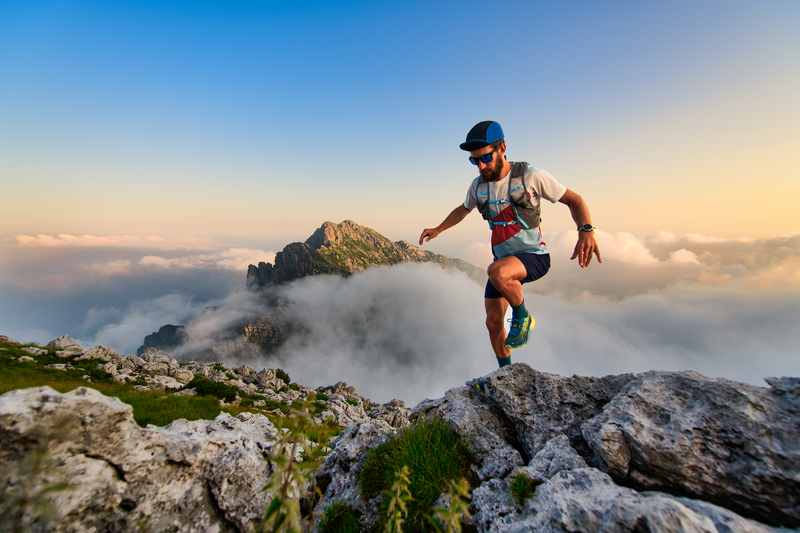
94% of researchers rate our articles as excellent or good
Learn more about the work of our research integrity team to safeguard the quality of each article we publish.
Find out more
REVIEW article
Front. Plant Sci. , 02 February 2016
Sec. Plant Nutrition
Volume 7 - 2016 | https://doi.org/10.3389/fpls.2016.00023
This article is part of the Research Topic Ethylene’s role in plant mineral nutrition View all 13 articles
The phytohormone ethylene is known to mediate a diverse array of signaling processes during abiotic stress in plants. Whereas many reports have demonstrated enhanced ethylene production in metal-exposed plants, the underlying molecular mechanisms are only recently investigated. Increasing evidence supports a role for ethylene in the regulation of plant metal stress responses. Moreover, crosstalk appears to exist between ethylene and the cellular redox balance, nutrients and other phytohormones. This review highlights our current understanding of the key role ethylene plays during responses to metal exposure. Moreover, particular attention is paid to the integration of ethylene within the broad network of plant responses to metal stress.
With the global population exceeding nine billion by 2050, it is of increasing importance to optimize plant growth and ensure food and feed supply. However, plant yield is severely affected by environmental stress factors such as drought, nutrient deficiency, salinity and metal pollution (Mittler, 2006; Dolferus, 2014). Toxic metals and metalloids accumulate in the environment because of industrial applications. Contamination peaks occurred throughout history (e.g. the Roman Empire and Industrial Revolution) and current production rates are still high. In addition, the contribution of metal-contaminated fertilizers, pesticides and sewage sludge to overall metal pollution should not be ignored (Alloway, 2012). Metals such as cadmium (Cd), mercury (Hg) or lead (Pb) are not essential for plants. Therefore, even low concentrations interfere with plant growth and development and cause significant yield losses worldwide. On the other hand, excess levels of essential micronutrients such as copper (Cu), iron (Fe), nickel (Ni) and zinc (Zn) are phytotoxic as well (Cuypers et al., 2009; Hänsch and Mendel, 2009).
Plants are primary producers and therefore constitute an important bridge between the soil elemental composition and the food chain. Non-essential trace elements such as As and Cd opportunistically enter plant tissues via the same transport systems used to take up essential nutrients (Verbruggen et al., 2009; Seth et al., 2012). Excessive accumulation of toxic metals in food and feed crops represents a severe threat to human health (Järup, 2003), indicating the need to remediate metal-contaminated soils. However, recent efforts regarding the use of plants to clean-up soils via phytoextraction are often hampered by metal phytotoxicity (Vangronsveld et al., 2009). Therefore, it is crucial to enhance our current understanding of metal-induced stress responses in plants and provide scientific clues to ameliorate phytoextraction strategies.
A recurring cellular response in metal-exposed plants, independent of the species and exposure time, is an increased generation of reactive oxygen species (ROS) such as superoxide (O), hydrogen peroxide (H2O2) and the hydroxyl radical (•OH; Schützendübel and Polle, 2002; Sharma and Dietz, 2009). Under optimal physiological conditions, ROS are constantly produced as by-products of aerobic metabolism in chloroplasts, mitochondria and peroxisomes. However, their production is tightly controlled and maintained at a low level by the antioxidative defense network of plant cells. This system consists of enzymes neutralizing O and H2O2 such as superoxide dismutase (SOD), catalase (CAT), peroxidases (POD) and peroxiredoxins (Prx), complemented by metabolites such as ascorbate (AsA) and glutathione (GSH). All subcellular compartments are equipped with specific antioxidative enzymes and metabolites maintaining the cellular redox balance within certain limits (Mittler et al., 2004). However, under abiotic stress conditions such as metal exposure, the equilibrium between ROS production and detoxification is disturbed in favor of the former. While redox-active metals such as Cu and Fe are able to directly generate ROS via Fenton and Haber-Weiss reactions, metals without redox properties (e.g. Cd or Hg) only indirectly contribute to ROS production (Schützendübel and Polle, 2002; Verbruggen et al., 2009).
Whereas ROS are closely linked to hormonal signaling networks in a developmental context (Overmyer et al., 2003; Diaz-Vivancos et al., 2013), it is now widely accepted that they also constitute an ambiguous role during stress responses (Dat et al., 2000). Being toxic molecules, ROS are able to oxidatively injure cells (Møller et al., 2007), but they also regulate defense pathways leading to cellular protection and acclimation (Mittler et al., 2004; Petrov and Van Breusegem, 2012). In addition, recent research also suggests a major role for plant hormones interacting with redox signaling to control adaptive responses to environmental stresses (Mittler et al., 2011; Bartoli et al., 2013; Baxter et al., 2014). More specifically, ethylene has been put forward as an important stress hormone under abiotic stress conditions (Dietz et al., 2010). Therefore, the aim of this review is to highlight our current understanding of the role ethylene plays during metal stress in plants. Experimental evidence for the relationship between ethylene and metal exposure is discussed at the level of ethylene biosynthesis as well as signaling, in which different reports support a link between ethylene and metal tolerance or sensitivity. Finally, special attention is paid to the growing body of evidence suggesting a clear integration between ethylene and the broad network of signaling responses activated in metal-exposed plants.
In the following sections, results of different studies are discussed and point toward a role for ethylene during metal stress responses in plants (Table 1). However, when interpreting these results, it is important to take various aspects related to the experimental design into account. First of all, metal-specific properties should be considered. As discussed before, both essential and non-essential metals cause phytotoxic responses, albeit at different exposure levels. Furthermore, experiments can be conducted using massive or environmentally realistic metal concentrations. Under severe stress conditions, ethylene production might be simply increased by tissue damage and necrosis (Lynch and Brown, 1997). Stress severity will affect the activation of specific signal transduction pathways, for example those related to ethylene (Kacperska, 2004). Although Kacperska (2004) proposed that increased ethylene synthesis is a characteristic feature of the alarm situation during severe stress, it was also observed during exposure to mild and environmentally realistic Cd concentrations (Schellingen et al., 2014). Nonetheless, the extent and consequences of augmented ethylene production should always be interpreted with the applied exposure concentrations in mind (Thao et al., 2015).
It is important to discriminate between primary and secondary metal stress-induced events in plants. For example, metal toxicity often leads to nutrient deficiency (Lynch and Brown, 1997; Cuypers et al., 2009), which in its turn is related to alterations in ethylene biosynthesis and signaling (Iqbal et al., 2013a). Furthermore, one of the primary responses of plants to metal stress is the generation of ROS and induction of an oxidative challenge. Redox-active and non-redox-active metals affect the cellular redox state in a different way, which might also influence plant responses related to ethylene as discussed in the section “Interaction between Ethylene and ROS Signaling.” Although some kinetic studies have been conducted (Montero-Palmero et al., 2014a; Schellingen et al., 2014, 2015a,b), more in-depth research is required to decipher the exact order of both primary and secondary events affecting ethylene production under metal stress.
Chelation followed by vacuolar sequestration is a common strategy exploited by plants to maintain low concentrations of free metal(loid)s in the cytosol. Important chelators either contain thiol groups [e.g. metallothioneins, glutathione and phytochelatins (PCs)] or not (e.g. histidine, nicotianamine and organic acids; Seth et al., 2012; Anjum et al., 2015). Especially for GSH, evidence is pointing toward a relationship with ethylene biosynthesis and signaling under metal stress (see section “Crosstalk between Ethylene and GSH”). However, it should be noted that not every metal(loid) is equally connected to this chelating compound (Anjum et al., 2015). Therefore, it is important to consider metal-specific properties when discussing the link between ethylene and GSH.
Finally, different experimental strategies are used to unravel the functional role of ethylene during metal stress. On the one hand, ethylene biosynthesis or signaling can be pharmacologically inhibited. On the other hand, different results can be obtained when studying mutants defective in one or both processes. Furthermore, not all mutations will lead to complete inhibition of ethylene biosynthesis or signaling due to functional redundancy (e.g. different ethylene receptors). Some studies use transformants that overexpress ethylene-related genes, often derived from other plants or even organisms, to study the functional role of ethylene in metal tolerance. Correct data interpretation is therefore only possible when the setup is taken into account (cfr. infra; Thao et al., 2015). The studies summarized in this review clearly point toward an intimate relationship between ethylene and metal stress in plants. However, much work remains to be done to finally determine the mechanistic processes underlying this link and apply this knowledge in field conditions, e.g. during phytoremediation.
In 1901, the Russian plant physiologist Neljubov reported that etiolated pea plants grew horizontally in the laboratory and upright in outside air (Neljubov, 1901). He attributed this abnormal growth response to ethylene in illuminating gas and is therefore credited with its discovery as biologically active compound (Bleecker and Kende, 2000). It took 33 more years to provide chemical proof that plants indeed synthesize this volatile molecule themselves (Gane, 1934), providing an important indication to investigate the function of ethylene as endogenous signaling molecule. Currently, this simple two-carbon atom molecule (C2H4) is “all around” and known to be involved in almost all developmental and physiological processes in plants (De Martinis et al., 2015). It triggers senescence, influences growth, leads to various morphogenetic effects and—important within the scope of this review—acts as “stress hormone” in diverse biotic and abiotic stress conditions (Bleecker and Kende, 2000; Lin et al., 2009; Vandenbussche et al., 2012; Van de Poel et al., 2015).
More than 30 years ago, Yang and co-workers elucidated the ethylene biosynthesis pathway, which involves the consecutive action of three enzymes (Figure 1; Yang and Hoffman, 1984). First, the amino acid methionine is converted to S-adenosyl-methionine (SAM) by SAM synthetase. Using SAM as a substrate, 1-aminocyclopropane-1-carboxylic acid (ACC) is produced by ACC synthase (ACS). This is the rate-limiting step in the ethylene biosynthesis pathway and releases 5′-methylthioadenosine (MTA), which is recycled back to methionine via the so-called “Yang cycle.” In the presence of O2, ACC is degraded by ACC oxidase (ACO) to produce ethylene, CO2 and cyanide (HCN; Figure 1). The latter is detoxified by β-cyanoalanine synthase to prevent toxicity of accumulating HCN at high ethylene biosynthesis rates (Bleecker and Kende, 2000; De Paepe and Van Der Straeten, 2005; Lin et al., 2009).
