2014; Tiburcio et al., 2014). Some of the reported anti-senescent effects of PAs have been associated with their ability to act as free radical scavengers and inhibitors of lipid peroxidation (Stoynova et al., 1999; Navakoudis et al., 2007; Yaakoubi et al., 2014). Therefore, the mechanisms of action of PAs seem multiple and additive. As such, the use of omic approaches might be useful for unraveling PA mechanistic processes, and to integrate PAs in the context of global metabolic networks.
Polyamine levels mostly depend on the balance between PA biosynthesis and catabolism. PA catabolism is mediated by two types of amine oxidases: copper-containing amine oxidases (CuAO) and FAD-containing PA oxidases (PAO) (Cona et al., 2006; Angelini et al., 2010). Spd, Spm, and thermospermine (tSpm) are preferential substrates of PAO activity (Takahashi et al., 2010; Fincato et al., 2011; Tavladoraki et al., 2012). PAOs are classified depending on whether they terminally oxidize PAs or catalyze their back-conversion (Angelini et al., 2010; Moschou et al., 2012). PAOs catalyzing PA back-conversion oxidize the carbon at the exo side of the N4 of Spd and Spm, producing Put and Spd, respectively. Arabidopsis thaliana (thereafter referred to as Arabidopsis), carries five genes coding for PAOs (AtPAO1–5; Takahashi et al., 2010; Fincato et al., 2011). Tissue- and organ-specific expression studies of AtPAO gene family members have shown some overlapping patterns but also contrasted differences. This, together with their different substrate specificity, suggests a functional evolutionary diversification of the AtPAO gene members (Takahashi et al., 2010). The different subcellular localization of AtPAO proteins may further support this view. AtPAO2–4 are peroxisomal proteins, whereas AtPAO1 and AtPAO5 are predicted to be cytosolic (Takahashi et al., 2010; Fincato et al., 2011, 2012; Kim et al., 2014).
Oxidation of PAs by amine oxidases not only contributes to the regulation of PA homeostasis but also generates products linked to different biological functions (Angelini et al., 2010; Tiburcio et al., 2014). PAs are metabolically linked to reactive oxygen species (ROS) through the production of H2O2 via PA catabolism (Moschou et al., 2008; Takahashi et al., 2010; Fincato et al., 2011; Ono et al., 2012). Indeed, H2O2 generated by amine oxidase activity has been shown to contribute to stomatal opening (An et al., 2008), trigger programmed cell death (PCD; Tisi et al., 2011) and γ-aminobutyric acid (GABA) accumulation (Bhatnagar et al., 2002; Mohapatra et al., 2010), which is thought to participate in stress signaling (Bouché et al., 2003; Shelp et al., 2012).
Peroxisomes constitute a very important source of ROS and reactive nitrogen species (RNS). Current data suggest a link between PAs and ROS/RNS in stress signaling (Molassiotis and Fotopoulos, 2011; Filippou et al., 2013; Tanou et al., 2014). However, the relationship between PAs, ROS, and RNS, and their integrated effects in plant physiology are not completely established.
PAO4 exhibits high affinity for Spm oxidation, and transforms via back-conversion Spm into Spd, but not Spd into Put (Kamada-Nobusada et al., 2008; Takahashi et al., 2010; Fincato et al., 2011). Previously, Arabidopsis pao4 loss-of-function mutants were found to display high Spm and low Spd levels in roots (Kamada-Nobusada et al., 2008). From a signaling perspective, Spm can modify the expression of several genes encoding redox components (Kamada-Nobusada et al., 2008; Mitsuya et al., 2009). Blockage of Spm oxidation by exogenous inhibitors suppressed this transcriptional response, thus suggesting that H2O2 derived from Spm oxidation underlies this response (Mitsuya et al., 2009). Even though a potential signaling role has been recognized for Spm through transcriptional approaches, global metabolite profiling in engineered genotypes in which Spm levels are endogenously affected are, to our knowledge, not yet reported. Such studies might provide clearer associations between genotypes and stress-tolerance phenotypes, as well as a better integration of PAs in the context of global metabolic networks (Bitrián et al., 2012).
In this work, we have studied the involvement of AtPAO4 in Arabidopsis during dark-induced senescence, through the phenotypic analysis of two independent pao4 loss-of-function mutant alleles (pao4-1 and pao4-2). We demonstrate that pao4 mutation leads to delayed dark-induced senescence. Global metabolic profiling of pao4 mutants and wild-type plants was carried out to investigate mechanisms linked to primary metabolism that underlie the anti-senescent properties. We found that pao4 mutation promotes the accumulation of hub metabolites in central metabolism and phytohormone biosynthesis, which are known to protect plants against abiotic stress. We also found interactions between PAs and oxidative, sugar, lipid, and nitrogen metabolism. Our results indicate that Spm accumulation modifies the metabolic profile of Arabidopsis plants, thus delaying dark-induced senescence.
Materials and Methods
Plant Material and Growth Conditions
Arabidopsis thaliana accession Columbia-0 (Col-0) was used as wild type (WT) in this study. Seeds were stratified for 3 days in the dark at 4°C and sown in pots containing a mixture of soil and vermiculite (1:1 [v/v]), irrigated with water and Hoagland-based mineral solution and grown at 21°C under long-day photoperiod (16 h of white fluorescent light, photon flux of 70–90 mmol m-2 s-1). Dark-induced senescence was carried out on adult plants. Fully expanded leaves from 4-week-old plants were used for all analyses. Dark-induced senescence was established essentially as described (Fotopoulos and Kanellis, 2013). In brief, leaves were floated on water in 25 mm-diameter Petri dishes and incubated in the dark at ambient temperature for a period of 4 days.
Isolation of pao4 Mutants and Gene Expression Analyses
Total RNA was isolated from 4-week-old Arabidopsis leaves using TRIzol (Invitrogen). Total RNA was treated with DNase I (RNase-free; Promega USA) and reverse-transcribed using the SUPERSCRIPT First-Strand Synthesis kit (Invitrogen) following manufacturer’s instructions. PCR from equal amounts of cDNA was performed using AtPAO4-specific primers and TaKaRa Ex TaqTM. Amplification of the Arabidopsis Actin 2 gene (AT3G18780.2) (forward primer, 5′-TCACCACAACAGCAGAGCGGGA -3′and reverse primer, 5′-GAAGATGCCCAGAAGTCT -3′) was used for normalization. The PCR conditions were as follows: 96°C 5 min, followed by 35 cycles (5 s at 96°C, 10 s at 64°C, and 40 s at 72°C). PCR products were separated on a 1.0% agarose gel. The analysis was repeated three times with identical results.
The AtPAO4 mutants [AtPAO4 SALK_109229 (Kamada-Nobusada et al., 2008), pao4-2 in this study; AtPAO4 SALK_133599 (Liu et al., 2014), named pao4-1] were obtained from SALK. The position of the T-DNA insertion in SALK_109229 was confirmed by PCR using a combination of AtPAO4 specific gene primers (forward, 5′- GGTGGTCATGGTCTAATGGTG-3′and reverse, 5′- GAGAGGCACAGTTGCAGTTTC-3′) and T-DNA primer (SALK-LB 5′-TTTGGGTGATGGTTCACGTAGTGGG-3′). For SALK_133599 we used SALK-LB in combination with AtPAO4 specific primers, forward 5′- TTCCGATAAGCTTCGTCGTTG -3′ and reverse 5′- TGGAGTCATCCCCGCTAGTTC -3′.
Polyamine Analyses
Polyamines were analyzed by high-performance liquid chromatography (HPLC) separation of dansyl chloride derivatives. The extraction and determination methods have been previously described (Marcé et al., 1995). The analyses were performed in triplicates from three or more independent experiments.
Pigments Content
Leaf pigments were extracted from 12 mm leaf disks in dimethyl sulfoxide as described by Richardson (Richardson et al., 2002). Chlorophyll concentrations were determined using the equations described by Sims and Gamon (2002).
Protein Extraction
Total protein was extracted with phenol, as previously described (Wang et al., 2006). Protein concentration was determined by Bradford (Bio-Rad), diluted to a final concentration of 20 μg/μl, and stored at -20°C. 20 μg of total protein extracts were separated by SDS-PAGE in 12.5% acrylamide gels. Bands were resolved using Colloidal Comassie Brilliant Blue G-250 stain.
Hydrogen Peroxide and Nitric Oxide Quantification
Hydrogen peroxide was quantified using the KI method, as described by Velikova et al. (2000). Nitrite-derived NO content was measured using the Griess reagent in homogenates prepared with Na-acetate buffer (pH 3.6) as described by Zhou et al. (2005). NO content was calculated by comparison to a standard curve of NaNO2.
Lipid Peroxidation
Lipid peroxidation was determined measuring malondialdehyde (MDA) content resulting from the thiobarbituric acid (TBA) reaction using an extinction coefficient of 155 mM-1cm-1 as described by Hodges et al. (1999).
Metabolite Profiling
Metabolite profiling by GC-time of flight (TOF)-MS was performed as previously described (Lisec et al., 2006; Erban et al., 2007). 110 mg of frozen ground homogenized material from rosette leaves was extracted in 360 μL of methanol including internal standard ([13C6] -sorbitol) at 70°C for 15 min and with 200 μL of chloroform at 37°C for 5 min. The polar fraction was prepared by liquid partitioning with 400 μL of water. An aliquot of 80 μL from the upper polar phase was dried in a Speed Vacuum Concentrator for derivatization by methoxyamination in pyridine (40 mg/mL) and subsequent trimethylsilylation in a final volume of 80 μL. Alkanes were added to pyridine for use as retention index standards. Samples were measured using GC-TOF-MS (LECO Instrumente GmbH, Mönchengladbach, Germany). Chromatograms and mass spectra were processed and evaluated using TagFinder software (Luedemann et al., 2008). Metabolite identification was manually supervised using the mass spectral and retention index collection of the Golm Metabolome Database (Kopka et al., 2005; Hummel et al., 2010). Peak heights of the mass fragments were normalized based on sample fresh weight and internal standard [13C6]-sorbitol.
Metabolic implication of reported altered metabolites in this work, further classification and simplified metabolic maps were made by the use of public database KEGG (Kanehisa and Goto, 1999; Kanehisa et al., 2014) and AraCyc developed by Plant Metabolic Network project (PMN; Mueller et al., 2003; Chae et al., 2012).
Statistical Analyses
Statistical analyses were performed using IBM® SPSS® Statistics V.22. Biochemical and physiological damage measurements were subjected to ANOVA. Significant differences between individual means were determined using Tukey’s HSD (Honestly significant difference) pairwise comparison test at the 5% confidence level. Data from metabolomics were analyzed and heat maps obtained from MeV: MultiExperiment Viewer v.4.9 (Saeed et al., 2003).
Results
Isolation of pao4 Mutants
Two independent AtPAO4 (At1g65840) T-DNA insertion mutants (pao4-1 and pao4-2) were isolated that carried single T-DNA insertions. pao4-2 exhibited no expression of PAO4, consistent with a loss-of-function mutation. Conversely, pao4-1 exhibited residual AtPAO4 expression and thus resulted in a knock-down mutation (Figure 1A). No obvious phenotypical differences were observed between pao4-1, pao4-2, and wild-type genotypes under optimal growth conditions, which is in agreement with previous reports (Kamada-Nobusada et al., 2008; Liu et al., 2014). Both pao4-1 and pao4-2 mutant alleles were used throughout the experiments.
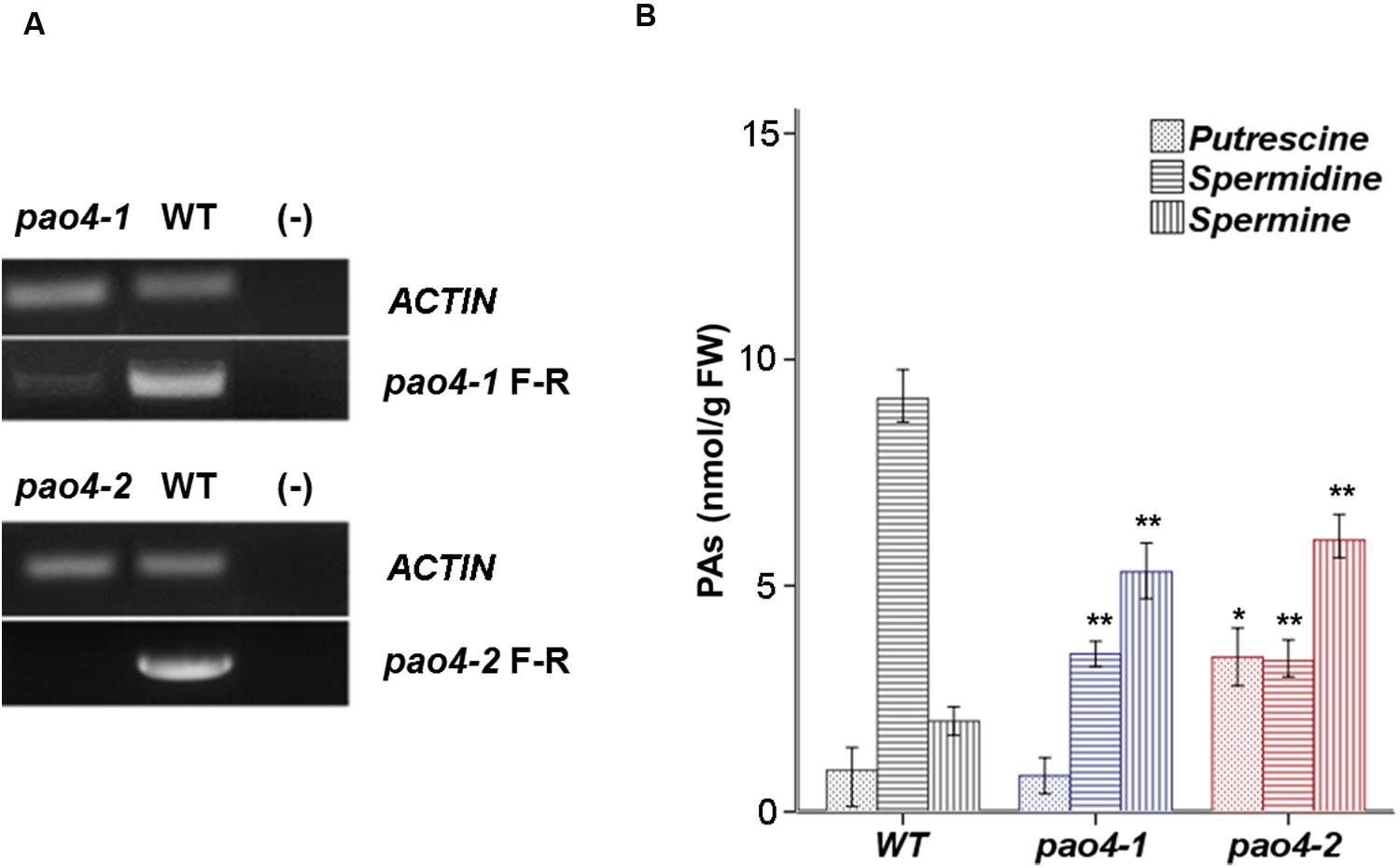
FIGURE 1. AtPAO4 expression and PA levels. (A) PAO4 gene expression in pao4 mutants compared with wild-type. (B) Free levels of Put, Spd, and Spm in 4 week-old pao4 mutants grown under optimal growth conditions. Values represent the mean of at least five biological replicates ±SD. Asterisks indicate values which are significally different from the wild-type, as determined by both one-way ANOVA and Tukey HSD post hoc tests (P < 0.05).
PA Levels in pao4 Mutants
The levels of free Put, Spd, and Spm levels were analyzed in 4 weeks-old pao4-1, pao4-2 and wild-type plants. PA analyses indicated that both pao4 mutants accumulated up to 10-fold higher levels of Spm than the wild-type, consistent with Spm being the preferential substrate of PAO4 activity. Conversely, both pao4-1 and pao4-2 mutants exhibited lower Spd levels than the wild-type (Figure 1B). The levels of Put were only increased in pao4-2 and not in pao4-1, probably as result of the residual PAO4 expression in the latter. We concluded that accumulation of Spm and dampening of Spd levels are common metabolic hallmarks of pao4-1 and pao4-2.
Dark-Induced Senescence in pao4 Mutants
We investigated the differential response of pao4 mutants and wild-type plants to early senescence induced by dark treatment. For this, detached mature leaves from 4 week-old pao4 mutants and wild-type plants grown under optimal conditions were used. No differences in size, senescence status (determined by total chlorophyll and protein levels) or turgor were visible between leaves of the wild-type and pao4 mutant before the dark-induced treatments (data not shown). Interestingly, both pao4-1 and pao4-2 mutants evidenced signs of delayed senescence after 4 days of continuous dark treatment (Figure 2A). Total protein levels were measured to quantify the extent of senescence delay induced by PAO4 mutation. Protein levels were significantly higher in pao4-1 and pao4-2 than the wild type, thus suggesting a lower rate of protein degradation consistent with delayed senescence (Figure 2B). Quantification of chlorophylls in pao4-1 and pao4-2 further supported these observations (Figure 2C), suggesting that pao4 mutation leads to delayed dark-induced senescence.
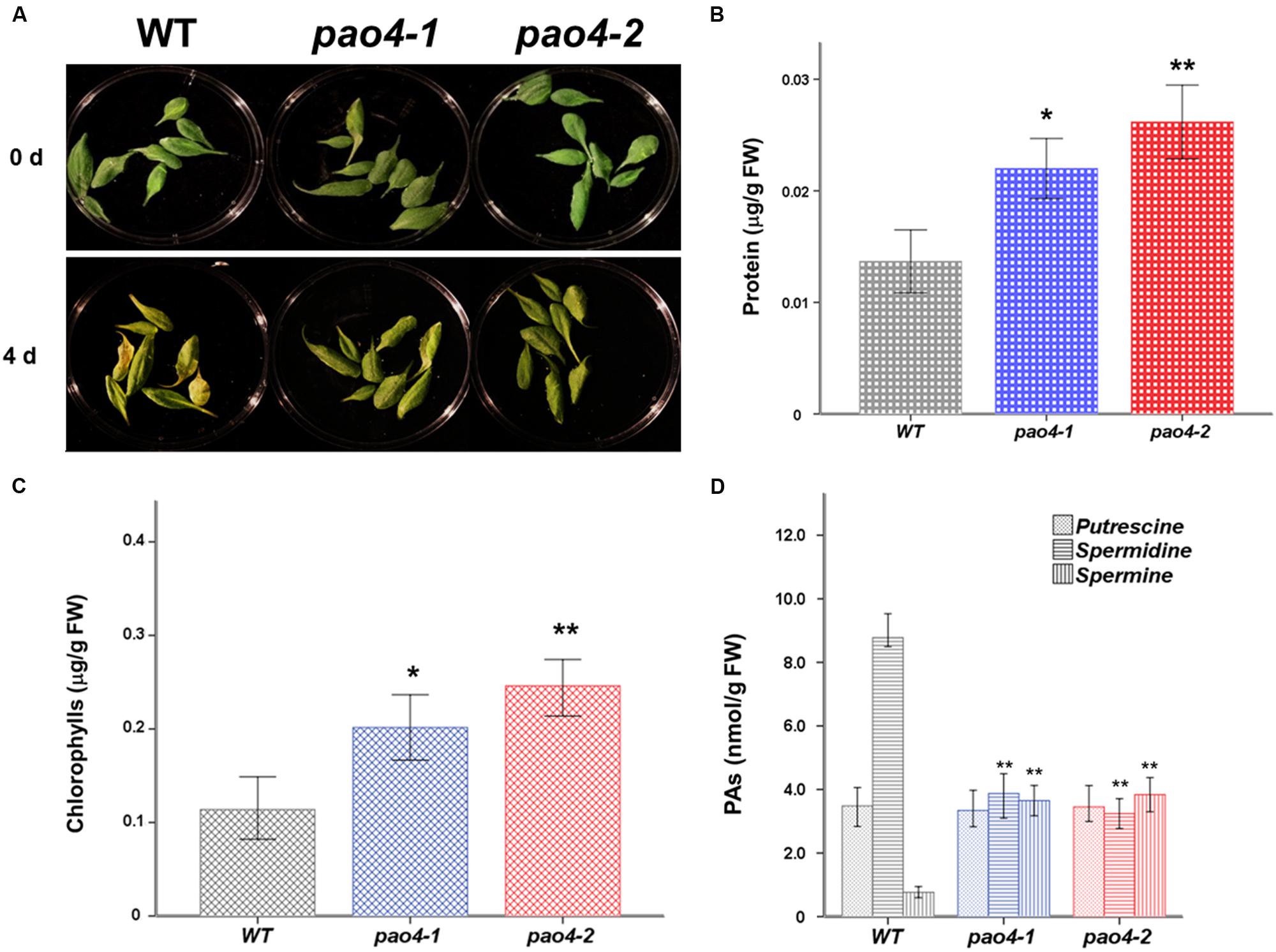
FIGURE 2. Effect of dark-induced senescence in wild-type and pao4 mutants. (A) Macroscopic observation of wild-type and pao4 leaves after dark-induced treatment. (B) Total protein levels in pao4 senescent leaves after dark-induced senescence treatment. (C) Chlorophyll contents in pao4 senescent leaves. (D) Free PAs (Put, Spd, and Spm) levels in senescent pao4 leaves. Each value represents the mean of at least five independent biological replicates ±SD. Asterisks indicate values which are significally different from the wild-type, as determined by both one-way ANOVA and Tukey HSD post hoc tests (P < 0.05).