Figure 1. Ethylene biosynthesis pathway. The amino acid methionine is converted to S-adenosyl-methionine (SAM) by SAM synthetase (1), which requires ATP. Using SAM as a substrate, 1-aminocyclopropane-1-carboxylic acid (ACC) is produced by ACC synthase (ACS) (2). This also releases 5′-methylthioadenosine (MTA), which is recycled back to methionine via the so-called “Yang cycle.” Finally, ACC is oxidized by ACC oxidase (ACO) (3) to produce ethylene, CO2 and cyanide (HCN). In addition, ACC can be converted to its major conjugate 1-malonyl-ACC (MACC) using malonyl-CoA. It can also react with GSH to form γ-glutamyl-ACC (GACC) or with JA to produce jasmonyl-ACC (JA-ACC).
Of the 12 members of the ACS multigene family in Arabidopsis thaliana, eight encode functional ACS enzymes (isoforms 2, 4–9 and 11). While ACS1 is inactive and ACS3 encodes a pseudogene, isoforms 10 and 12 encode aminotransferases (Yamagami et al., 2003; Van de Poel and Van Der Straeten, 2014). The complexity of the ACS family is further enhanced at the structural and functional level by the formation of heterodimers. Although individual members of the gene family display specific developmental and physiological roles, significant combinatorial interplay exists between different isoforms. Various internal as well as external stimuli [developmental cues (e.g. senescence and ripening), light, hormones (e.g. auxin, cytokinin and ethylene), biotic (e.g. pathogens), and abiotic (e.g. heat) stress factors] regulate the production of ethylene at the level of ACS gene expression (Tsuchisaka et al., 2009; Van de Poel and Van Der Straeten, 2014). For example, ACS8 transcript levels are controlled by light and shade as well as the circadian clock (Vandenbussche et al., 2003; Thain et al., 2004). Expression of ACS2 and ACS6 often appears to be regulated by different stresses such as ozone, salinity and hypoxia (Vahala et al., 1998; Arteca and Arteca, 1999; Peng et al., 2005). In addition, ACS enzymes have a highly variable carboxylic end that serves as a regulatory domain responsible for post-transcriptional regulation. This is due to the presence of mitogen-activated protein kinase (MAPK) and/or calcium-dependent protein kinase (CDPK) target sites, with phosphorylation playing an important role in ACS protein stability (Chae and Kieber, 2005; Yoon and Kieber, 2013).
In the final biosynthetic reaction, ACC is converted to ethylene by ACO. When ethylene production rates are high, for example during post-climacteric ripening of tomato fruit (Van de Poel et al., 2012), ACO can also act rate-limiting in ethylene biosynthesis. It is a ferrous-dependent non-heme oxygenase and uses a single electron from AsA to open the ACC ring (Murphy et al., 2014). In A. thaliana, five different ACO genes appear to be expressed in all tissues. However, differential accumulation of specific ACO transcripts is observed during various physiological processes and environmental conditions (De Paepe and Van Der Straeten, 2005; Argueso et al., 2007; Lin et al., 2009; Ruduś et al., 2012). Several ACO genes were shown to be auto-regulated by ethylene (De Paepe et al., 2004) and recently, evidence is suggesting post-transcriptional/translational regulation mechanisms for ACO as well (Dilley et al., 2013; Van de Poel et al., 2014; Van de Poel and Van Der Straeten, 2014).
Instead of being degraded by ACO, ACC can also be converted to its major conjugate 1-malonyl-ACC (MACC) using malonyl-coenzyme-A. Secondly, ACC can react with GSH to form γ-glutamyl-ACC (GACC). Finally, jasmonic acid also forms a conjugate with ACC, producing jasmonyl-ACC (JA-ACC; Figure 1). These conjugates could regulate the pool of available ACC and potentially affect ethylene production. However, the exact molecular and biochemical function of ACC conjugates deserves further investigation, as recent studies report ACC to function as a signal itself (Yoon and Kieber, 2013; Van de Poel and Van Der Straeten, 2014). Increased levels of conjugated ACC were observed in both roots and leaves of Cd-exposed A. thaliana plants (Schellingen et al., 2014; Table 1), supporting a role for ACC conjugation during metal stress. Future research should be conducted to reveal the molecular nature of these conjugates. In particular, GACC might be involved as GSH is known to play a central role in defense to metal stress via its chelating, antioxidant and signaling properties (Jozefczak et al., 2012; Hernández et al., 2015).
Several reports have shown that the effects of metal stress on ethylene production in plants are both metal- and concentration-specific (Abeles et al., 1992; Thao et al., 2015; Table 1). It has been suggested that Cd could be the most phytotoxic inorganic ion able to stimulate ethylene production by plants (Abeles et al., 1992; Arteca and Arteca, 2007). Cadmium-induced increases in ethylene production were observed in Hordeum vulgare (Vassilev et al., 2004), Lycopersicon esculentum (Iakimova et al., 2008), Pisum sativum (Rodríguez-Serrano et al., 2006, 2009), Brassica juncea (Masood et al., 2012; Asgher et al., 2014), Glycine max (Chmielowska-Bąk et al., 2013), A. thaliana (Schellingen et al., 2014) and Triticum aestivum plants (Khan et al., 2015). On the other hand, long-term (16 days) Cd exposure decreased ethylene release in A. thaliana (Carrió-Seguí et al., 2015). Interestingly, a Cd-tolerant H. vulgare genotype showed a larger increase in ethylene emission after 15 days of Cd exposure as compared to a Cd-sensitive genotype (Cao et al., 2014). Up to 6 h after exposure to excess Cu or Zn (25–500 μM), seven-days-old A. thaliana seedlings grown on hydroponics produced more ethylene than unexposed seedlings (Mertens et al., 1999). In contrast, no significant changes in ethylene emission were detected for A. thaliana seedlings in vitro grown in the presence of 25 or 50 μM Cu during 9 days (Lequeux et al., 2010), suggesting an effect of exposure time and/or plant age. Excess Cu (500 μM) did induce increased ethylene production in Helianthus annuus and T. aestivum leaf discs. On the other hand, exposure to 500 μM Cd only enhanced its emission in T. aestivum leaves (Groppa et al., 2003), pointing toward species-specific responses to metal stress. Moreover, different A. thaliana plant parts showed a various induction of ethylene release after exposure to excess Cu or Cd, with the highest production rate observed in inflorescences. This response declined with increasing age of the different plant parts and did not occur in plants exposed to Ni or Zn (Arteca and Arteca, 2007). Nonetheless, Ni and Zn exposure led to higher ethylene release from B. juncea leaves (Khan and Khan, 2014) and aluminum (Al) induced a rapid evolution of ethylene from Lotus japonicus (Sun et al., 2007) and A. thaliana root apices (Sun et al., 2010). Also Fe (Yamauchi and Peng, 1995) and lithium (Li) toxicity (Naranjo et al., 2003) were reported to be linked to stress-induced ethylene production (Table 1).
Although most studies only investigated the effects of metal exposure on ethylene release by plants, the mechanistic basis is becoming increasingly clear (Table 1). For example, Cu induced an increased expression of ACO1 and ACO3 genes in Nicotiana glutinosa (Kim et al., 1998). It has been suggested that upregulation of ACO genes serves as a good ethylene production indicator (Ruduś et al., 2012). Nevertheless, ACC production by ACS covers the rate-limiting step in the ethylene biosynthesis pathway. Sun et al. (2007) have attributed the induction of ethylene evolution from roots of Al-exposed L. japonicus plants to increased ACO activity, but also observed upregulated ACS and ACO gene expression in Medicago truncatula after Al exposure. While Li had a variable effect on ACS expression (Liang et al., 1996), Cu highly increased ACS transcript levels in A. thaliana plants (Weber et al., 2006). Activity of ACS increased in B. juncea plants exposed to Cd (Asgher et al., 2014), Ni or Zn (Khan and Khan, 2014), as well as in Cd-exposed T. aestivum plants (Khan et al., 2015). Transcript levels of ACS and ACO genes were rapidly enhanced in Cu-exposed B. oleracea (Jakubowicz et al., 2010), Al-exposed A. thaliana (Sun et al., 2010), chromium (Cr)-exposed Oryza sativa (Trinh et al., 2014), and Hg-treated O. sativa (Chen et al., 2014) and M. sativa plants (Montero-Palmero et al., 2014a). In addition, Cd was shown to enhance ACS gene expression in G. max (Chmielowska-Bąk et al., 2013) and ACS and/or ACO transcription in H. vulgare (Cao et al., 2014), O. sativa (Trinh et al., 2014) and A. thaliana plants (Herbette et al., 2006; Weber et al., 2006; Schellingen et al., 2014; Table 1). In the latter study, the Cd-induced increase in ACC and ethylene biosynthesis was mainly attributed to upregulated ACS2 and ACS6 expression, as mutants lacking both isoforms did not show enhanced ethylene release when exposed to Cd (Schellingen et al., 2014). These enzymes are both phosphorylated by the MAPKs MPK3 and MPK6, increasing their half-life (Liu and Zhang, 2004; Joo et al., 2008; Lin et al., 2009; Han et al., 2010; Skottke et al., 2011). Furthermore, MPK3 and MPK6 are able to induce ACS2 and ACS6 transcription via the transcription factor WRKY33 (Li et al., 2012). As MAPKs are clearly implicated in metal-induced signaling responses in plants (Opdenakker et al., 2012), they might affect ethylene biosynthesis during metal stress. Finally, whereas most studies focused on ACS or ACO gene expression levels, Dorling et al. (2011) have pointed out the importance of also examining the effects of metal stress on enzyme abundance, activity and post-translational modifications.
The ethylene signaling cascade starts with its perception by a family of membrane-bound receptors that are predominantly localized at the endoplasmic reticulum (ER). Because of its volatile nature, ethylene can freely diffuse throughout the cell from the site of production to the ER. In A. thaliana, five genes encode a high affinity receptor for ethylene: ETHYLENE RESISTANT 1 and 2 (ETR1/2), ETHYLENE RESPONSE SENSOR 1 and 2 (ERS1/2), and ETHYLENE INSENSITIVE 4 (EIN4). Although some functional specificity exists among the different isoforms, they are largely redundant in controlling the ethylene response in plants (Merchante et al., 2013). In the absence of ethylene, its receptors actively suppress the downstream response (Hua and Meyerowitz, 1998). All receptors possess an N-terminal transmembrane domain to bind ethylene, a domain involved in protein-protein interactions between different receptor types and a C-terminal domain to interact with downstream components of the signaling cascade. A functional receptor unit consists of a homo- or heterodimer able to bind ethylene, although associations of higher order can give rise to receptor clusters in the ER membrane (Merchante et al., 2013). REVERSION TO ETHYLENE SENSITIVITY 1 (RTE1) negatively regulates ethylene responses by specifically activating ETR1 (Resnick et al., 2006, 2008). Furthermore, Cu is required for ethylene binding as well as receptor functionality and is delivered to the receptors by the intracellular RESPONSIVE TO ANTAGONIST 1 (RAN1) Cu transporter (Hirayama et al., 1999). Although the role of Cu in ethylene perception is well established, recent results point toward its involvement in ethylene biosynthesis as well. Indeed, A. thaliana plants grown under Cu deficient conditions release less ethylene (Carrió-Seguí et al., 2015).
In the absence of ethylene, the receptors activate a Raf-like protein kinase called CONSTITUTIVE TRIPLE RESPONSE 1 (CTR1), which is a negative regulator of the downstream ethylene signaling cascade (Kieber et al., 1993; Ju et al., 2012). Because of its physical interaction with the ethylene receptors, CTR1 also resides at the ER membrane (Gao et al., 2003). Without ethylene binding to its receptors, CTR1 forms a homodimer and functions as serine/threonine protein kinase to phosphorylate—and thereby inactivate—the downstream molecule ETHYLENE INSENSITIVE 2 (EIN2) (Figure 2; Ju et al., 2012). The EIN2 protein is an essential positive regulator of ethylene signaling. Furthermore, it is the only gene of the ethylene pathway where a loss-of-function mutation leads to complete ethylene insensitivity (Alonso et al., 1999). Similar to CTR1, EIN2 interacts with the ethylene receptors and is therefore localized at the ER membrane (Bisson et al., 2009; Bisson and Groth, 2010).