Polyamine levels were determined during senescence in pao4 mutants and wild-type. Levels remained constant for most PAs throughout the induced senescence, except for Spd levels, which dropped in pao4 from fivefold lower than the wild-type under basal conditions to 10-fold lower than the wild-type after senescence treatment (Figure 2D).
H2O2, MDA, and NO Levels in pao4 Mutants During Dark-Induced Senescence
Reactive oxygen species and RNS are important players of the oxidative and nitrosative response that exhibit contrasted effects on senescence. While ROS generally promote senescence (Khanna-Chopra, 2012), RNS might underlie anti-senescence effects (Niu and Guo, 2012; Liu and Guo, 2013). We measured H2O2 and NO levels in pao4-1, pao4-2 and wild-type plants after dark-induced senescence (Figure 3). Both pao4 mutants exhibited lower H2O2 levels than the wild-type plant after the senescence treatment, thus suggesting the enhancement of the antioxidative machinery in pao4 (Figure 3A). Consistent with these observations, the levels of MDA (a measurement of membrane damage by lipid peroxidation) were significantly lower in pao4 than the wild-type (Figure 3A). Interestingly, the levels of NO exhibited an opposite pattern and accumulated in pao4 compared with the wild-type (Figure 3B). We concluded that ROS production induced by senescence is restricted in pao4 mutants, whereas NO production is stimulated.
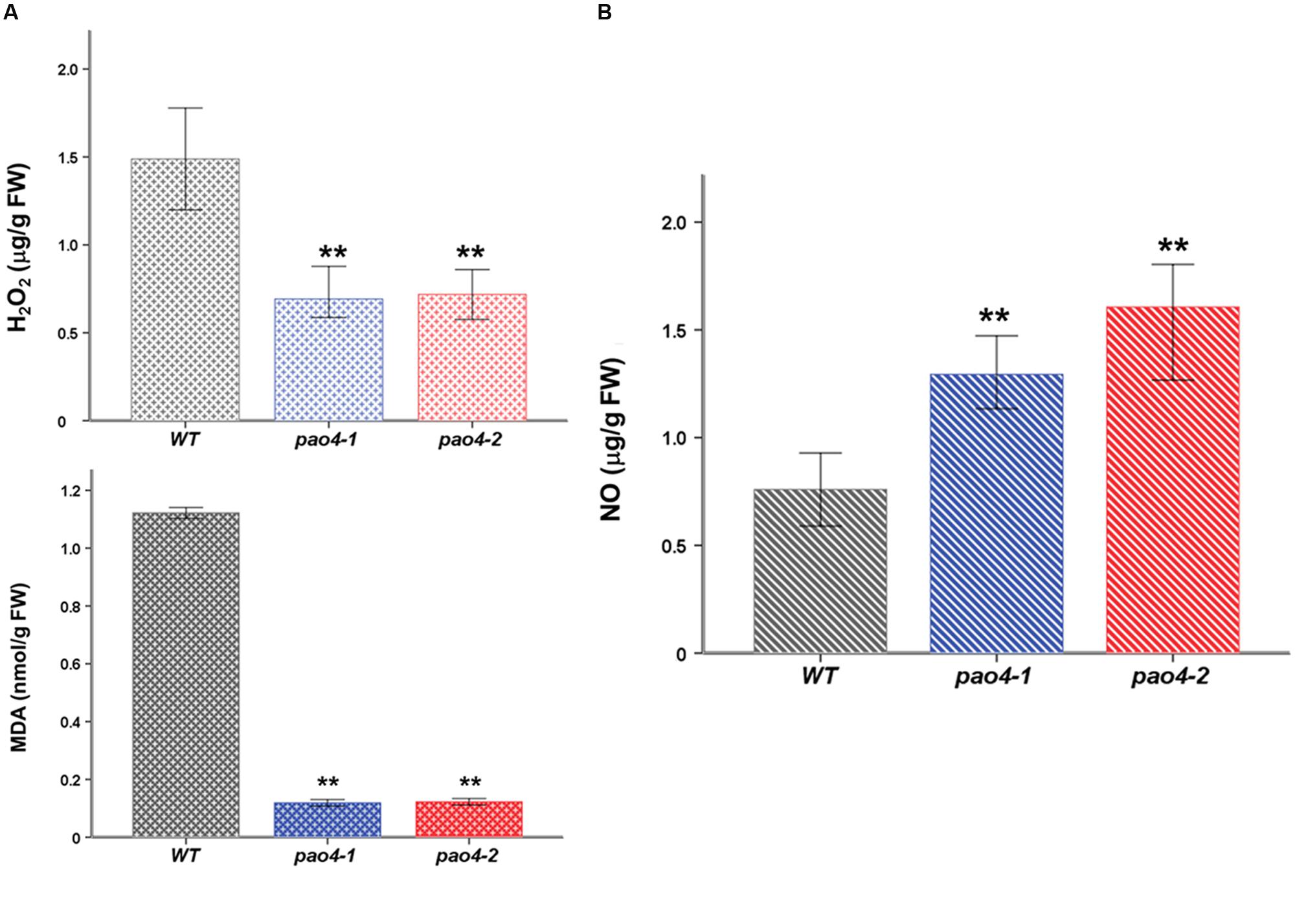
FIGURE 3. H2O2, MDA, and NO levels in pao4 mutants and wild-type after dark-induced senescence treatment. (A) H2O2 and MDA levels (B) NO levels. Free radical levels were determined after dark-induced senescence treatment. Values represent the mean of at least five biological replicates ±SD. Asterisks indicate values which are significally different from those of the corresponding wild-type plants, as determined by both one-way ANOVA and Tukey HSD post hoc tests (P < 0.05).
Metabolomic Profiling of pao4 Mutants Under Basal Conditions
In order to analyze the metabolic consequences of PAO4 loss-of-function on primary metabolism, we performed GC-TOF/MS metabolomic profiling (Erban et al., 2007; Allwood et al., 2011) in 4-week-old pao4 mutants and wild-type plants grown under optimal conditions in the absence of stress, referred to as ‘basal’ conditions. Primary metabolite profiling identified a total of 75 metabolites, 37 of which did not show significant differences respect to the wild-type (Supplementary Table S1). From the remaining 38 metabolites, 28 were increased (Figure 4) and 10 decreased in pao4 compared to the wild-type (Supplementary Table S2). Most down-regulated metabolites could not be classified into metabolic groups, because their chemical structure is unknown (Supplementary Table S2). Up-regulated metabolites could be sorted into four major metabolic categories belonging to oxidative and nitrogen metabolism, sugars and lipids. However, many metabolites were shared between categories (Figure 4A). Increased metabolites in pao4 included sugars (galactose), sugar alcohols (myo-Inositol, erythritol), ethanolamine and many amino acids (Ser; aromatic amino acids Phe and Tyr; precursors of PAs Orn and Met; branched-chain amino acids Ile and Val). Indeed, amino acids represented the largest group of up-regulated metabolites in pao4 under basal conditions (Figure 4A). Other important upregulated metabolites included pyruvate, which is a crucial hub metabolite, GABA, which is suggested to participate in stress responses, and ascorbate/dehydroascorbate (ASC/DHA), which are important metabolites involved in antioxidant defense pathways. Pearson’s correlation analyses indicated the occurrence of strong positive correlations between Spm and up-regulated metabolites, but negative correlations with Spd (P < 0.05; Figure 4B). Based on these analyses, we conclude that pao4 mutants exhibit constitutive accumulation of several amino acids and important stress protection metabolites, and this associates with higher Spm levels and/or Spm/Spd ratios.
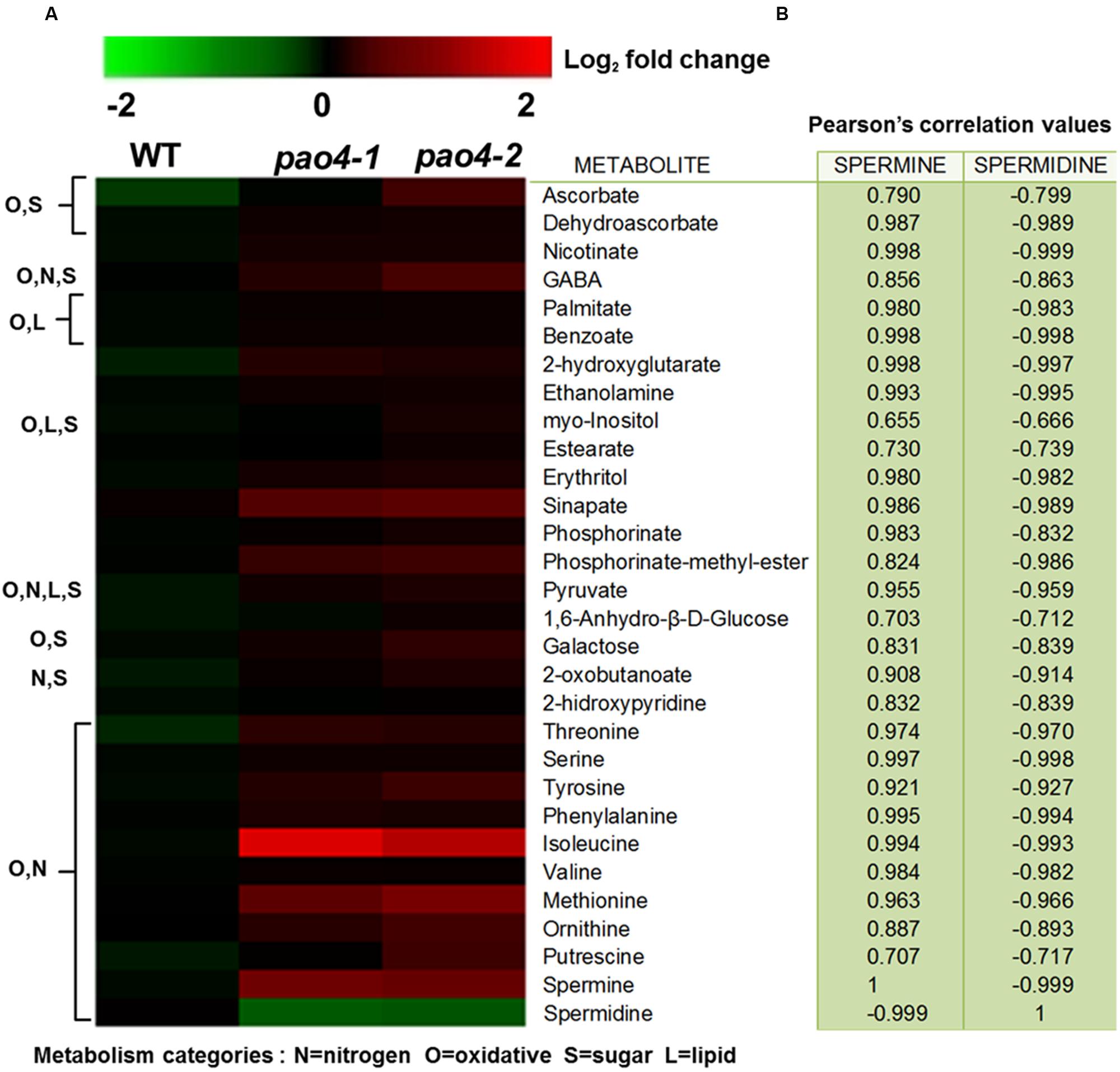
FIGURE 4. Heat-map representation of up-regulated metabolites in pao4 compared with wild-type under control conditions. (A) Metabolite levels were determined in 4 week-old plants and are expressed as log2 relative to wild-type. (B) Pearson’s correlation values (r) related to Spm and Spd. Values were obtained from at least four independent biological experiments (P < 0.05).
Metabolomic Profiling of pao4 Mutants After Dark-Induced Senescence
Metabolomic profiling after dark-induced senescence in pao4 and wild-type leaves identified a total of 103 metabolites (Figure 5A and Supplementary Table S3), 28 of which exhibited significant differences between pao4 and wild-type senescent leaves (Figure 5A). Among these, 13 metabolites were up-regulated and 15 down-regulated in pao4 compared to the wild-type (Figure 5A). 8 of the 13 up-regulated metabolites were already increased in pao4 compared to the wild-type under basal conditions (Figures 4A and 5A). Such constitutively up-regulated metabolites were the PAs Put and Spm, antioxidative metabolites ASC/DHA, myo-Inositol, GABA and the amino acids Thr and Phe. Among up-regulated metabolites exclusively induced after senescence treatment in pao4, and not in the wild-type, we identified sugars (glucose and xylose) and the TCA cycle intermediate 2-oxoglutarate (Figure 5A).
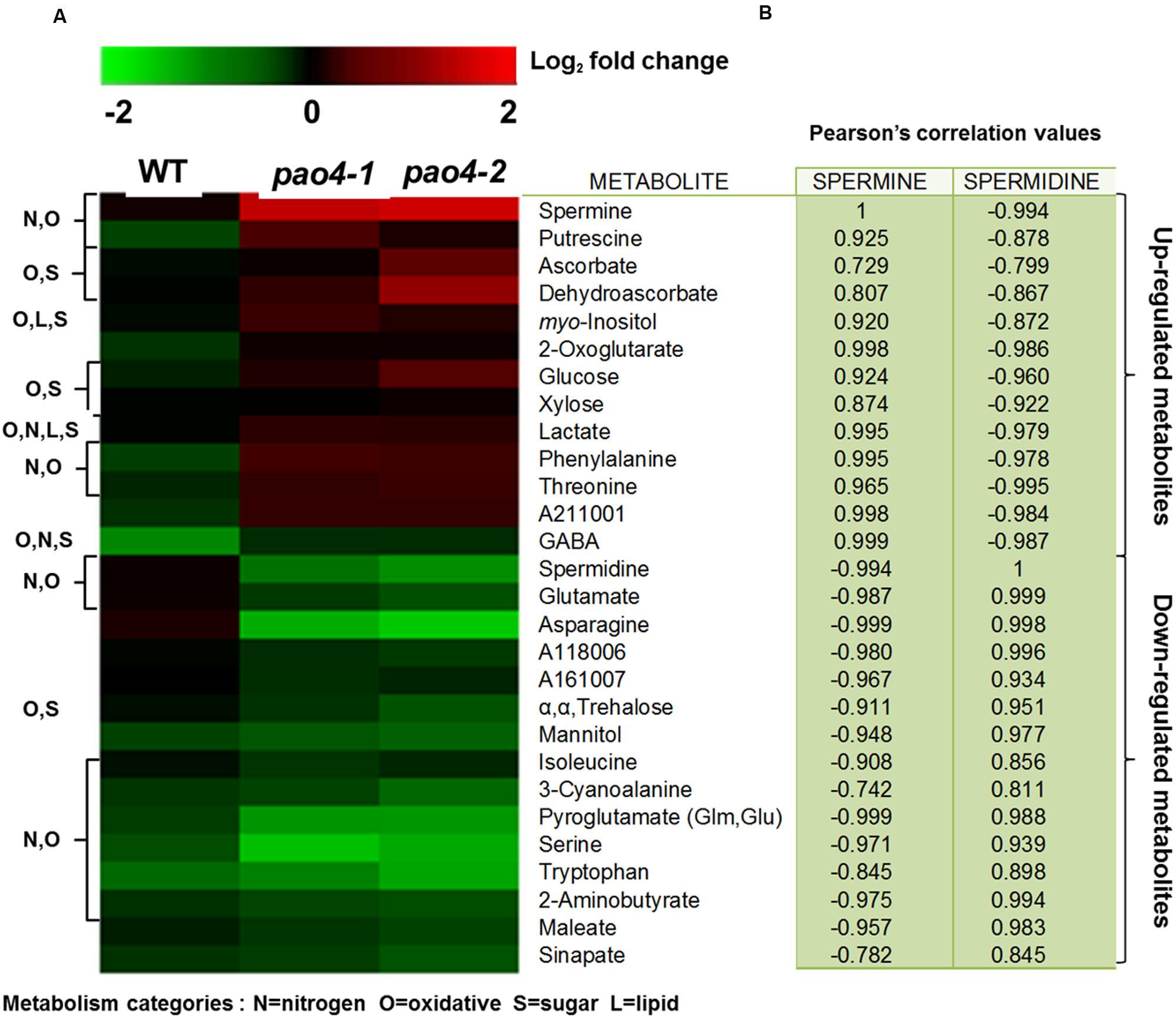
FIGURE 5. Heat map of pao4 mutants altered metabolite pools after dark-induced senescence. (A) Metabolite levels were determined from detached leaves of 4 week-old plants grown as described (see Materials and Methods). Values represent log2-transformed fold-changes relative to the wild-type. The first group (top) represents up-regulated metabolites in pao4 compared with wild-type, and the second group (bottom) down-regulated metabolites in the same comparison. Altered metabolites were detected with MeV tool V.4.9 by rank product statistical test (P < 0.05). (B) Pearson correlation values (r) related to Spm and Spd, values were obtained from at least four independent biological experiments (P < 0.05).
Down-regulated metabolites in senescent pao4 leaves were amino acids involved in senescence signaling such as Glu, pyroglutamate, Trp, Asn, and 3-Cyanoalanine (Figure 5A). The decrease in Glu and Asn is associated with late senescence partly because Asn and 3-Cyanoalanine are products of the cyanide detoxification pathway induced by ethylene biosynthesis (Diaz et al., 2005). Other molecules involved in glucose biosynthesis/degradation, such as α,α, trehalose were down-regulated in pao4.
A strong positive correlation was found between up-regulated metabolites in senescent pao4 leaves and Spm levels, but negative correlations with Spd (P < 0.05; Figure 5B). Conversely, down-regulated metabolites showed an opposite pattern of strong positive correlation with Spd but negative with Spm, suggesting that homeostasis of these PAs may be relevant in the response to senescence (P < 0.05; Figure 5B).
Discussion
The identification of metabolic networks in which PAs are integrated is a necessary step to elucidate potential mechanisms underlying PA-triggered stress protection (Shi and Chan, 2014). Here, we report that loss-of-function mutations in PAO4, a member of the five Arabidopsis AtPAO gene family, leads to delayed dark-induced senescence and this associates with higher Spm and/or lower Spd/Spm ratios.
Accumulation of Spm in pao4 mutants (Figure 1B) is consistent with the reported higher affinity of PAO4 enzyme toward Spm (Kamada-Nobusada et al., 2008; Takahashi et al., 2010; Fincato et al., 2011). Given the previously reported anti-senescence properties of Spm in plants and animals (Pandey et al., 2000; Serafini-Fracassini et al., 2010; Del Duca et al., 2014; Moschou and Roubelakis-Angelakis, 2014), and the high Spm levels in pao4-1 and pao4-2 mutants, current findings suggest that the delayed pao4 senescence may be associated with the endogenous Spm levels. However, because pao4 mutants also exhibit lower Spd levels, it cannot be completely ruled out that the Spd/Spm ratio may modulate this response. In any case, global metabolic analyses in both pao4 mutants indicated that primary metabolism is intricately connected with PA metabolism, and this is differentially regulated in pao4 under senescence conditions. Our results indicate that loss of PAO4 functionality is beneficial to prevent senescence under dark-inductive conditions.
Global metabolite analyses in pao4 mutants under basal conditions (Figure 4) identified amino acids as the largest group of metabolites which were up-regulated, compared with wild-type plants. Up-regulated amino acids included PA precursors (Met and Orn), branched-chain amino acids, aromatic and polar uncharged, which are essential for post-translational modifications. In addition, most altered amino acids were either involved in day/night cycle transitions (Gibon et al., 2006) or adaptation to extended dark conditions (Gibon et al., 2006, 2009). Spm has previously been shown to reprogram the oxidative status of citrus plants exposed to salt stress, and to increase the ASC redox state (Tanou et al., 2014). In this study, the metabolic profile of pao4 suggests the constitutive enhancement of anti-oxidative mechanisms, mainly through the accumulation of ASC/DHA, nicotinate and sinapate, which are essential metabolites in the maintenance of anti-oxidative capacity (Hashida et al., 2010; Wang et al., 2010; Foyer and Noctor, 2011; Gallie, 2012).
pao4-1 and pao4-2 exhibited accumulation of metabolites in central metabolism and signaling hubs under basal conditions. Such metabolites included pyruvate and myo-Inositol, which is involved in sugar and phospholipid signaling (Gillaspy, 2011; Williams et al., 2015). AtPAO4 loss-of-function also led to the up-regulation of nitrogen-mobilization molecules, such as GABA (Bitrián et al., 2012; Shelp et al., 2012). The role of GABA during stress remains unclear. However, GABA has been proposed to act as a signaling molecule that coordinates the C:N balance in challenging environments, such as prolonged dark conditions (Buchanan-Wollaston et al., 2005). GABA also serves as nitrogen-storage molecule during nitro-oxidative stress (Tanou et al., 2012). Overall, the metabolic profile of pao4 mutants under basal conditions is consistent with a prime-like status, in which the antioxidant machinery is pre-activated and GABA accumulates. It is therefore suggested that Spm and/or low Spd/Spm ratio triggers pre-acclimation to stress in Arabidopsis.