Figure 2. Ethylene signal transduction pathway. In the absence of ethylene (left part), the ER-membrane embedded receptors such as ETHYLENE RESISTANT 1 (ETR1) activate CONSTITUTIVE TRIPLE RESPONSE 1 (CTR1). This Raf-like protein kinase phosphorylates ETHYLENE INSENSITIVE 2 (EIN2) at the C-terminal domain, which is thereby inactivated. When ethylene is present (right part), its binding to the receptors inactivates CTR1. The C-terminal domain of EIN2 translocates to the nucleus and activates the downstream signaling cascade via ETHYLENE INSENSITIVE 3 (EIN3)/EIN3-LIKE 1 (EIL1) and ETHYLENE RESPONSIVE FACTOR 1 (ERF1), finally affecting the transcription of ethylene responsive genes.
Upon ethylene binding to its receptors, CTR1 is inactivated (Ju et al., 2012; Shakeel et al., 2015). As a result, EIN2 is released from its inhibition by CTR1 and transduces the signal via its C-terminal end that physically moves from the ER membrane to the nucleus to activate the downstream components ETHYLENE INSENSITIVE 3 (EIN3) and EIN3-LIKE 1 (EIL1). These short-lived transcription factors act as positive regulators of the ethylene signaling pathway and activate target genes such as ETHYLENE RESPONSIVE FACTOR 1 (ERF1) that in turn affect the expression of secondary response genes in the ethylene-dependent transcription cascade (Figure 2; Yoo et al., 2009; Merchante et al., 2013). The above-described linear signaling pathway is subject to feedback regulation and turnover of different signaling components at the mRNA and protein level as described elsewhere (Guo and Ecker, 2003; Qiao et al., 2009; Zhao and Guo, 2011; Merchante et al., 2013). As it has not been described yet if and how metal stress affects these regulatory mechanisms, this paves the way for future research in this area.
Several studies support a role for ethylene signaling in response to different metals, mostly related to ERF expression (Table 1). The ERF proteins belong to the APETALA2/ethylene response element binding protein (AP2/EREBP) transcription factor family, which is known to mediate and integrate hormonal and redox signaling pathways during abiotic stress (Dietz et al., 2010). Roots of A. thaliana plants exposed to 50 μM Cd for 2 h showed increased expression levels of ERF1, ERF2 and ERF5, while only ERF1 expression was induced when Cu (10 μM) was applied (Weber et al., 2006). In addition, exposure to 5 or 10 μM Cd induced expression of ERF1, ETR2 and ACO2 in roots and leaves of A. thaliana plants after 24 and 72 h (Schellingen et al., 2014). Expression of ERF2 and ERF5 was increased in A. thaliana roots and shoots after 2, 6 and 30 h exposure to 5 or 50 μM Cd (Herbette et al., 2006). Recently, a whole-genome transcriptional profile from M. sativa seedlings exposed to 3 μM Hg for 3, 6 and 24 h demonstrated significant upregulation of several ethylene-responsive genes such as ERF1, mostly during the earliest hours of exposure (Montero-Palmero et al., 2014a). Similarly, Hg exposure affected genes related to ethylene signaling in M. truncatula (Zhou et al., 2013), O. sativa (Chen et al., 2014), H. vulgare plants (Lopes et al., 2013). Furthermore, roots of O. sativa plants exposed to 200 μM Cr for up to 3 h showed an increased expression of the EIN3;4 gene (Trinh et al., 2014), while the EIN2 gene was induced in Pb-exposed A. thaliana plants (Cao et al., 2009; Table 1). It is clear from these studies that ethylene signaling is involved in the response of plants to toxic metals (Montero-Palmero et al., 2014b).
Various reports discuss the potential implication of ethylene in plant adaptation or tolerance to toxic metals, and plant genotypes emitting more ethylene were suggested to be more metal resistant than those that release less (Lu and Kirkham, 1991). Moreover, the Pb-hyperaccumulator Sesbania drummondii showed increasing mRNA levels of a putative ACS/ACO gene upon exposure to Pb (Srivastava et al., 2007). Recently, Fu et al. (2014) conducted a transcriptome profiling of genes and pathways associated with As tolerance and toxicity in two A. thaliana ecotypes. In the more tolerant Columbia ecotype, genes encoding components of the ethylene signaling pathway were significantly enriched after short-term As exposure as compared to the sensitive Wassilewskija ecotype (Fu et al., 2014). Similarly, Cao et al. (2014) suggested that Cd tolerance in H. vulgare is related to the induction of ethylene signaling. Transgenic N. tabacum plants overexpressing an ERF gene from Lycium chinense displayed greater tolerance to Cd stress than non-transformed plants (Guan et al., 2015). On the other hand, ethylene insensitive etr1-1 and ein3-3 A. thaliana mutants were shown to be less sensitive to Li than WT plants (Bueso et al., 2007). These apparent conflicting results can be attributed to metal-specific properties, but are definitely related to the chosen experimental setup as discussed before (metal concentration, exposure time, plant species; Table 1, see section “Weighing the Evidence for a Relation between Ethylene and Metal Stress”). Nevertheless, still little is known about the underlying mechanisms of ethylene regulating plant responses to metal stress and potentially affecting sensitivity vs. tolerance (Asgher et al., 2015).
Mutants defective in ethylene biosynthesis and signaling, together with pharmacological compounds to induce or inhibit these processes, have provided an elegant framework to further unravel the involvement of ethylene in plant metal stress responses. In this way, it was shown that ethylene signaling plays an important role during Cd-induced cell death in cultured tomato cells. Exposure to CdSO4 induced rapid cell death and a transiently increased ethylene production within 24 h. Addition of aminoethoxyvinylglycine (AVG) to inhibit ethylene biosynthesis or silver thiosulfate (STS) to block the ethylene receptor led to a marked decrease in Cd-induced cell death (Iakimova et al., 2008). A similar inhibitory effect of AVG was observed during Al-induced cell death in tomato suspension cells (Yakimova et al., 2007).
Using the ethylene-insensitive Never ripe (Nr) tomato mutant, ethylene was demonstrated to be involved in Cd-induced lipid peroxidation in roots, leaves and fruits (Gratão et al., 2012). Mutant A. thaliana plants without functional ACS2 and ACS6 enzymes did not show an increased ethylene release upon short-term (24 to 72 h) exposure to 5 or 10 μM Cd as compared to wild-type (WT) plants. Moreover, Cd-induced decreases in leaf fresh weight were less pronounced in mutants than in WT plants, pointing to a lower Cd sensitivity in the absence of ACS2/6 (Schellingen et al., 2014, 2015a). After prolonged exposure to the same Cd concentrations however, WT and acs2-1/6-1 knockout mutants were equally sensitive, suggesting an early and transient role for ethylene in Cd-induced stress responses (Schellingen et al., 2015a).
Ethylene insensitive ein2-1 mutants are more sensitive to Pb (Cao et al., 2009). This was attributed to an increased uptake of Pb and a diminished GSH content (Cao et al., 2009), revealing crosstalk between ethylene and the biosynthesis of this antioxidant and metal chelating compound. Also other studies link ethylene to the metal-induced oxidative stress response (Sun and Guo, 2013; Zhang et al., 2014; Montero-Palmero et al., 2014a; Schellingen et al., 2015a,b), as is discussed in the next section. These results clearly point toward the potential benefit of altering ethylene biosynthesis and/or signaling in future phytoremediation strategies (Montero-Palmero et al., 2014a). This is also supported by the fact that bacteria producing ACC deaminase (ACD) and thereby diminishing ethylene levels in their host plant, have been successfully used in laboratory and field conditions to protect plants from growth inhibition by elements such as As, Cd, Cu, Ni, Pb, and Zn (reviewed by Glick et al., 2007). This enzyme converts the ethylene precursor ACC into α-ketobutyrate and ammonia, which is subsequently used as nitrogen source by the bacteria. This reduces deleterious ethylene levels in planta and alleviates the associated stress symptoms (Arshad et al., 2007; DalCorso et al., 2013; Glick, 2014). However, it must be emphasized that the beneficial effects of plant-associated bacteria are also related to the increased availability of nutrients such as P and Fe, the production of phytohormones such as auxins and cytokinins and their competition with phytopathogens that could negatively affect plant health and growth (Weyens et al., 2009). Nonetheless, diminishing ethylene levels seems a promising path to explore, as transgenic plants expressing a bacterial ACD gene display a more resistant phenotype than non-transformed plants when exposed to different metals (Arshad et al., 2007; Glick et al., 2007). It has even been shown that plants possess ACD activity themselves (McDonnell et al., 2009), an intriguing asset which could also be exploited in phytoremediation of metal-polluted soils. However, ethylene production and signaling might also be a beneficial part of metal stress responses in plants (Cao et al., 2014; Fu et al., 2014; Thao et al., 2015). Indeed, ethylene can promote as well as inhibit plant growth (Pierik et al., 2006). Therefore, much work remains to be done prior to altering the ethylene response and improving phytoremediation of metal-contaminated soils.
With regard to plant growth in metal-polluted areas, the root architecture is of great importance. Interestingly, ethylene modulates local and systemic responses to low phosphate (Pi), thereby contributing to the remodeling of the root system architecture to increase Pi uptake (Nagarajan and Smith, 2011). As the root system of plants exposed to toxic metals is also drastically changed (Remans et al., 2012), this opens the window to study the potential involvement of ethylene in this response specifically (De Smet et al., 2015). For example, WT A. thaliana plants exposed to increasing Cd concentrations showed a higher lateral root density, which was abolished at higher exposure concentrations. In contrast, the Cd-induced increase in lateral root density was maintained at these higher exposure levels in ethylene insensitive ein3-1 mutants. Ethylene might therefore modulate lateral root outgrowth during high Cd exposure (Remans et al., 2012). Furthermore, ethylene is implicated in the development of root hairs in Cd-exposed B. napus seedlings, as the use of the ethylene biosynthesis inhibitors cobalt chloride (CoCl2) and aminooxyacetic acid (AOA) attenuated the Cd-mediated increase in root hair density (Sun and Guo, 2013). Ethylene may also inhibit primary root growth during the early response to Hg, as roots of M. sativa seedlings exposed to the ethylene receptor inhibitor 1-methylcyclopropene (1-MCP) as well as roots of ethylene insensitive ein2-5 A. thaliana mutants grew more in the presence of moderate Hg concentrations as compared to their untreated or WT counterparts (Montero-Palmero et al., 2014a). In addition, ethylene insensitive etr1-3 and ein2-1 A. thaliana plants were more tolerant to Al stress, as root elongation of both mutants was less inhibited than in WT plants (Sun et al., 2010). For ein2-1, root and leaf growth was also less compromised as compared to the WT after 14 days of Al exposure (Zhang et al., 2014). Similarly, root elongation of Al-exposed A. thaliana plants was less affected in the presence of antagonists of ethylene biosynthesis (AVG and CoCl2) and perception [silver nitrate (AgNO3); Sun et al., 2010]. Recently, it was shown that ethylene negatively regulates Al-induced efflux of malate anions in wheat. As malate forms extracellular complexes with Al, this explains the increased Al tolerance observed in ethylene insensitive genotypes (Tian et al., 2014). Again, the potential benefits of ethylene able to reduce root and plant growth during metal stress should not be ignored when interpreting the above-mentioned results.