Subsequently, mechanisms underlying the pao4 anti-senescence phenotype from a metabolic perspective were investigated. The levels of H2O2 and NO were determined in wild-type and pao4 mutants after dark treatment. Interestingly, delayed senescence in pao4 correlated with significant increases in NO levels (Figure 3B), which is a pattern consistent with previous observations (Niu and Guo, 2012; Liu and Guo, 2013). Conversely, the levels of H2O2 were lower in pao4 than wild-type plants (Figure 3A), which is in agreement with promotion of the ASC/DHA cycle in pao4 (Figure 4A) and supports previous findings in which ROS inhibition leads to delayed senescence in tobacco and wheat (Hui et al., 2012; Fotopoulos and Kanellis, 2013; Tian et al., 2013). NO might be an inductive element of the oxidative response after stress imposition (Linka and Theodoulou, 2013; Corpas and Barroso, 2014). It can be hypothesized that priming by Spm confer a more intense dark-induced stress response involving NO signaling.
Compared with the wild-type, most metabolites altered by dark in pao4 were related to oxidative and nitrogen metabolism (Figure 5). Down-regulated metabolites in dark-treated pao4 were amino acids and compounds involved in their metabolism (Figure 5A). This pattern is consistent with high nitrogen mobilization in pao4 induced by senescence (Soudry et al., 2005). Indeed, interactions have been observed between PA and amino acid metabolism during senescence in Arabidopsis (Mattoo et al., 2010; Watanabe et al., 2013). NO is also known to be involved in the regulation of free amino acid levels during the stress response by induction of the γ-glutamyl cycle for GSH biosynthesis (Innocenti et al., 2007), and through modulation of proteolytic mechanisms such as autophagy or the TOR pathway in Arabidopsis and other species (López-Berges et al., 2010; Tripathi et al., 2013). Some down-regulated amino acids by dark-induced senescence in pao4 have important implications in senescence signaling. As such, Glu influences adaptation to dark periods in Arabidopsis (Gibon et al., 2009). Glu is also a product of glutathione catabolism along with pyroglutamate (Ohkama-Ohtsu et al., 2007, 2008), which is also involved in mitochondrial reassembly during oxidative stress (Obata et al., 2011) and GABA formation (Soudry et al., 2005; Watanabe et al., 2013). Recent evidence also indicates that increases in nitrogen assimilation favors GSH biosynthesis with concomitant decreases in pyroglutamate and Glu levels (Paulose et al., 2013).
The above data suggest the potential modulation of GSH homeostasis by PAO4 activity, which conditions Spm or Spd/Spm ratio. Metabolite profiling suggests the occurrence of a Spm-triggered oxidative response involved in the maintenance of the redox status throughout modulation of amino acid transport and recycling. Trp is a main precursor of the phytohormone indole-3-acetic acid (IAA; Zhao, 2014), and it participates in plant development and dark-induced senescence signaling (Van der Graaff et al., 2006). Asn and 3-cyanoalanine are products of cyanide detoxification pathway (Piotrowski et al., 2001), which is activated after the final biosynthetic reaction of ethylene (Yamagami et al., 2003). Both Asn and 3-cyanoalanine are considered as senescence markers (Van der Graaff et al., 2006; Watanabe et al., 2013). Cross-talk between PAs and hormones such as ethylene and IAA has been reported, but the molecular nature of such interactions remains elusive (Bitrián et al., 2012). Because pao4 mutants display lower levels of 3-cyanoalanine, Asn and Trp, it is suggested that high Spm levels might promote delayed entry into dark-induced senescence through inhibition of ethylene biosynthesis, although this requires further investigation.
Aromatic and branched-chain amino acids have been shown to act as alternative electron donors for mitochondrial respiration during the stress response, in a process whereby the hydrolysis of 2-hydroxyglutarate (2-HG) produces 2-oxoglutarate (2-OG) with concomitant release of electrons donated to ubiquinol via the ETFQO complex (Ishizaki et al., 2005; Araújo et al., 2010, 2011; Obata et al., 2011). Interestingly, Phe, 2-HG, and 2-OG were increased in pao4 mutants compared with wild-type plants, thus suggesting that Spm promotes the alternative electron donor pathway for mitochondrial respiration (Figure 5). In support to this view, an Spm-induced signaling pathway leading to mitochondrial dysfunction has previously been reported during biotic stress in tobacco and Arabidopsis (Takahashi et al., 2004; Mitsuya et al., 2009). Therefore, it seems reasonable that increases in Spm and NO might enhance mitochondrial energy production after dark-induced senescence.
Other molecules involved in glucose biosynthesis/degradation and enhancement of oxidative burst were also identified, such as α,α, Trehalose (O’Hara et al., 2013). This metabolite has emerged as a redox signaling molecule with a proposed role during stress and senescence (Fernandez et al., 2010; Krasensky et al., 2014). Trehalose degradation confers drought tolerance by producing glucose (Van Houtte et al., 2013), a pattern which has also been observed during dark-induced senescence (Buchanan-Wollaston et al., 2005; Gibon et al., 2006), and is consistent with the increase in glucose levels observed in pao4 after dark treatment (Figure 5).
Furthermore, increases in xylose observed in dark-treated pao4 plants suggest activation of the phosphate-pentose pathway, which is reported to be up-regulated in Arabidopsis roots after oxidative stress imposition (Lehmann et al., 2009) as a source of reducing equivalents in peroxisomes for GSH biosynthesis (Corpas et al., 2009). Increased lactate was also found, which is consistent with a link between sugar and pyruvate-related amino acid metabolism.
Overall, we provide a global view of metabolic changes affected by PAO4 mutation in Arabidopsis, which are associated with delayed entry into dark-induced senescence (Figure 6). Current findings suggest that the delayed pao4 senescence may be associated with high Spm levels, reduced ROS production and increased NO levels. Furthermore, our results point to an important role of Spm as a ‘signaling’ metabolite promoting stress protection through metabolic connections involving ASC/GSH redox state modifications, changes in sugar and nitrogen metabolism, cross-talk with ethylene biosynthesis and mitochondrial electron transport chain modulation, all of which are involved in the nitro-oxidative response after stress imposition.
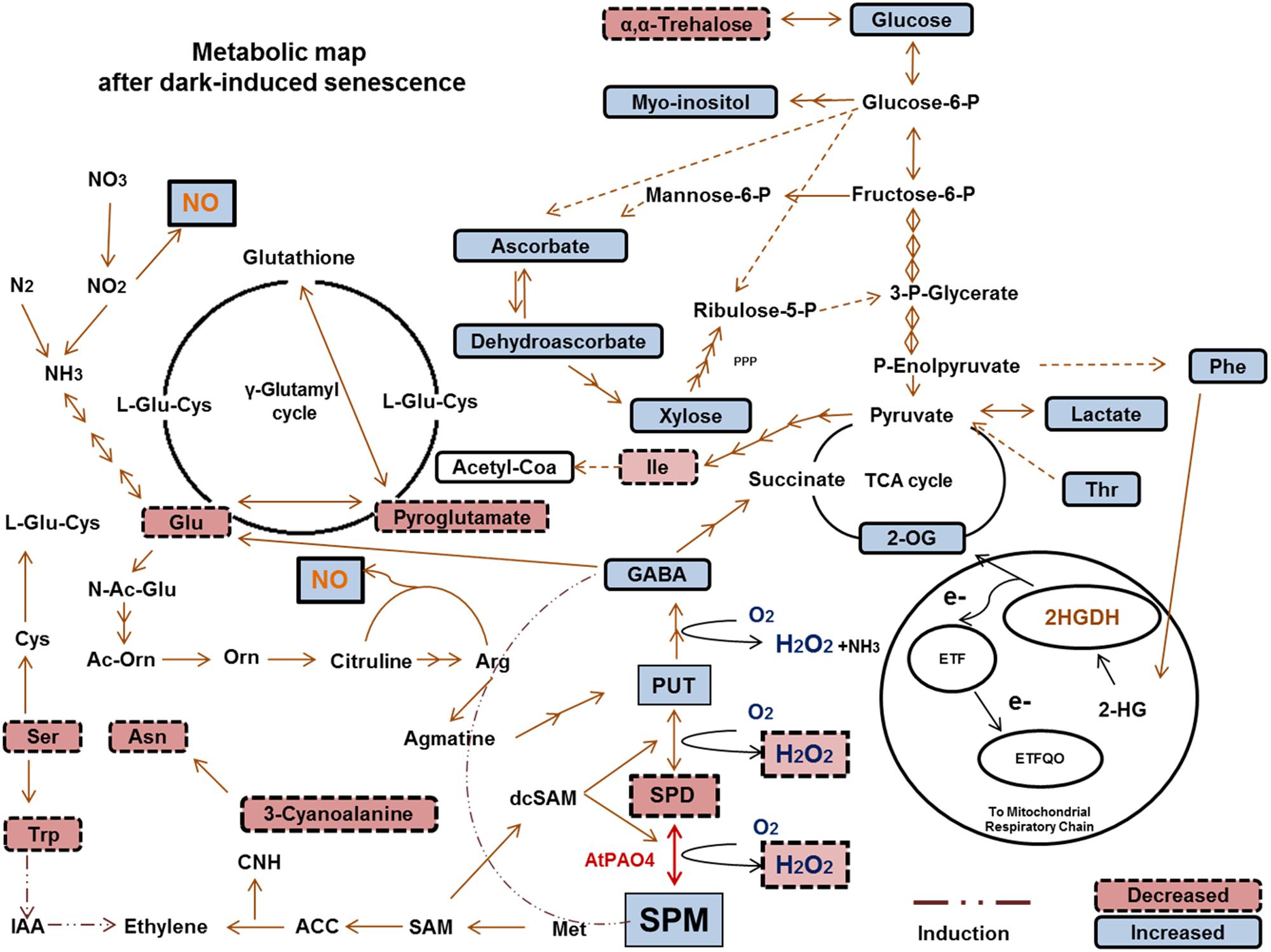
FIGURE 6. Metabolic interactions between PAs and primary metabolism, and the observed increases/decreases in the comparisons between pao4 and wild-type under dark-induced senescence. 2-HG, 2-Hydroxyglutarate; 2HGDH, 2-Hydroxyglutarate Dehydrogenase; ETF, Electron-transfer flavoprotein; ETFQO, Electron-transfer flavoprotein: Ubiquinone oxidoreductase; 2-OG, 2-Oxo-glutarate; SAM, S-Adenosylmethionine; dcSAM, Decarboxilated S-Adenosylmethionine; ACC, Aminocyclopropane Carboxilic Acid; CNH, Hydrogen Cyanide; IAA, Indole-3-Acetic Acid; SA, Salycilic Acid; JA, Jasmonic acid N-Ac-Glu; N-Acetyl-L-Glutamate; Ac-Orn, Acetylornithine; L-Glu-Cys, L-Glutamylcysteine.