Research over the past years points toward an intimate interaction between ethylene and other signaling components implicated in the response of plants to metal stress (Figure 3). In the following sections, the experimental evidence is summarized. Nonetheless, it must be emphasized that our knowledge is still scarce, revealing the need for future research to obtain an integrated picture and potentially apply this information in strategies to cope with phytotoxic metals (Thao et al., 2015).
Figure 3. Ethylene participates in the network of metal-induced signaling responses in plants. Different signaling pathways are affected by metal exposure in plants. (1) Phytohormones such as ethylene, jasmonic acid (JA) and salicylic acid (SA) are influenced by metal stress. In particular, ethylene biosynthesis is generally activated at the level of ACC synthase (ACS) and oxidase (ACO), thereby stimulating the ethylene signaling cascade. (2) Increased generation of reactive oxygen species (ROS, e.g. H2O2) and reactive nitrogen species (e.g. NO•) sets oxidative signaling pathways in motion, for example those mediated by mitogen-activated protein kinases (MAPK). (3) Glutathione (GSH) is a central player in the metal-induced stress network, not only because of its antioxidant function, but also as a precursor for metal-chelating phytochelatins. It is increasingly clear that these individual players integrate and interact within a broad signaling network in metal-exposed plants. Direct interaction between oxidative stress and ethylene biosynthesis is demonstrated by the MAPK-mediated activation of ACS. In addition, ethylene is shown to affect other players such as JA, SA, ROS, NO•, MAPK, and the GSH metabolism as well (indicated by orange dashed arrows).
It is widely accepted that ROS act as signaling molecules in abiotic stress responses, interacting with other signaling pathways in a spatiotemporal manner (Bartoli et al., 2013; Baxter et al., 2014). Oxidative stress characterized by an imbalance between ROS and antioxidants in favor of the former is a recurrent response of metal-exposed plants (Sharma and Dietz, 2009), which triggers downstream responses potentially leading to acclimation. Furthermore, it is increasingly clear that signals related to an increased ROS generation are linked to hormonal signaling pathways (Fujita et al., 2006; Baxter et al., 2014). Different studies demonstrated the involvement of ethylene in the stress-induced oxidative burst, as reviewed by Steffens (2014) during salinity, flooding and metal stress responses in O. sativa. When ethylene production or perception was inhibited by AVG or STS, respectively, camptothecin-induced H2O2 production was blocked in L. esculentum suspension cells (de Jong et al., 2002). As compared to WT plants, ethylene insensitive ein2-1 A. thaliana plants produced less H2O2 and showed a lower O production rate when exposed to paraquat. Consequently, mutant seedlings had a lower increase in malondialdehyde (MDA) content, which suggests less oxidative damage compared to the WT (Cao et al., 2006). Similarly, H2O2 production and MDA content were lower in ein2-1 as compared to WT plants after Al exposure (Zhang et al., 2014). Furthermore, application of the ethylene receptor blocker STS significantly reduced the H2O2 content in roots of Cd-exposed Phaseolus coccineus plants after 1 and 2 h (Maksymiec, 2011). Cadmium-induced production of O at the growing root hair tips of B. napus was blocked by the ethylene biosynthesis inhibitor AOA, suggesting that ethylene signaling acts upstream of O (Sun and Guo, 2013). Finally, A. thaliana cat2-1 mutants that accumulate more H2O2 under normal growth conditions were more tolerant to Li, although they took up more Li as compared to WT plants. Lithium-exposed cat2-1 mutants produced less ethylene and showed less induction of ethylene responsive genes than the WT. Therefore, the authors attributed the increased Li tolerance of cat2-1 mutants to a reduced ethylene production and sensitivity (Bueso et al., 2007).
These results suggest an interaction between ethylene and the ROS network of plants, with ethylene able to affect ROS producing as well as scavenging enzymes and metabolites. The ROS producing NADPH oxidases [also known as respiratory burst oxidase homologs (RBOH)] are put forward as critical signaling hubs in the response of plants to environmental stimuli (Suzuki et al., 2011) such as metal exposure (Remans et al., 2010). Ethylene is an important upstream regulator of O-producing NADPH oxidases (Chae and Lee, 2001), with a regulatory interaction between the ethylene biosynthesis gene ACS1 and RBOHD/F transcription in B. oleracea seedlings (Jakubowicz et al., 2010). In Ipomoea batatas, the NADPH oxidase inhibitor diphenyleneiodonium decreased ROS production induced by ethephon, an ethylene releasing compound (Chen et al., 2013). Furthermore, ethylene seems to stimulate the apoplastic release of H2O2 by activating NADPH oxidase isoform D (RBOHD) during biotic stress, as flagellin (flg22)-induced ROS generation diminished in ethylene insensitive etr1-1 and ein2-1 A. thaliana mutants as compared to the WT (Mersmann et al., 2010). Recent reports also indicate a relationship between ethylene and NADPH oxidase during metal stress. For example, inhibition of the ethylene receptors by 1-MCP reduced or even abolished the increase in extracellular H2O2 production and NADPH oxidase activity observed during the first 6 h of Hg exposure in M. sativa root segments (Montero-Palmero et al., 2014a). In addition, Hg-exposed ethylene insensitive ein2-5 mutants produced less H2O2 as compared to their WT counterparts (Montero-Palmero et al., 2014a). Upon Cd exposure, expression of RBOHC did not increase to WT levels in leaves of acs2-1/6-1 knockout, ein2-1, and ein2-5 mutant A. thaliana plants (Keunen et al., 2015), again supporting a link between ethylene and ROS production by NADPH oxidases during metal stress. Furthermore, ethylene signaling was also related to the transcriptional induction of ALTERNATIVE OXIDASE 1a/d (AOX1a/d), which was lower in leaves of Cd-exposed ein2-1 and ein2-5 mutants as compared to WT plants. This enzyme regulates ROS levels and is suggested to modulate the Cd-induced oxidative challenge in A. thaliana, requiring ethylene—either directly or indirectly via RBOHC—to be fully induced at the transcript level (Keunen et al., 2015). In line with this, AOX was demonstrated to be involved in ethylene-induced plant cell death as well (Lei et al., 2003).
On the other hand, ethylene might affect the plant's antioxidative defense network as shown by Cao et al. (2006). They demonstrated a constitutively higher transcription of Cu/Zn SOD 2 (CSD2) and CAT3 genes, leading to enhanced SOD and CAT enzyme activities in ein2-1 mutants as compared to WT plants. This was also shown in Al-exposed ein2-1 mutants, which showed differential responses at the level of SOD and CAT activities compared to WT plants (Zhang et al., 2014). The interaction between ethylene and antioxidative defense is further underlined by the fact that ein2-1, ein3-1 and ein4 mutant A. thaliana plants have a higher AsA content in leaves (Gergoff et al., 2010), which was also observed in ethylene insensitive Nr tomato fruits (Alba et al., 2005). Conversely, the ctr1-1 mutant with a loss-of-function of the negative regulator CTR1 displayed lower leaf AsA levels (Gergoff et al., 2010). Concurrently, ethylene signaling was reported to suppress AsA synthesis and accumulation in tomato leaves (Mazorra Morales et al., 2014). In this regard, it is noteworthy to mention that ethylene biosynthesis requires AsA in the final step (cfr. supra), further supporting crosstalk between both compounds. Finally, mutants defective in ethylene perception (etr1-1) as well as those overproducing ethylene (eto1-1) showed up to five-fold higher α-tocopherol levels during leaf aging. Furthermore, ethylene insensitive ein3-1 mutants showed a delayed increase in α-tocopherol during water stress (Cela et al., 2009). This antioxidant compound was shown to be essential for the tolerance of A. thaliana plants to metal-induced oxidative stress (Collin et al., 2008). Therefore, the interaction between ethylene and the antioxidative defense network mounted during metal exposure can ultimately affect responses leading to sensitivity or tolerance and deserves further investigation. In particular, a relation between ethylene and GSH is suggested and is discussed in a separate section.
Besides ROS, plant cells also produce reactive nitrogen species (RNS) such as nitric oxide (NO•) during abiotic stress (Corpas et al., 2011) such as Cd exposure (Arasimowicz-Jelonek et al., 2011; Chmielowska-Bąk et al., 2014). However, the functional role of NO• in metal-challenged plants is not yet fully understood. Different results point toward an interaction between NO• and ethylene, as recently reviewed by Mur et al. (2013). During salt stress, NO• and ethylene were shown to cooperate in the modulation of ion homeostasis. Salt stress-induced NO• production greatly enhanced ethylene emission in A. thaliana callus. In its turn, ethylene stimulated the expression of plasma membrane H+-ATPase genes, which has been suggested to facilitate Na+ efflux into the apoplast and attenuate Na+ toxicity under saline conditions (Wang et al., 2009). Such regulatory interactions between NO• and ethylene might also be involved in the response of plants to metal stress. Interestingly, both ethylene and NO• are involved in the upregulation of key genes related to Fe acquisition and homeostasis in A. thaliana (García et al., 2010). Iron deficiency is a well-known consequence of metal toxicity [e.g. Cd (Xu et al., 2015)] and seems to increase ethylene sensitivity (García et al., 2010), which potentially affects metal-induced responses related to ethylene as well. It has been shown that nutrient stress—either a reduced or increased availability—affects ethylene biosynthesis and perception in plants via the induction of an oxidative burst (Iqbal et al., 2013a), again highlighting the link between ethylene and ROS signaling.
Tolerance to toxic metals is highly dependent on the metabolism of GSH, a widely distributed biothiol tripeptide (γ-glutamyl-cysteinyl-glycine) in plant cells. As metal chelator, antioxidant and signaling compound, GSH is a key player in metal-induced oxidative stress defenses (Seth et al., 2012). This multifunctional role is related to the sulfhydryl group in cysteine, which has a high affinity toward metals such as Cd and Hg. Furthermore, GSH is the precursor molecule for the synthesis of phytochelatins (PCs), which consist of 2 to 11 GSH molecules and limit the cellular concentration of free metal ions (Jozefczak et al., 2012; Hernández et al., 2015). Using ethephon, it was shown that ethylene induces the activity of ATP sulfurylase (ATPS), leading to an accumulation of sulfur (S) in B. juncea (Iqbal et al., 2012). Recently, Iqbal et al. (2013b) reviewed the crosstalk between S assimilation and ethylene signaling in plants. Since GSH synthesis is affected by S availability to produce the amino acid cysteine, ethylene might modulate this process in order to meet the increasing demands for GSH during metal stress. In this regard, it is important to mention that ethylene synthesis itself also requires cysteine to ultimately produce SAM (Figure 4).
Figure 4. Simplified scheme of the interaction between sulfur assimilation, ethylene and glutathione biosynthesis in plants. Sulfur is taken up from the soil as sulfate, which is converted into adenosine 5-phosphosulfate by the enzyme ATP sulfurylase (ATPS). This is further reduced by adenosine 5-phosphosulfate reductase (APR) into sulfite, which is subsequently reduced into sulfide by sulfite reductase (SiR). The enzyme O-acetylserine (thiol) lyase (OASTL) produces cysteine, which is one of the three building blocks that make up glutathione. During glutathione biosynthesis, cysteine is coupled to glutamate by γ-glutamylcysteine synthetase (GSH1) to form γ-glutamylcysteine. In the next step, glycine is added by glutathione synthetase (GSH2) to finally produce glutathione. In addition, cysteine is also required for ethylene formation, as methionine is derived from cysteine via different reactions (depicted by the dashed arrow). In the ethylene biosynthetic pathway, methionine is converted to S-adenosyl-methionine (SAM) by SAM synthetase. In the next steps, 1-aminocyclopropane-1-carboxylic acid (ACC) is produced by ACC synthase (ACS) and subsequently oxidized by ACC oxidase (ACO) to form ethylene (see Figure 1). As ethylene and glutathione fulfill important functions in metal-exposed plants, a trade-off between both might lie at the heart of their interaction regulating plant responses to metal stress.
Crosstalk between ethylene and GSH is suggested by the observed upregulation of different genes encoding ERF transcription factors in the severe GSH deficient root meristemless 1-1 (rml1-1) A. thaliana mutants as compared to WT plants (Schnaubelt et al., 2015). On the other hand, ERF2 expression was significantly repressed in cadmium sensitive 2-1 (cad2-1) A. thaliana mutants that also have lower GSH levels than WT plants, but not as low as those of rml1-1 mutants (Han et al., 2013). Transgenic N. tabacum plants with an enhanced GSH content showed induced ethylene biosynthesis (ACO) and signaling (ERF) genes (Ghanta et al., 2014). The potential interplay between GSH and ethylene is further underlined by the results of Chen et al. (2013), who demonstrated that exogenous GSH mitigated the ethephon-induced increase in ROS production in sweet potato. Furthermore, ethylene was suggested to increase de novo biosynthesis of GSH in ozone-exposed A. thaliana plants, thereby protecting against leaf injury (Yoshida et al., 2009).
Increasing evidence points toward a close relationship between ethylene and GSH metabolism during metal stress. For example, ethephon treatment increased GSH levels in Cd-exposed B. juncea (Masood et al., 2012). Similarly, GSH levels in Ni- and Zn-exposed B. juncea plants were higher after ethephon application, which alleviated metal toxicity (Khan and Khan, 2014). While ACS activity and ethylene production decreased, GSH levels increased in Cd-exposed T. aestivum supplied with S (Khan et al., 2015). Transcript levels of genes encoding GSH biosynthesis enzymes were significantly less upregulated, concomitantly with lower GSH levels in leaves of Cd-exposed acs2-1/6-1 knockout vs. WT A. thaliana plants. Therefore, increased ethylene biosynthesis upon Cd exposure seems crucial to mount effective defense responses related to GSH (Schellingen et al., 2015a). In addition, ethylene signaling is implicated in the accumulation of GSH in Al-exposed A. thaliana (Zhang et al., 2014), Cd-exposed L. chinense (Guan et al., 2015), and A. thaliana plants (Schellingen et al., 2015b). The increased Cd tolerance of transgenic tobacco plants overexpressing an ERF gene from L. chinense was related to an enhanced expression level of GSH biosynthesis genes (Guan et al., 2015). Similar results were obtained in Pb-exposed A. thaliana plants, where EIN2 is indispensable to confer metal resistance partially by increasing GSH levels (Cao et al., 2009). These results confirm the suggested interplay between ethylene and GSH in determining metal tolerance vs. sensitivity and open the window to future experiments exploiting this relationship in phytoremediation strategies (Hernández et al., 2015). As discussed before, it should be kept in mind that not all metal(loid)s are equally strong inducers of PC synthesis (Anjum et al., 2015) and will therefore differentially affect GSH levels in plants.
A signaling pathway linked to ethylene and worthwhile to discuss in the light of metal stress is the ROS-induced MAPK cascade. These kinases are activated at transcript and activity level in different plant species exposed to metals. Furthermore, they interfere with hormone biosynthesis and signaling to activate downstream responses (Opdenakker et al., 2012). As mentioned before, the stress-responsive MPK3 and MPK6 isoforms increase ethylene production by affecting ACS2 and ACS6 transcription as well as protein stability (Liu and Zhang, 2004; Li et al., 2012). In addition, MAPK kinase 9 (MKK9) was shown to activate the MPK3/MPK6 cascade and stimulate ethylene biosynthesis in A. thaliana (Xu et al., 2008). Other studies have indicated that MAPKs could be involved in ethylene signaling as well (Ouaked et al., 2003; Hahn and Harter, 2009). This might come as no surprise since the negative regulator of ethylene signaling, CTR1, shows sequence similarities with Raf protein kinases and has been presumed to function as a MAPK kinase kinase (MAPKKK). However, no conclusive CTR1-targeted kinases have been identified yet (Zhao and Guo, 2011; Ju and Chang, 2012; Merchante et al., 2013). Nonetheless, nuclear EIN3 was shown to be regulated not only by CTR1 but also by a novel MAPK cascade mediated by MKK9 and MPK3/6 in A. thaliana. This cascade functions downstream of CTR1, is activated when ethylene binds to its receptors and stabilizes EIN3 by phosphorylation (Yoo et al., 2008). Recently, Schellingen et al. (2015b) proposed a model where MPK3/6 link ROS production and ethylene signaling during Cd stress in A. thaliana leaves. In this model, Cd exposure activates NADPH oxidases, which produce ROS that are sensed by the oxidative signal-inducible kinase1 (OXI1). This kinase then activates MPK3/6, both affecting ACS2/6 enzymes at multiple levels (Schellingen et al., 2015b). Furthermore, Liu et al. (2010) have shown that pretreatment with GSH reduced the activation of MPK3 and MPK6 under Cd stress in A. thaliana. This suggests that ROS are involved in Cd-induced MAPK signaling, with a relation to ethylene as both MPK3/6 are able to affect ethylene biosynthesis enzymes (Thao et al., 2015). Therefore, the potential implication of MAPK signaling and its relation with ethylene biosynthesis and/or signaling during metal stress should be explored in more detail.
Interactions between various phytohormones are required to integrate environmental signals and stress tolerance responses (De Paepe and Van Der Straeten, 2005; Kohli et al., 2013). In addition to ethylene, jasmonic acid (JA) and salicylic acid (SA) are mostly implicated in plant stress responses (Van de Poel et al., 2015) such as those mounted during metal exposure (De Smet et al., 2015). Different genes involved in ethylene and JA biosynthesis as well as genes responsive to these hormones were differentially expressed after Hg exposure in M. sativa, M. truncatula and H. vulgare plants (Montero-Palmero et al., 2014b). Gene expression profiling in Cr-stressed O. sativa roots indicated activation of ethylene and JA signaling pathways (Trinh et al., 2014). Furthermore, JA levels rapidly increased in A. thaliana and P. coccineus plants exposed to Cd or Cu (Maksymiec et al., 2005). From a signaling point of view, JA was shown to trigger ROS production in short-term Cd- and Cu-exposed A. thaliana plants, as inhibiting JA biosynthesis by propyl gallate decreased O and H2O2 levels after metal exposure (Maksymiec and Krupa, 2006).
A mutual relationship exists between ethylene and JA signaling (Song et al., 2014), which might ultimately affect metal stress responses as well. For example, it has been shown that the JA receptor CORONATINE INSENSITIVE 1 (COI1) is implicated in the inhibition of Arabidopsis root growth mediated by ethylene in the light (Adams and Turner, 2010). Furthermore, ethylene insensitive ein2-1 mutants become ethylene responsive by reducing JA levels via a genetic or chemical approach (Kim et al., 2013). Crosstalk between ethylene and SA during metal stress was supported by the results of Zhang et al. (2014). They showed that Arabidopsis mutants insensitive to ethylene (ein2-1) or SA [nonexpressor of pathogenesis-related proteins 1-1 (npr1-1)] were more tolerant to Al exposure as compared to WT plants. However, ein2-1/npr1-1 double mutants were less tolerant than WT plants, indicating that the tolerant phenotype of ein2-1 and npr1-1 single mutants depended on remaining NPR or EIN function, respectively (Zhang et al., 2014). These results further support studying the complex interaction between various hormonal signaling pathways mediating metal stress responses in plants.
As for ethylene, different studies support a functional link between GSH and JA as well. Mutant A. thaliana plants without functional GR1 displayed a constitutive increase in oxidized glutathione disulfide (GSSG), which affected the expression of genes involved in JA metabolism dependent on day length conditions (Mhamdi et al., 2010). Expression levels of two JA signaling marker genes [plant defensin 1.2 (PDF1.2) and vegetative storage protein 2 (VSP2)] were significantly lower in GSH deficient cad2-1 or regulator of APX2 1-1 (rax1-1) mutants. Similar results were obtained when GSH biosynthesis was chemically inhibited by buthionine sulfoximine (BSO) in WT plants. In addition, microarray analysis revealed a multitude of genes involved in JA synthesis, activation and signaling to be differentially expressed in cad2-1 mutants, indicating that the basal expression of JA-associated genes is affected by the content of GSH (Han et al., 2013). In the conditional oxidative stress signaling mutant cat2 with H2O2-induced accumulation of GSSG under ambient air and moderate light conditions, the JA pathway was upregulated. However, this response was attenuated in a cat2 cad2 double mutant, showing that GSH also regulates oxidative stress-induced JA-related gene expression in A. thaliana (Han et al., 2013). In addition to JA, SA was also shown to interact with GSH, as transgenic tobacco plants with an enhanced GSH content showed induction of SA-related genes such as PATHOGENESIS-RELATED PROTEIN 1a (PR1a; Ghanta et al., 2014). As ethylene and GSH are clearly intertwined during metal stress responses, it might be worthwhile to investigate the involvement of JA and SA in this interaction. In addition, JA signaling also involves the MAPK cascade MKK3/MPK6 (Takahashi et al., 2007) and NO• does not only affect ethylene but also JA and SA signaling cascades (Mur et al., 2013). Therefore, it is recommended to further dissect the hormonal crosstalk affecting plant responses to metal stress (also reviewed by Thao et al., 2015) and address their interaction with oxidative stress and particularly GSH, the MAPK cascade and NO• in future experiments.
Ethylene is involved in many processes throughout the entire life cycle of plants, including responses to environmental stimuli such as metal exposure. Our current knowledge on the role of ethylene in metal-induced stress responses, as well as its integration within a broad network of signaling compounds, is gradually expanding. Recent evidence points toward an intimate link between ethylene, the cellular redox balance with GSH as important antioxidant and other phytohormones such as JA and SA, finally affecting plant metal sensitivity vs. tolerance. Nevertheless, much work remains to be done before this information can be applied in practice.
EK, KS, JV and AC participated in the conception of the topic. EK and AC wrote the manuscript. All authors read and approved the final manuscript after critically revising it for important intellectual content.
This work was supported by the Research Foundation Flanders (FWO) by a grant for EK and project [G0D3414]. Additional funding came from Hasselt University through a PhD grant for KS and the Methusalem project [08M03VGRJ].
The authors declare that the research was conducted in the absence of any commercial or financial relationships that could be construed as a potential conflict of interest.
Abeles, F. B., Morgan, P. W., and Saltveit, M. E. (1992). Ethylene in Plant Biology. San Diego, CA: Academic Press Inc.
Adams, E., and Turner, J. (2010). COI1, a jasmonate receptor, is involved in ethylene-induced inhibition of Arabidopsis root growth in the light. J. Exp. Bot. 61, 4373–4386. doi: 10.1093/jxb/erq240
Alba, R., Payton, P., Fei, Z., McQuinn, R., Debbie, P., Martin, G. B., et al. (2005). Transcriptome and selected metabolite analyses reveal multiple points of ethylene control during tomato fruit development. Plant Cell 17, 2954–2965. doi: 10.1105/tpc.105.036053
Alloway, B. J. (2012). “Sources of heavy metals and metalloids in soils,” in Heavy Metals in Soils: Trace Metals And Metalloids in Soils and Their Bioavailability, ed B. J. Alloway (Heidelberg: Springer), 11–50.