Author Contributions
Performed research: MS-M, AE, KA; Analyzed the data; MS-M, AE, JK, VF, RA, AT; Designed research: JK, JB, VF, RA, AT; Wrote the paper: MS-M, VF, RA, AT.
Funding
Research has been financially supported by Spanish Ministerio de Ciencia e Innovación (BIO2011-29683), the Programa de Ayudas al Personal Investigador en Formación (APIF) of the Universitat de Barcelona, the European Cooperation in Science and Technology (COST) action FA0605 (ECOST-STSM-FA0605-200411-008114), and the Generalitat de Catalunya (SGR2009-1060). RA and AT are currently members of the Grup de Recerca Consolidat SGR2014-920.
Supplementary Material
The Supplementary Material for this article can be found online at: http://journal.frontiersin.org/article/10.3389/fpls.2016.00173
Conflict of Interest Statement
The authors declare that the research was conducted in the absence of any commercial or financial relationships that could be construed as a potential conflict of interest.
The reviewer PT and handling Editor declared their shared affiliation, and the handling Editor states that the process nevertheless met the standards of a fair and objective review.
References
Alcázar, R., Altabella, T., Marco, F., Bortolotti, C., Reymond, M., Koncz, C., et al. (2010). Polyamines: molecules with regulatory functions in plant abiotic stress tolerance. Planta 231, 1237–1249. doi: 10.1007/s00425-010-1130-0
Allwood, J. W., De Vos, R. C. H., Moing, A., Deborde, C., Erban, A., Kopka, J., et al. (2011). Plant metabolomics and its potential for systems biology research: background concepts, technology, and methodology. Methods Enzymol. 500, 299–336. doi: 10.1016/B978-0-12-385118-5.00016-5
An, Z., Jing, W., Liu, Y., and Zhang, W. (2008). Hydrogen peroxide generated by copper amine oxidase is involved in abscisic acid-induced stomatal closure in Vicia faba. J. Exp. Bot. 59, 815–825. doi: 10.1093/jxb/erm370
Angelini, R., Cona, A., Federico, R., Fincato, P., Tavladoraki, P., and Tisi, A. (2010). Plant amine oxidases “on the move”: an update. Plant Physiol. Biochem. 48, 560–564. doi: 10.1016/j.plaphy.2010.02.001
Araújo, W. L., Ishizaki, K., Nunes-Nesi, A., Larson, T. R., Tohge, T., Krahnert, I., et al. (2010). Identification of the 2-hydroxyglutarate and isovaleryl-CoA dehydrogenases as alternative electron donors linking lysine catabolism to the electron transport chain of Arabidopsis mitochondria. Plant Cell 22, 1549–1563. doi: 10.1105/tpc.110.075630
Araújo, W. L., Tohge, T., Ishizaki, K., Leaver, C. J., and Fernie, A. R. (2011). Protein degradation - an alternative respiratory substrate for stressed plants. Trends Plant Sci. 16, 489–498. doi: 10.1016/j.tplants.2011.05.008
Bhatnagar, P., Minocha, R., and Minocha, S. C. (2002). Genetic Manipulation of the metabolism of polyamines in poplar cells. the regulation of putrescine catabolism. Plant Physiol. 128, 1455–1469. doi: 10.1104/pp.010792.1
Bitrián, M., Zarza, X., Altabella, T., Tiburcio, A. F., and Alcázar, R. (2012). Polyamines under abiotic stress: metabolic crossroads and hormonal crosstalks in plants. Metabolites 2, 516–528. doi: 10.3390/metabo2030516
Bouché, N., Lacombe, B., and Fromm, H. (2003). GABA signaling: a conserved and ubiquitous mechanism. Trends Cell Biol. 13, 607–610. doi: 10.1016/j.tcb.2003.10.001
Buchanan-Wollaston, V., Page, T., Harrison, E., Breeze, E., Pyung, O. L., Hong, G. N., et al. (2005). Comparative transcriptome analysis reveals significant differences in gene expression and signalling pathways between developmental and dark/starvation-induced senescence in Arabidopsis. Plant J. 42, 567–585. doi: 10.1111/j.1365-313X.2005.02399.x
Cai, G., Sobieszczuk-Nowicka, E., Aloisi, I., Fattorini, L., Serafini-Fracassini, D., and Del Duca, S. (2014). Polyamines are common players in different facets of plant programmed cell death. Amino Acids 47, 27–44. doi: 10.1007/s00726-014-1865-1
Chae, L., Lee, I., Shin, J., and Rhee, S. Y. (2012). Towards understanding how molecular networks evolve in plants. Curr. Opin. Plant Biol. 15, 177–184. doi: 10.1016/j.pbi.2012.01.006
Cona, A., Rea, G., Angelini, R., Federico, R., and Tavladoraki, P. (2006). Functions of amine oxidases in plant development and defence. Trends Plant Sci. 11, 80–88. doi: 10.1016/j.tplants.2005.12.009
Corpas, F. J., and Barroso, J. B. (2014). Peroxynitrite (ONOO-) is endogenously produced in arabidopsis peroxisomes and is overproduced under cadmium stress. Ann. Bot. 113, 87–96. doi: 10.1093/aob/mct260
Corpas, F. J., Hayashi, M., Mano, S., Nishimura, M., and Barroso, J. B. (2009). Peroxisomes are required for in vivo nitric oxide accumulation in the cytosol following salinity stress of Arabidopsis plants. Plant Physiol. 151, 2083–2094. doi: 10.1104/pp.109.146100
Del Duca, S., Serafini-Fracassini, D., and Cai, G. (2014). Senescence and programmed cell death in plants: polyamine action mediated by transglutaminase. Front. Plant Sci. 5:120. doi: 10.3389/fpls.2014.00120
Diaz, C., Purdy, S., Christ, A., Morot-Gaudry, J.-F., Wingler, A., and Masclaux-Daubresse, C. (2005). Characterization of markers to determine the extent and variability of leaf senescence in Arabidopsis. A metabolic profiling approach 1. Plant Physiol. 138, 898–908. doi: 10.1104/pp.105.060764.898
Erban, A., Schauer, N., Fernie, A. R., and Kopka, J. (2007). Nonsupervised construction and application of mass spectral and retention time index libraries from time-of-flight gas chromatography-mass spectrometry metabolite profiles. Methods Mol. Biol. 358, 19–38. doi: 10.1007/978-1-59745-244-1_2
Fernandez, O., Béthencourt, L., Quero, A., Sangwan, R. S., and Clément Christophe, C. (2010). Trehalose and plant stress responses: friend or foe? Trends Plant Sci. 15, 409–417. doi: 10.1016/j.tplants.2010.04.004
Filippou, P., Antoniou, C., and Fotopoulos, V. (2013). The nitric oxide donor sodium nitroprusside regulates polyamine and proline metabolism in leaves of Medicago truncatula plants. Free Radic. Biol. Med. 56, 172–183. doi: 10.1016/j.freeradbiomed.2012.09.037
Fincato, P., Moschou, P. N., Ahou, A., Angelini, R., Roubelakis-Angelakis, K. A., Federico, R., et al. (2012). The members of Arabidopsis thaliana PAO gene family exhibit distinct tissue- and organ-specific expression pattern during seedling growth and flower development. Amino Acids 42, 831–841. doi: 10.1007/s00726-011-0999-7
Fincato, P., Moschou, P. N., Spedaletti, V., Tavazza, R., Angelini, R., Federico, R., et al. (2011). Functional diversity inside the Arabidopsis polyamine oxidase gene family. J. Exp. Bot. 62, 1155–1168. doi: 10.1093/jxb/erq341
Fotopoulos, V., and Kanellis, A. K. (2013). Altered apoplastic ascorbate redox state in tobacco plants via ascorbate oxidase overexpression results in delayed dark-induced senescence in detached leaves. Plant Physiol. Biochem. 73, 154–160. doi: 10.1016/j.plaphy.2013.09.002
Foyer, C. H., and Noctor, G. (2011). Ascorbate and glutathione: the heart of the redox hub. Plant Physiol. 155, 2–18. doi: 10.1104/pp.110.167569
Gallie, D. R. (2012). The role of L-ascorbic acid recycling in responding to environmental stress and in promoting plant growth. J. Exp. Bot. 64, 433–443. doi: 10.1093/jxb/err313
Gibon, Y., Pyl, E. T., Sulpice, R., Lunn, J. E., Höhne, M., Günther, M., et al. (2009). Adjustment of growth, starch turnover, protein content and central metabolism to a decrease of the carbon supply when Arabidopsis is grown in very short photoperiods. Plant Cell Environ. 32, 859–874. doi: 10.1111/j.1365-3040.2009.01965.x
Gibon, Y., Usadel, B., Blaesing, O. E., Kamlage, B., Hoehne, M., Trethewey, R., et al. (2006). Integration of metabolite with transcript and enzyme activity profiling during diurnal cycles in Arabidopsis rosettes. Genome Biol. 7:R76. doi: 10.1186/gb-2006-7-8-r76
Gillaspy, G. E. (2011). The cellular language of myo-Inositol signaling. New Phytol. 192, 823–839. doi: 10.1111/j.1469-8137.2011.03939.x
Hashida, S. N., Itami, T., Takahashi, H., Takahara, K., Nagano, M., Kawai-Yamada, M., et al. (2010). Nicotinate/nicotinamide mononucleotide adenyltransferase-mediated regulation of NAD biosynthesis protects guard cells from reactive oxygen species in ABA-mediated stomatal movement in Arabidopsis. J. Exp. Bot. 61, 3813–3825. doi: 10.1093/jxb/erq190
He, L., Nada, K., Kasukabe, Y., and Tachibana, S. (2002). Enhanced susceptibility of photosynthesis to low-temperature photoinhibition due to interruption of chill-induced increase of S-adenosylmethionine decarboxylase activity in leaves of spinach (Spinacia oleracea L.). Plant Cell Physiol. 43, 196–206. doi: 10.1093/pcp/pcf021
Hodges, D. M., DeLong, J. M., Forney, C. F., and Prange, R. K. (1999). Improving the thiobarbituric acid-reactive-substances assay for estimating lipid peroxidation in plant tissues containing anthocyanin and other interfering compounds. Planta 207, 604–611. doi: 10.1007/s004250050524
Hui, Z., Tian, F. X., Wang, G. K., Wang, G. P., and Wang, W. (2012). The antioxidative defense system is involved in the delayed senescence in a wheat mutant tasg1. Plant Cell Rep. 31, 1073–1084. doi: 10.1007/s00299-012-1226-z
Hummel, J., Strehmel, N., Selbig, J., Walther, D., and Kopka, J. (2010). Decision tree supported substructure prediction of metabolites from GC-MS profiles. Metabolomics 6, 322–333. doi: 10.1007/s11306-010-0198-7