Alonso, J. M., Hirayama, T., Roman, G., Nourizadeh, S., and Ecker, J. R. (1999). EIN2, a bifunctional transducer of ethylene and stress responses in Arabidopsis. Science 284, 2148–2152. doi: 10.1126/science.284.5423.2148
Anjum, N. A., Hasanuzzaman, M., Hossain, M. A., Thangavel, P., Roychoudhury, A., Gill, S. S., et al. (2015). Jacks of metal/metalloid chelation trade in plants – an overview. Front. Plant Sci. 6:192. doi: 10.3389/fpls.2015.00192
Arasimowicz-Jelonek, M., Floryszak-Wieczorek, J., and Gwóźdź, E. A. (2011). The message of nitric oxide in cadmium challenged plants. Plant Sci. 181, 612–620. doi: 10.1016/j.plantsci.2011.03.019
Argueso, C. T., Hansen, M., and Kieber, J. J. (2007). Regulation of ethylene biosynthesis. J. Plant Growth Regul. 26, 92–105. doi: 10.1007/s00344-007-0013-5
Arshad, M., Saleem, M., and Hussain, S. (2007). Perspectives of bacterial ACC deaminase in phytoremediation. Trends Biotechnol. 25, 356–362. doi: 10.1016/j.tibtech.2007.05.005
Arteca, J. M., and Arteca, R. N. (1999). A multi-responsive gene encoding 1-aminocyclopropane-1-carboxylate synthase (ACS6) in mature Arabidopsis leaves. Plant Mol. Biol. 39, 209–219. doi: 10.1023/A:1006177902093
Arteca, R. N., and Arteca, J. M. (2007). Heavy-metal-induced ethylene production in Arabidopsis thaliana. J. Plant Physiol. 164, 1480–1488. doi: 10.1016/j.jplph.2006.09.006
Asgher, M., Khan, M. I. R., Anjum, N. A., and Khan, N. A. (2015). Minimising toxicity of cadmium in plants - role of plant growth regulators. Protoplasma 252, 399–413. doi: 10.1007/s00709-014-0710-4
Asgher, M., Khan, N. A., Khan, M. I. R., Fatma, M., and Masood, A. (2014). Ethylene production is associated with alleviation of cadmium-induced oxidative stress by sulfur in mustard types differing in ethylene sensitivity. Ecotoxicol. Environ. Saf. 106, 54–61. doi: 10.1016/j.ecoenv.2014.04.017
Bartoli, C. G., Casalongué, C. A., Simontacchi, M., Marquez-Garcia, B., and Foyer, C. H. (2013). Interactions between hormone and redox signalling pathways in the control of growth and cross tolerance to stress. Environ. Exp. Bot. 94, 73–88. doi: 10.1016/j.envexpbot.2012.05.003
Baxter, A., Mittler, R., and Suzuki, N. (2014). ROS as key players in plant stress signalling. J. Exp. Bot. 65, 1229–1240. doi: 10.1093/jxb/ert375
Bisson, M. M. A., Bleckmann, A., Allekotte, S., and Groth, G. (2009). EIN2, the central regulator of ethylene signalling, is localized at the ER membrane where it interacts with the ethylene receptor ETR1. Biochem. J. 424, 1–6. doi: 10.1042/BJ20091102
Bisson, M. M. A., and Groth, G. (2010). New insight in ethylene signaling: autokinase activity of ETR1 modulates the interaction of receptors and EIN2. Mol. Plant 3, 882–889. doi: 10.1093/mp/ssq036
Bleecker, A. B., and Kende, H. (2000). Ethylene: a gaseous signal molecule in plants. Annu. Rev. Cell Dev. Biol. 16, 1–18. doi: 10.1146/annurev.cellbio.16.1.1
Bueso, E., Alejandro, S., Carbonell, P., Perez-Amador, M. A., Fayos, J., Bellés, J. M., et al. (2007). The lithium tolerance of the Arabidopsis cat2 mutant reveals a cross-talk between oxidative stress and ethylene. Plant J. 52, 1052–1065. doi: 10.1111/j.1365-313X.2007.03305.x
Cao, F., Chen, F., Sun, H., Zhang, G., Chen, Z.-H., and Wu, F. (2014). Genome-wide transcriptome and functional analysis of two contrasting genotypes reveals key genes for cadmium tolerance in barley. BMC Genomics 15:611. doi: 10.1186/1471-2164-15-611
Cao, S., Chen, Z., Liu, G., Jiang, L., Yuan, H., Ren, G., et al. (2009). The Arabidopsis Ethylene-Insensitive 2 gene is required for lead resistance. Plant Physiol. Biochem. 47, 308–312. doi: 10.1016/j.plaphy.2008.12.013
Cao, S., Jiang, S., and Zhang, R. (2006). Evidence for a role of Ethylene-Insensitive 2 gene in the regulation of the oxidative stress response in Arabidopsis. Acta Physiol. Plant. 28, 417–425. doi: 10.1007/BF02706624
Carrió-Seguí, A., Garcia-Molina, A., Sanz, A., and Peñarrubia, L. (2015). Defective copper transport in the copt5 mutant affects cadmium tolerance. Plant Cell Physiol. 56, 442–454. doi: 10.1093/pcp/pcu180
Cela, J., Falk, J., and Munné-Bosch, S. (2009). Ethylene signaling may be involved in the regulation of tocopherol biosynthesis in Arabidopsis thaliana. FEBS Lett. 583, 992–996. doi: 10.1016/j.febslet.2009.02.036
Chae, H. S., and Kieber, J. J. (2005). Eto brute? Role of ACS turnover in regulating ethylene biosynthesis. Trends Plant Sci. 10, 291–296. doi: 10.1016/j.tplants.2005.04.006
Chae, H. S., and Lee, W. S. (2001). Ethylene- and enzyme-mediated superoxide production and cell death in carrot cells grown under carbon starvation. Plant Cell Rep. 20, 256–261. doi: 10.1007/s002990000307
Chen, H.-J., Huang, C.-S., Huang, G.-J., Chow, T.-J., and Lin, Y.-H. (2013). NADPH oxidase inhibitor diphenyleneiodonium and reduced glutathione mitigate ethephon-mediated leaf senescence, H2O2elevation and senescence-associated gene expression in sweet potato (Ipomoea batatas). J. Plant Physiol. 170, 1471–1483. doi: 10.1016/j.jplph.2013.05.015
Chen, Y.-A., Chi, W.-C., Trinh, N. N., Huang, L.-Y., Chen, Y.-C., Cheng, K.-T., et al. (2014). Transcriptome profiling and physiological studies reveal a major role for aromatic amino acids in mercury stress tolerance in rice seedlings. PLoS ONE 9:e95163. doi: 10.1371/journal.pone.0095163
Chmielowska-Bąk, J., Gzyl, J., Ruciñska-Sobkowiak, R., Arasimowicz-Jelonek, M., and Deckert, J. (2014). The new insights into cadmium sensing. Front. Plant Sci. 5:245. doi: 10.3389/fpls.2014.00245
Chmielowska-Bąk, J., Lefèvre, I., Lutts, S., and Deckert, J. (2013). Short term signaling responses in roots of young soybean seedlings exposed to cadmium stress. J. Plant Physiol. 170, 1585–1594. doi: 10.1016/j.jplph.2013.06.019
Collin, V. C., Eymery, F., Genty, B., Rey, P., and Havaux, M. (2008). Vitamin E is essential for the tolerance of Arabidopsis thaliana to metal-induced oxidative stress. Plant Cell Environ. 31, 244–257. doi: 10.1111/j.1365-3040.2007.01755.x
Corpas, F. J., Leterrier, M., Valderrama, R., Airaki, M., Chaki, M., Palma, J. M., et al. (2011). Nitric oxide imbalance provokes a nitrosative response in plants under abiotic stress. Plant Sci. 181, 604–611. doi: 10.1016/j.plantsci.2011.04.005
Cuypers, A., Smeets, K., and Vangronsveld, J. (2009). “Heavy metal stress in plants” in Plant Stress Biology. From Genomics to Systems Biology, ed H. Hirt (Weinheim: Wiley-VCH Verlagsgesellschaft GmbH & Co. KGaA), 161–178.
DalCorso, G., Manara, A., and Furini, A. (2013). An overview of heavy metal challenge in plants: from roots to shoots. Metallomics 5, 1117–1132. doi: 10.1039/c3mt00038a
Dat, J., Vandenabeele, S., Vranová, E., Van Montagu, M., Inzé, D., and Van Breusegem, F. (2000). Dual action of the active oxygen species during plant stress responses. Cell. Mol. Life Sci. 57, 779–795. doi: 10.1007/s000180050041
de Jong, A., Yakimova, E., Kapchina, V. M., and Woltering, E. J. (2002). A critical role for ethylene in hydrogen peroxide release during programmed cell death in tomato suspension cells. Planta 214, 537–545. doi: 10.1007/s004250100654
De Martinis, D., Koyama, T., and Chang, K. (2015). Ethylene is all around. Front. Plant Sci. 6:76. doi: 10.3389/fpls.2015.00076
De Paepe, A., and Van Der Straeten, D. (2005). Ethylene biosynthesis and signaling: an overview. Vitam. Horm. 72, 399–430. doi: 10.1016/S0083-6729(05)72011-2
De Paepe, A., Vuylsteke, M., Van Hummelen, P., Zabeau, M., and Van Der Straeten, D. (2004). Transcriptional profiling by cDNA-AFLP and microarray analysis reveals novel insights into the early response to ethylene in Arabidopsis. Plant J. 39, 537–559. doi: 10.1111/j.1365-313X.2004.02156.x
De Smet, S., Cuypers, A., Vangronsveld, J., and Remans, T. (2015). Gene networks involved in hormonal control of root development in Arabidopsis thaliana: a framework for studying its disturbance by metal stress. Int. J. Mol. Sci. 16, 19195–19224. doi: 10.3390/ijms160819195
Diaz-Vivancos, P., Barba-Espín, G., and Hernández, J. A. (2013). Elucidating hormonal/ROS networks during seed germination: insights and perspectives. Plant Cell Rep. 32, 1491–1502. doi: 10.1007/s00299-013-1473-7
Dietz, K.-J., Vogel, M. O., and Viehhauser, A. (2010). AP2/EREBP transcription factors are part of gene regulatory networks and integrate metabolic, hormonal and environmental signals in stress acclimation and retrograde signalling. Protoplasma 245, 3–14. doi: 10.1007/s00709-010-0142-8
Dilley, D. R., Wang, Z., Kadirjan-Kalbach, D. K., Ververidis, F., Beaudry, R., and Padmanabhan, K. (2013). 1-Aminocyclopropane-1-carboxylic acid oxidase reaction mechanism and putative post-translational activities of the ACCO protein. AoB Plants 5, plt031. doi: 10.1093/aobpla/plt031
Dolferus, R. (2014). To grow or not to grow: a stressful decision for plants. Plant Sci. 229, 247–261. doi: 10.1016/j.plantsci.2014.10.002
Dorling, S. J., Leung, S., Anderson, C. W. N., Albert, N. W., and McManus, M. T. (2011). Changes in 1-aminocyclopropane-1-carboxlate (ACC) oxidase expression and enzyme activity in response to excess manganese in white clover (Trifolium repens L.). Plant Physiol. Biochem. 49, 1013–1019. doi: 10.1016/j.plaphy.2011.04.007
Fu, S.-F., Chen, P.-Y., Nguyen, Q. T. T., Huang, L.-Y., Zeng, G.-R., Huang, T.-L., et al. (2014). Transcriptome profiling of genes and pathways associated with arsenic toxicity and tolerance in Arabidopsis. BMC Plant Biol. 14:94. doi: 10.1186/1471-2229-14-94
Fujita, M., Fujita, Y., Noutoshi, Y., Takahashi, F., Narusaka, Y., Yamaguchi-Shinozaki, K., et al. (2006). Crosstalk between abiotic and biotic stress responses: a current view from the points of convergence in the stress signaling networks. Curr. Opin. Plant Biol. 9, 436–442. doi: 10.1016/j.pbi.2006.05.014
Gane, R. (1934). Production of ethylene by some ripening fruits. Nature 134, 1008. doi: 10.1038/1341008a0
Gao, Z., Chen, Y.-F., Randlett, M. D., Zhao, X.-C., Findell, J. L., Kieber, J. J., et al. (2003). Localization of the Raf-like kinase CTR1 to the endoplasmic reticulum of Arabidopsis through participation in ethylene receptor signaling complexes. J. Biol. Chem. 278, 34725–34732. doi: 10.1074/jbc.M305548200
García, M. J., Lucena, C., Romera, F. J., Alcántara, E., and Pérez-Vicente, R. (2010). Ethylene and nitric oxide involvement in the up-regulation of key genes related to iron acquisition and homeostasis in Arabidopsis. J. Exp. Bot. 61, 3885–3899. doi: 10.1093/jxb/erq203
Gergoff, G., Chaves, A., and Bartoli, C. G. (2010). Ethylene regulates ascorbic acid content during dark-induced leaf senescence. Plant Sci. 178, 207–212. doi: 10.1016/j.plantsci.2009.12.003
Ghanta, S., Datta, R., Bhattacharyya, D., Sinha, R., Kumar, D., Hazra, S., et al. (2014). Multistep involvement of glutathione with salicylic acid and ethylene to combat environmental stress. J. Plant Physiol. 171, 940–950. doi: 10.1016/j.jplph.2014.03.002
Glick, B. R. (2014). Bacteria with ACC deaminase can promote plant growth and help to feed the world. Microbiol. Res. 169, 30–39. doi: 10.1016/j.micres.2013.09.009
Glick, B. R., Cheng, Z., Czarny, J., and Duan, J. (2007). Promotion of plant growth by ACC deaminase-producing soil bacteria. Eur. J. Plant Pathol. 119, 329–339. doi: 10.1007/s10658-007-9162-4
Gratão, P. L., Monteiro, C. C., Carvalho, R. F., Tezotto, T., Piotto, F. A., Peres, L. E. P., et al. (2012). Biochemical dissection of diageotropica and Never ripe tomato mutants to Cd-stressful conditions. Plant Physiol. Biochem. 56, 79–96. doi: 10.1016/j.plaphy.2012.04.009
Groppa, M. D., Benavides, M. P., and Tomaro, M. L. (2003). Polyamine metabolism in sunflower and wheat leaf discs under cadmium or copper stress. Plant Sci. 164, 293–299. doi: 10.1016/S0168-9452(02)00412-0
Guan, C., Ji, J., Wu, D., Li, X., Jin, C., Guan, W., et al. (2015). The glutathione synthesis may be regulated by cadmium-induced endogenous ethylene in Lycium chinense, and overexpression of an ethylene responsive transcription factor gene enhances tolerance to cadmium stress in tobacco. Mol. Breed. 35, 123. doi: 10.1007/s11032-015-0313-6
Guo, H., and Ecker, J. R. (2003). Plant responses to ethylene gas are mediated by SCFEBF1∕EBF2-dependent proteolysis of EIN3 transcription factor. Cell 115, 667–677. doi: 10.1016/S0092-8674(03)00969-3
Hahn, A., and Harter, K. (2009). Mitogen-activated protein kinase cascades and ethylene: signaling, biosynthesis, or both? Plant Physiol. 149, 1207–1210. doi: 10.1104/pp.108.132241
Han, L., Li, G.-J., Yang, K.-Y., Mao, G., Wang, R., Liu, Y., et al. (2010). Mitogen-activated protein kinase 3 and 6 regulate Botrytis cinerea-induced ethylene production in Arabidopsis. Plant J. 64, 114–127. doi: 10.1111/j.1365-313x.2010.04318.x
Han, Y., Mhamdi, A., Chaouch, S., and Noctor, G. (2013). Regulation of basal and oxidative stress-triggered jasmonic acid-related gene expression by glutathione. Plant Cell Environ. 36, 1135–1146. doi: 10.1111/pce.12048
Hänsch, R., and Mendel, R. R. (2009). Physiological functions of mineral micronutrients (Cu, Zn, Mn, Fe, Ni, Mo, B, Cl). Curr. Opin. Plant Biol. 12, 259–266. doi: 10.1016/j.pbi.2009.05.006
Herbette, S., Taconnat, L., Hugouvieux, V., Piette, L., Magniette, M. -L. M., Cuine, S., et al. (2006). Genome-wide transcriptome profiling of the early cadmium response of Arabidopsis roots and shoots. Biochimie 88, 1751–1765. doi: 10.1016/j.biochi.2006.04.018
Hernández, L. E., Sobrino-Plata, J., Montero-Palmero, M. B., Carrasco-Gil, S., Flores-Cáceres, M. L., Ortega-Villasante, C., et al. (2015). Contribution of glutathione to the control of cellular redox homeostasis under toxic metal and metalloid stress. J. Exp. Bot. 66, 2901–2911. doi: 10.1093/jxb/erv063
Hirayama, T., Kieber, J. J., Hirayama, N., Kogan, M., Guzman, P., Nourizadeh, S., et al. (1999). RESPONSIVE-TO-ANTAGONIST1, a Menkes/Wilson disease–related copper transporter, is required for ethylene signaling in Arabidopsis. Cell 97, 383–393. doi: 10.1016/S0092-8674(00)80747-3
Hua, J., and Meyerowitz, E. M. (1998). Ethylene responses are negatively regulated by a receptor gene family in Arabidopsis thaliana. Cell 94, 261–271. doi: 10.1016/S0092-8674(00)81425-7
Iakimova, E. T., Woltering, E. J., Kapchina-Toteva, V. M., Harren, F. J. M., and Cristescu, S. M. (2008). Cadmium toxicity in cultured tomato cells - Role of ethylene, proteases and oxidative stress in cell death signaling. Cell Biol. Int. 32, 1521–1529. doi: 10.1016/j.cellbi.2008.08.021
Iqbal, N., Khan, N. A., Nazar, R., and Teixeira da Silva, J. A. (2012). Ethylene-stimulated photosynthesis results from increased nitrogen and sulfur assimilation in mustard types that differ in photosynthetic capacity. Environ. Exp. Bot. 78, 84–90. doi: 10.1016/j.envexpbot.2011.12.025
Iqbal, N., Masood, A., Khan, M. I. R., Asgher, M., Fatma, M., and Khan, N. A. (2013b). Cross-talk between sulfur assimilation and ethylene signaling in plants. Plant Signal. Behav. 8, 104–112. doi: 10.4161/psb.22478
Iqbal, N., Trivellini, A., Masood, A., Ferrante, A., and Khan, N. A. (2013a). Current understanding on ethylene signaling in plants: the influence of nutrient availability. Plant Physiol. Biochem. 73, 128–138. doi: 10.1016/j.plaphy.2013.09.011
Jakubowicz, M., Gałañska, H., Nowak, W., and Sadowski, J. (2010). Exogenously induced expression of ethylene biosynthesis, ethylene perception, phospholipase D, and Rboh-oxidase genes in broccoli seedlings. J. Exp. Bot. 61, 3475–3491. doi: 10.1093/jxb/erq177
Järup, L. (2003). Hazards of heavy metal contamination. Br. Med. Bull. 68, 167–182. doi: 10.1093/bmb/ldg032
Joo, S., Liu, Y., Lueth, A., and Zhang, S. (2008). MAPK phosphorylation-induced stabilization of ACS6 protein is mediated by the non-catalytic C-terminal domain, which also contains the cis-determinant for rapid degradation by the 26S proteasome pathway. Plant J. 54, 129–140. doi: 10.1111/j.1365-313X.2008.03404.x
Jozefczak, M., Remans, T., Vangronsveld, J., and Cuypers, A. (2012). Glutathione is a key player in metal-induced oxidative stress defenses. Int. J. Mol. Sci. 13, 3145–3175. doi: 10.3390/ijms13033145
Ju, C., and Chang, C. (2012). Advances in ethylene signalling: protein complexes at the endoplasmic reticulum membrane. AoB Plants 2012:pls031. doi: 10.1093/aobpla/pls031
Ju, C., Yoon, G. M., Shemansky, J. M., Lin, D. Y., Ying, I., Chang, J., et al. (2012). CTR1 phosphorylates the central regulator EIN2 to control ethylene hormone signaling from the ER membrane to the nucleus in Arabidopsis. Proc. Natl. Acad. Sci. U.S.A. 109, 19486–19491. doi: 10.1073/pnas.1214848109
Kacperska, A. (2004). Sensor types in signal transduction pathways in plant cells responding to abiotic stressors: do they depend on stress intensity? Physiol. Plant. 122, 159–168. doi: 10.1111/j.0031-9317.2004.00388.x
Keunen, E., Schellingen, K., Van Der Straeten, D., Remans, T., Colpaert, J., Vangronsveld, J., et al. (2015). ALTERNATIVE OXIDASE1a modulates the oxidative challenge during moderate Cd exposure in Arabidopsis thaliana leaves. J. Exp. Bot. 66, 2967–2977. doi: 10.1093/jxb/erv035
Khan, M. I. R., and Khan, N. A. (2014). Ethylene reverses photosynthetic inhibition by nickel and zinc in mustard through changes in PS II activity, photosynthetic nitrogen use efficiency, and antioxidant metabolism. Protoplasma 251, 1007–1019. doi: 10.1007/s00709-014-0610-7
Khan, M. I. R., Nazir, F., Asgher, M., Per, T. S., and Khan, N. A. (2015). Selenium and sulfur influence ethylene formation and alleviate cadmium-induced oxidative stress by improving proline and glutathione production in wheat. J. Plant Physiol. 173, 9–18. doi: 10.1016/j.jplph.2014.09.011
Kieber, J. J., Rothenberg, M., Roman, G., Feldmann, K. A., and Ecker, J. R. (1993). CTR1, a negative regulator of the ethylene response pathway in Arabidopsis, encodes a member of the Raf family of protein kinases. Cell 72, 427–441. doi: 10.1016/0092-8674(93)90119-B
Kim, J., Patterson, S. E., and Binder, B. M. (2013). Reducing jasmonic acid levels causes ein2 mutants to become ethylene responsive. FEBS Lett. 587, 226–230. doi: 10.1016/j.febslet.2012.11.030
Kim, Y. S., Choi, D., Lee, M. M., Lee, S. H., and Kim, W. T. (1998). Biotic and abiotic stress-related expression of 1-aminocyclopropane-1-carboxylate oxidase gene family in Nicotiana glutinosa L. Plant Cell Physiol. 39, 565–573. doi: 10.1093/oxfordjournals.pcp.a029406
Kohli, A., Sreenivasulu, N., Lakshmanan, P., and Kumar, P. P. (2013). The phytohormone crosstalk paradigm takes center stage in understanding how plants respond to abiotic stresses. Plant Cell Rep. 32, 945–957. doi: 10.1007/s00299-013-1461-y
Lei, X. -Y., Zhu, R. -Y., Zhang, G. -Y., and Dai, Y -R. (2003). Possible involvement of the mitochondrial alternative pathway in ethylene-induced apoptosis in tomato protoplasts. Plant Growth Regul. 41, 111–116. doi: 10.1023/A:1027355502538
Lequeux, H., Hermans, C., Lutts, S., and Verbruggen, N. (2010). Response to copper excess in Arabidopsis thaliana: impact on the root system architecture, hormone distribution, lignin accumulation and mineral profile. Plant Physiol. Biochem. 48, 673–682. doi: 10.1016/j.plaphy.2010.05.005