Innocenti, G., Pucciariello, C., Le Gleuher, M., Hopkins, J., De Stefano, M., Delledonne, M., et al. (2007). Glutathione synthesis is regulated by nitric oxide in Medicago truncatula roots. Planta 225, 1597–1602. doi: 10.1007/s00425-006-0461-3
Ishizaki, K., Larson, T. R., Schauer, N., Fernie, A. R., Graham, I. A., and Leaver, C. J. (2005). The critical role of Arabidopsis electron-transfer flavoprotein:ubiquinone oxidoreductase during dark-induced starvation. Plant Cell 17, 2587–2600. doi: 10.1105/tpc.105.035162
Kamada-Nobusada, T., Hayashi, M., Fukazawa, M., Sakakibara, H., and Nishimura, M. (2008). A putative peroxisomal polyamine oxidase, AtPAO4, is involved in polyamine catabolism in Arabidopsis thaliana. Plant Cell Physiol. 49, 1272–1282. doi: 10.1093/pcp/pcn114
Kanehisa, M., and Goto, S. (1999). KEGG: kyoto encyclopedia of genes and genomes. Nucleic Acids Res. 27, 29–34. doi: 10.1093/nar/27.1.29
Kanehisa, M., Goto, S., Sato, Y., Kawashima, M., Furumichi, M., and Tanabe, M. (2014). Data, information, knowledge and principle: back to metabolism in KEGG. Nucleic Acids Res. 42, 199–205. doi: 10.1093/nar/gkt1076
Khanna-Chopra, R. (2012). Leaf senescence and abiotic stresses share reactive oxygen species-mediated chloroplast degradation. Protoplasma 249, 469–481. doi: 10.1007/s00709-011-0308-z
Kim, D. W., Watanabe, K., Murayama, C., Izawa, S., Niitsu, M., Michael, A. J., et al. (2014). Polyamine oxidase 5 regulates Arabidopsis thaliana growth through a thermospermine oxidase activity. Plant Physiol. 168, 690–707. doi: 10.1104/pp.114.242610
Kopka, J., Schauer, N., Krueger, S., Birkemeyer, C., Usadel, B., Bergmüller, E., et al. (2005). GMD@CSB.DB: the golm metabolome database. Bioinformatics 21, 1635–1638. doi: 10.1093/bioinformatics/bti236
Krasensky, J., Broyart, C., Rabanal, F. A., and Jonak, C. (2014). The redox-sensitive chloroplast trehalose-6-phosphate phosphatase AtTPPD regulates salt stress tolerance. Antioxid. Redox Signal. 21, 1–16. doi: 10.1089/ars.2013.5693
Lehmann, M., Schwarzländer, M., Obata, T., Sirikantaramas, S., Burow, M., Olsen, C. E., et al. (2009). The metabolic response of Arabidopsis roots to oxidative stress is distinct from that of heterotrophic cells in culture and highlights a complex relationship between the levels of transcripts, metabolites, and flux. Mol. Plant 2, 390–406. doi: 10.1093/mp/ssn080
Linka, N., and Theodoulou, F. L. (2013). “Peroxisomes and their key role in cellular signaling and metabolism,” in Peroxisomes and Their Key Role in Cellular Signaling and Metabolism, ed. R. Luis (Berlin: Springer), 169–194.
Lisec, J., Schauer, N., Kopka, J., Willmitzer, L., and Fernie, A. R. (2006). Gas chromatography mass spectrometry-based metabolite profiling in plants. Nat. Protoc. 1, 387–396. doi: 10.1038/nprot.2006.59
Liu, F., and Guo, F. Q. (2013). Nitric oxide deficiency accelerates chlorophyll breakdown and stability loss of thylakoid membranes during dark-induced leaf senescence in Arabidopsis. PLoS ONE 8:e56345. doi: 10.1371/journal.pone.0056345
Liu, J. H., Kitashiba, H., Wang, J., Ban, Y., and Moriguchi, T. (2007). Polyamines and their ability to provide environmental stress tolerance to plants. Plant Biotechnol. 24, 117–126. doi: 10.5511/plantbiotechnology.24.117
Liu, T., Dobashi, H., Kim, D. W., Sagor, G. H. M., Niitsu, M., Berberich, T., et al. (2014). Arabidopsis mutant plants with diverse defects in polyamine metabolism show unequal sensitivity to exogenous cadaverine probably based on their spermine content. Physiol. Mol. Biol. Plants 20, 151–159. doi: 10.1007/s12298-014-0227-5
López-Berges, M. S., Rispail, N., Prados-Rosales, R. C., and Di Pietro, A. (2010). A nitrogen response pathway regulates virulence functions in Fusarium oxysporum via the protein kinase TOR and the bZIP protein MeaB. Plant Cell 22, 2459–2475. doi: 10.1105/tpc.110.075937
Luedemann, A., Strassburg, K., Erban, A., and Kopka, J. (2008). TagFinder for the quantitative analysis of gas chromatography–mass spectrometry (GC-MS)-based metabolite profiling experiments. Bioinformatics 24, 732–737. doi: 10.1093/bioinformatics/btn023
Marcé, M., Brown, D. S., Capell, T., Figueras, X., and Tiburcio, A. F. (1995). Rapid high-performance liquid chromatographic method for the quantitation of polyamines as their dansyl derivatives: application to plant and animal tissues. J. Chromatogr. 666, 329–335. doi: 10.1016/0378-4347(94)00586-T
Mattoo, A. K., Minocha, S. C., Minocha, R., and Handa, A. K. (2010). Polyamines and cellular metabolism in plants: transgenic approaches reveal different responses to diamine putrescine versus higher polyamines spermidine and spermine. Amino Acids 38, 405–413. doi: 10.1007/s00726-009-0399-4
Minocha, R., Majumdar, R., and Minocha, S. C. (2014). Polyamines and abiotic stress in plants: a complex relationship. Front. Plant Sci. 5:175. doi: 10.3389/fpls.2014.00175
Mitsuya, Y., Takahashi, Y., Berberich, T., Miyazaki, A., Matsumura, H., Takahashi, H., et al. (2009). Spermine signaling plays a significant role in the defense response of Arabidopsis thaliana to cucumber mosaic virus. J. Plant Physiol. 166, 626–643. doi: 10.1016/j.jplph.2008.08.006
Mohapatra, S., Minocha, R., Long, S., and Minocha, S. C. (2010). Transgenic manipulation of a single polyamine in poplar cells affects the accumulation of all amino acids. Amino Acids 38, 1117–1129. doi: 10.1007/s00726-009-0322-z
Molassiotis, A., and Fotopoulos, V. (2011). Oxidative and nitrosative signaling in plants: two branches in the same tree? Plant Signal. Behav. 6, 210–214. doi: 10.4161/psb.6.2.14878
Moschou, P. N., Paschalidis, K. A., Delis, I. D., Andriopoulou, A. H., Lagiotis, G. D., Yakoumakis, D. I., et al. (2008). Spermidine exodus and oxidation in the apoplast induced by abiotic stress is responsible for H2O2 signatures that direct tolerance responses in tobacco. Plant Cell 20, 1708–1724. doi: 10.1105/tpc.108.059733
Moschou, P. N., and Roubelakis-Angelakis, K. A. (2014). Polyamines and programmed cell death. J. Exp. Bot. 65, 1285–1296. doi: 10.1093/jxb/ert373
Moschou, P. N., Wu, J., Cona, A., Tavladoraki, P., Angelini, R., and Roubelakis-Angelakis, K. A. (2012). The polyamines and their catabolic products are significant players in the turnover of nitrogenous molecules in plants. J. Exp. Bot. 63, 5003–5015. doi: 10.1093/jxb/err313
Mueller, L. A., Zhang, P., and Rhee, S. Y. (2003). AraCyc: a biochemical pathway database for Arabidopsis. Plant Physiol. 132, 453–460. doi: 10.1104/pp.102.017236
Navakoudis, E., Vrentzou, K., and Kotzabasis, K. (2007). A polyamine- and LHCII protease activity-based mechanism regulates the plasticity and adaptation status of the photosynthetic apparatus. Biochim. Biophys. Acta 1767, 261–271. doi: 10.1016/j.bbabio.2007.02.008
Niu, Y. H., and Guo, F. Q. (2012). Nitric oxide regulates dark-induced leaf senescence through EIN2 in Arabidopsis. J. Integr. Plant Biol. 54, 516–525. doi: 10.1111/j.1744-7909.2012.01140.x
Obata, T., Matthes, A., Koszior, S., Lehmann, M., Araújo, W. L., Bock, R., et al. (2011). Alteration of mitochondrial protein complexes in relation to metabolic regulation under short-term oxidative stress in Arabidopsis seedlings. Phytochemistry 72, 1081–1091. doi: 10.1016/j.phytochem.2010.11.003
O’Hara, L. E., Paul, M. J., and Wingler, A. (2013). How do sugars regulate plant growth and development? New insight into the role of trehalose-6-phosphate. Mol. Plant 6, 261–274. doi: 10.1093/mp/sss120
Ohkama-Ohtsu, N., Oikawa, A., Zhao, P., Xiang, C., Saito, K., and Oliver, D. J. (2008). A gamma-glutamyl transpeptidase-independent pathway of glutathione catabolism to glutamate via 5-oxoproline in Arabidopsis. Plant Physiol. 148, 1603–1613. doi: 10.1104/pp.108.125716
Ohkama-Ohtsu, N., Zhao, P., Xiang, C., and Oliver, D. J. (2007). Glutathione conjugates in the vacuole are degraded by gamma-glutamyl transpeptidase GGT3 in Arabidopsis. Plant J. 49, 878–888. doi: 10.1111/j.1365-313X.2006.03005.x
Ono, Y., Kim, D. W., Watanabe, K., Sasaki, A., Niitsu, M., Berberich, T., et al. (2012). Constitutively and highly expressed Oryza sativa polyamine oxidases localize in peroxisomes and catalyze polyamine back conversion. Amino Acids 42, 867–876. doi: 10.1007/s00726-011-1002-3
Pandey, S., Ranade, S. A., Nagar, P. K., and Kumar, N. (2000). Role of polyamines and ethylene as modulators of plant senescence. J. Biosci. 25, 291–299. doi: 10.1007/BF02703938
Paulose, B., Chhikara, S., Coomey, J., Jung, H. I., Vatamaniuk, O., and Dhankher, O. P. (2013). A γ-Glutamyl cyclotransferase protects Arabidopsis plants from heavy metal toxicity by recycling glutamate to maintain glutathione homeostasis. Plant Cell 25, 4580–4595. doi: 10.1105/tpc.113.111815
Piotrowski, M., Schönfelder, S., and Weiler, E. W. (2001). The Arabidopsis thaliana isogene NIT4 and its orthologs in tobacco encode β-Cyano-L-alanine Hydratase/Nitrilase. J. Biol. Chem. 276, 2616–2621. doi: 10.1074/jbc.M007890200
Richardson, A. D., Duigan, S. P., and Berlyn, G. P. (2002). An evaluation of noninvasive methods to estimate foliar chlorophyll content. New Phytol. 153, 185–194. doi: 10.1046/j.0028-646X.2001.00289.x
Saeed, A. I., Sharov, V., White, J., Li, W., Liang, N., Bhagabati, J., et al. (2003). TM4: a free, open-source system for microarray data managment and analysis. BioTechniques 34, 374–378.
Schuber, F. (1989). Influence of polyamines on membrane functions. Biochem. J. 260, 1–10. doi: 10.1042/bj2600001
Serafini-Fracassini, D., Di Sandro, A., and Del Duca, S. (2010). Spermine delays leaf senescence in Lactuca sativa and prevents the decay of chloroplast photosystems. Plant Physiol. Biochem. 48, 602–611. doi: 10.1016/j.plaphy.2010.03.005
Shelp, B. J., Bozzo, G. G., Trobacher, C. P., Zarei, A., Deyman, K. L., and Brikis, C. J. (2012). Hypothesis/review: contribution of putrescine to 4-aminobutyrate (GABA) production in response to abiotic stress. Plant Sci. 193–194, 130–135. doi: 10.1016/j.plantsci.2012.06.001
Shi, H., and Chan, Z. (2014). Improvement of plant abiotic stress tolerance through modulation of the polyamine pathway. J. Integr. Plant Biol. 56, 114–121. doi: 10.1111/jipb.12128
Sims, D. A., and Gamon, J. A. (2002). Relationships between leaf pigment content and spectral reflectance across a wide range of species, leaf structures and developmental stages. Remote Sens. Environ. 81, 337–354. doi: 10.1016/S0034-4257(02)00010-X
Soudry, E., Ulitzur, S., and Gepstein, S. (2005). Accumulation and remobilization of amino acids during senescence of detached and attached leaves: in planta analysis of tryptophan levels by recombinant luminescent bacteria. J. Exp. Bot. 56, 695–702. doi: 10.1093/jxb/eri054
Stoynova, E. Z., Karanov, E. N., and Alexieva, V. (1999). Subcellular aspects of the protective effect of spermine against atrazine in pea plants. Plant Growth Regul. 29, 175–180. doi: 10.1023/A:1006249514986
Takahashi, T., and Kakehi, J.-I. (2010). Polyamines: ubiquitous polycations with unique roles in growth and stress responses. Ann. Bot. 105, 1–6. doi: 10.1093/aob/mcp259
Takahashi, Y., Cong, R., Sagor, G. H. M., Niitsu, M., Berberich, T., and Kusano, T. (2010). Characterization of five polyamine oxidase isoforms in Arabidopsis thaliana. Plant Cell Rep. 29, 955–965. doi: 10.1007/s00299-010-0881-1
Takahashi, Y., Uehara, Y., Berberich, T., Ito, A., Saitoh, H., Miyazaki, A., et al. (2004). A subset of hypersensitive response marker genes, including HSR203J, is the downstream target of a spermine signal transduction pathway in tobacco. Plant J. 40, 586–595. doi: 10.1111/j.1365-313X.2004.02234.x
Tanou, G., Filippou, P., Belghazi, M., Job, D., Diamantidis, G., Fotopoulos, V., et al. (2012). Oxidative and nitrosative-based signaling and associated post-translational modifications orchestrate the acclimation of citrus plants to salinity stress. Plant J. 72, 585–599. doi: 10.1111/j.1365-313X.2012.05100.x
Tanou, G., Ziogas, V., Belghazi, M., Christou, A., Filippou, P., Job, D., et al. (2014). Polyamines reprogram oxidative and nitrosative status and the proteome of citrus plants exposed to salinity stress. Plant Cell Environ. 37, 864–885. doi: 10.1111/pce.12204
Tavladoraki, P., Cona, A., Federico, R., Tempera, G., Viceconte, N., Saccoccio, S., et al. (2012). Polyamine catabolism: target for antiproliferative therapies in animals and stress tolerance strategies in plants. Amino Acids 42, 411–426. doi: 10.1007/s00726-011-1012-1
Tian, F., Gong, J., Zhang, J., Zhang, M., Wang, G., Li, A., et al. (2013). Enhanced stability of thylakoid membrane proteins and antioxidant competence contribute to drought stress resistance in the tasg1 wheat stay-green mutant. J. Exp. Bot. 64, 1509–1520. doi: 10.1093/jxb/ert004
Tiburcio, A. F., Altabella, T., Bitrián, M., and Alcázar, R. (2014). The roles of polyamines during the lifespan of plants: from development to stress. Planta 240, 1–18. doi: 10.1007/s00425-014-2055-9
Tisi, A., Angelini, R., and Cona, A. (2011). Does polyamine catabolism influence root development and xylem differentiation under stress conditions? Plant Signal. Behav. 6, 1844–1847. doi: 10.4161/psb.6.11.17640
Tripathi, D. N., Chowdhury, R., Trudel, L. J., Tee, A. R., Slack, R. S., Walker, C. L., et al. (2013). Reactive nitrogen species regulate autophagy through ATM-AMPK-TSC2-mediated suppression of mTORC1. Proc. Natl. Acad. Sci. U.S.A. 110:E2950. doi: 10.1073/pnas.1307736110
Van der Graaff, E., Schwacke, R., Schneider, A., Desimone, M., and Kunze, R. (2006). Transcription analysis of Arabidopsis membrane transporters and hormone pathways during developmental and induced leaf senescence. Plant Physiol. 141, 776–792. doi: 10.1104/pp.106.079293.Leaf
Van Houtte, H., Vandesteene, L., López-Galvis, L., Lemmens, L., Kissel, E., Carpentier, S., et al. (2013). Overexpression of the trehalase gene AtTRE1 leads to increased drought stress tolerance in Arabidopsis and is involved in abscisic acid-induced stomatal closure. Plant Physiol. 161, 1158–1171. doi: 10.1104/pp.112.211391
Velikova, V., Yordanov, I., and Edreva, A. (2000). Oxidative stress and some antioxidant systems in acid rain-treated bean plants. Plant Sci. 151, 59–66. doi: 10.1016/S0168-9452(99)00197-1
Wang, W., Vignani, R., Scali, M., and Cresti, M. (2006). A universal and rapid protocol for protein extraction from recalcitrant plant tissues for proteomic analysis. Electrophoresis 27, 2782–2786. doi: 10.1002/elps.200500722
Wang, Z., Xiao, Y., Chen, W., Tang, K., and Zhang, L. (2010). Increased vitamin C content accompanied by an enhanced recycling pathway confers oxidative stress tolerance in Arabidopsis. J. Integr. Plant Biol. 52, 400–409. doi: 10.1111/j.1744-7909.2010.00921.x
Watanabe, M., Balazadeh, S., Tohge, T., Erban, A., Giavalisco, P., Kopka, J., et al. (2013). Comprehensive dissection of spatiotemporal metabolic shifts in primary, secondary, and lipid metabolism during developmental senescence in Arabidopsis. Plant Physiol. 162, 1290–1310. doi: 10.1104/pp.113.217380
Williams, S. P., Gillaspy, G. E., and Perera, I. Y. (2015). Biosynthesis and possible functions of inositol pyrophosphates in plants. Front. Plant Sci. 6:67. doi: 10.3389/fpls.2015.00067
Yaakoubi, H., Hamdani, S., Bekalé, L., and Carpentier, R. (2014). Protective action of spermine and spermidine against photoinhibition of photosystem i in isolated thylakoid membranes. PLoS ONE 9:e112893. doi: 10.1371/journal.pone.0112893
Yamagami, T., Tsuchisaka, A., Yamada, K., Haddon, W. F., Harden, L. A., and Theologis, A. (2003). Biochemical diversity among the 1-amino-cyclopropane-1-carboxylate synthase isozymes encoded by the Arabidopsis gene family. J. Biol. Chem. 278, 49102–49112. doi: 10.1074/jbc.M308297200
Keywords: Arabidopsis, polyamines, senescence, spermine, oxidative stress, polyamine oxidases
Citation: Sequera-Mutiozabal MI, Erban A, Kopka J, Atanasov KE, Bastida J, Fotopoulos V, Alcázar R and Tiburcio AF (2016) Global Metabolic Profiling of Arabidopsis Polyamine Oxidase 4 (AtPAO4) Loss-of-Function Mutants Exhibiting Delayed Dark-Induced Senescence. Front. Plant Sci. 7:173. doi: 10.3389/fpls.2016.00173
Received: 20 November 2015; Accepted: 01 February 2016;
Published: 18 February 2016.
Edited by:
Riccardo Angelini, Roma Tre University, ItalyReviewed by:
Paraskevi Tavladoraki, Roma Tre University, ItalyOscar A. Ruiz, Instituto de Investigaciones Biotecnológicas-Instituto Tecnológico de Chascomús, Argentina
Copyright © 2016 Sequera-Mutiozabal, Erban, Kopka, Atanasov, Bastida, Fotopoulos, Alcázar and Tiburcio. This is an open-access article distributed under the terms of the Creative Commons Attribution License (CC BY). The use, distribution or reproduction in other forums is permitted, provided the original author(s) or licensor are credited and that the original publication in this journal is cited, in accordance with accepted academic practice. No use, distribution or reproduction is permitted which does not comply with these terms.
*Correspondence: Antonio F. Tiburcio, YWZlcm5hbmRlekB1Yi5lZHU=