Li, G., Meng, X., Wang, R., Mao, G., Han, L., Liu, Y., et al. (2012). Dual-level regulation of ACC synthase activity by MPK3/MPK6 cascade and its downstream WRKY transcription factor during ethylene induction in Arabidopsis. PLoS Genet. 8:e1002767. doi: 10.1371/journal.pgen.1002767
Liang, X., Shen, N. F., and Theologis, A. (1996). Li+-regulated 1-aminocyclopropane-1-carboxylate synthase gene expression in Arabidopsis thaliana. Plant J. 10, 1027–1036. doi: 10.1046/j.1365-313X.1996.10061027.x
Lin, Z., Zhong, S., and Grierson, D. (2009). Recent advances in ethylene research. J. Exp. Bot. 60, 3311–3336. doi: 10.1093/jxb/erp204
Liu, X. M., Kim, K. E., Kim, K. C., Nguyen, X. C., Han, H. J., Jung, M. S., et al. (2010). Cadmium activates Arabidopsis MPK3 and MPK6 via accumulation of reactive oxygen species. Phytochemistry 71, 614–618. doi: 10.1016/j.phytochem.2010.01.005
Liu, Y., and Zhang, S. (2004). Phosphorylation of 1-aminocyclopropane-1-carboxylic acid synthase by MPK6, a stress-responsive mitogen-activated protein kinase, induces ethylene biosynthesis in Arabidopsis. Plant Cell 16, 3386–3399. doi: 10.1105/tpc.104.026609
Lopes, M. S., Iglesia-Turiño, S., Cabrera-Bosquet, L., Serret, M. D., Bort, J., Febrero, A., et al. (2013). Molecular and physiological mechanisms associated with root exposure to mercury in barley. Metallomics 5, 1305–1315. doi: 10.1039/c3mt00084b
Lu, W. P., and Kirkham, M. B. (1991). Genotypic tolerance to metals as indicated by ethylene production. Water Air Soil Pollut. 57–58, 605–615. doi: 10.1007/BF00282924
Lynch, J., and Brown, K. M. (1997). Ethylene and plant responses to nutritional stress. Physiol. Plant. 100, 613–619. doi: 10.1111/j.1399-3054.1997.tb03067.x
Maksymiec, W. (2011). Effects of jasmonate and some other signalling factors on bean and onion growth during the initial phase of cadmium action. Biol. Plant. 55, 112–118. doi: 10.1007/s10535-011-0015-9
Maksymiec, W., and Krupa, Z. (2006). The effects of short-term exposition to Cd, excess Cu ions and jasmonate on oxidative stress appearing in Arabidopsis thaliana. Environ. Exp. Bot. 57, 187–194. doi: 10.1016/j.envexpbot.2005.05.006
Maksymiec, W., Wianowska, D., Dawidowicz, A. L., Radkiewicz, S., Mardarowicz, M., and Krupa, Z. (2005). The level of jasmonic acid in Arabidopsis thaliana and Phaseolus coccineus plants under heavy metal stress. J. Plant Physiol. 162, 1338–1346. doi: 10.1016/j.jplph.2005.01.013
Masood, A., Iqbal, N., and Khan, N. A. (2012). Role of ethylene in alleviation of cadmium-induced photosynthetic capacity inhibition by sulphur in mustard. Plant Cell Environ. 35, 524–533. doi: 10.1111/j.1365-3040.2011.02432.x
Mazorra Morales, L. M., Senn, M. E., Grozeff, G. E. G., Fanello, D. D., Carrión, C. A., Núñez, M., et al. (2014). Impact of brassinosteroids and ethylene on ascorbic acid accumulation in tomato leaves. Plant Physiol. Biochem. 74, 315–322. doi: 10.1016/j.plaphy.2013.11.021
McDonnell, L., Plett, J. M., Andersson-Gunnerås, S., Kozela, C., Dugardeyn, J., Van Der Straeten, D., et al. (2009). Ethylene levels are regulated by a plant encoded 1-aminocyclopropane-1-carboxylic acid deaminase. Physiol. Plant. 136, 94–109. doi: 10.1111/j.1399-3054.2009.01208.x
Merchante, C., Alonso, J. M., and Stepanova, A. N. (2013). Ethylene signaling: simple ligand, complex regulation. Curr. Opin. Plant Biol. 16, 554–560. doi: 10.1016/j.pbi.2013.08.001
Mersmann, S., Bourdais, G., Rietz, S., and Robatzek, S. (2010). Ethylene signaling regulates accumulation of the FLS2 receptor and is required for the oxidative burst contributing to plant immunity. Plant Physiol. 154, 391–400. doi: 10.1104/pp.110.154567
Mertens, J., Vangronsveld, J., Van Der Straeten, D., and Van Poucke, M. (1999). “Effects of copper and zinc on the ethylene production of Arabidopsis thaliana,” in Biology and Biotechnology of the Plant Hormone Ethylene II, eds A. K. Kanellis, C. Chang, H. Klee, A. B. Bleecker, J. C. Pech, and D. Grierson (Dordrecht: Kluwer Academic Publishers), 333–338.
Mhamdi, A., Hager, J., Chaouch, S., Queval, G., Han, Y., Taconnat, L., et al. (2010). Arabidopsis GLUTATHIONE REDUCTASE1 plays a crucial role in leaf responses to intracellular hydrogen peroxide and in ensuring appropriate gene expression through both salicylic acid and jasmonic acid signaling pathways. Plant Physiol. 153, 1144–1160. doi: 10.1104/pp.110.153767
Mittler, R. (2006). Abiotic stress, the field environment and stress combination. Trends Plant Sci. 11, 15–19. doi: 10.1016/j.tplants.2005.11.002
Mittler, R., Vanderauwera, S., Gollery, M., and Van Breusegem, F. (2004). Reactive oxygen gene network of plants. Trends Plant Sci. 9, 490–498. doi: 10.1016/j.tplants.2004.08.009
Mittler, R., Vanderauwera, S., Suzuki, N., Miller, G., Tognetti, V. B., Vandepoele, K., et al. (2011). ROS signaling: the new wave? Trends Plant Sci. 16, 300–309. doi: 10.1016/j.tplants.2011.03.007
Møller, I. M., Jensen, P. E., and Hansson, A. (2007). Oxidative modifications to cellular components in plants. Annu. Rev. Plant Biol. 58, 459–481. doi: 10.1146/annurev.arplant.58.032806.103946
Montero-Palmero, M. B., Martín-Barranco, A., Escobar, C., and Hernández, L. E. (2014a). Early transcriptional responses to mercury: a role for ethylene in mercury-induced stress. New Phytol. 201, 116–130. doi: 10.1111/nph.12486
Montero-Palmero, M. B., Ortega-Villasante, C., Escobar, C., and Hernández, L. E. (2014b). Are plant endogenous factors like ethylene modulators of the early oxidative stress induced by mercury? Front. Environ. Sci. 2:34. doi: 10.3389/fenvs.2014.00034
Mur, L. A. J., Prats, E., Pierre, S., Hall, M. A., and Hebelstrup, K. H. (2013). Integrating nitric oxide into salicylic acid and jasmonic acid/ethylene plant defense pathways. Front. Plant Sci. 4:215. doi: 10.3389/fpls.2013.00215
Murphy, L. J., Robertson, K. N., Harroun, S. G., Brosseau, C. L., Werner-Zwanziger, U., Moilanen, J., et al. (2014). A simple complex on the verge of breakdown: Isolation of the elusive cyanoformate ion. Science 344, 75–78. doi: 10.1126/science.1250808
Nagarajan, V. K., and Smith, A. P. (2011). Ethylene's role in phosphate starvation signaling: more than just a root growth regulator. Plant Cell Physiol. 53, 277–286. doi: 10.1093/pcp/pcr186
Naranjo, M. A., Romero, C., Bellés, J. M., Montesinos, C., Vicente, O., and Serrano, R. (2003). Lithium treatment induces a hypersensitive-like response in tobacco. Planta 217, 417–424. doi: 10.1007/s00425-003-1017-4
Neljubov, D. (1901). Ueber die horizontale Nutation der Stengel von Pisum sativum und einiger anderen Pflanzen. Beiheifte Bot. Zentralblatt 10, 128–139.
Opdenakker, K., Remans, T., Vangronsveld, J., and Cuypers, A. (2012). Mitogen-activated protein (MAP) kinases in plant metal stress: regulation and responses in comparison to other biotic and abiotic stresses. Int. J. Mol. Sci. 13, 7828–7853. doi: 10.3390/ijms13067828
Ouaked, F., Rozhon, W., Lecourieux, D., and Hirt, H. (2003). A MAPK pathway mediates ethylene signaling in plants. EMBO J. 22, 1282–1288. doi: 10.1093/emboj/cdg131
Overmyer, K., Brosché, M., and Kangasjärvi, J. (2003). Reactive oxygen species and hormonal control of cell death. Trends Plant Sci. 8, 335–342. doi: 10.1016/S1360-1385(03)00135-3
Peng, H.-P., Lin, T.-Y., Wang, N.-N., and Shih, M.-C. (2005). Differential expression of genes encoding 1-aminocyclopropane-1-carboxylate synthase in Arabidopsis during hypoxia. Plant Mol. Biol. 58, 15–25. doi: 10.1007/s11103-005-3573-4
Petrov, V. D., and Van Breusegem, F. (2012). Hydrogen peroxide - a central hub for information flow in plant cells. AoB Plants 2012, pls014. doi: 10.1093/aobpla/pls014
Pierik, R., Tholen, D., Poorter, H., Visser, E. J. W., and Voesenek, L. A. C. J. (2006). The Janus face of ethylene: growth inhibition and stimulation. Trends Plant Sci. 11, 176–183. doi: 10.1016/j.tplants.2006.02.006
Qiao, H., Chang, K. N., Yazaki, J., and Ecker, J. R. (2009). Interplay between ethylene, ETP1/ETP2 F-box proteins, and degradation of EIN2 triggers ethylene responses in Arabidopsis. Genes Dev. 23, 512–521. doi: 10.1101/gad.1765709
Remans, T., Opdenakker, K., Smeets, K., Mathijsen, D., Vangronsveld, J., and Cuypers, A. (2010). Metal-specific and NADPH oxidase dependent changes in lipoxygenase and NADPH oxidase gene expression in Arabidopsis thaliana exposed to cadmium or excess copper. Funct. Plant Biol. 37, 532–544. doi: 10.1071/FP09194
Remans, T., Thijs, S., Truyens, S., Weyens, N., Schellingen, K., Keunen, E., et al. (2012). Understanding the development of roots exposed to contaminants and the potential of plant-associated bacteria for optimization of growth. Ann. Bot. 110, 239–252. doi: 10.1093/aob/mcs105
Resnick, J. S., Rivarola, M., and Chang, C. (2008). Involvement of RTE1 in conformational changes promoting ETR1 ethylene receptor signaling in Arabidopsis. Plant J. 56, 423–431. doi: 10.1111/j.1365-313X.2008.03615.x
Resnick, J. S., Wen, C. -K., Shockey, J. A., and Chang, C. (2006). REVERSION-TO-ETHYLENE SENSITIVITY1, a conserved gene that regulates ethylene receptor function in Arabidopsis. Proc. Natl. Acad. Sci. U.S.A. 103, 7917–7922. doi: 10.1073/pnas.0602239103
Rodríguez-Serrano, M., Romero-Puertas, M. C., Pazmiño, D. M., Testillano, P. S., Risueño, M. C., del Río, L. A., et al. (2009). Cellular response of pea plants to cadmium toxicity: Cross talk between reactive oxygen species, nitric oxide, and calcium. Plant Physiol. 150, 229–243. doi: 10.1104/pp.108.131524
Rodríguez-Serrano, M., Romera-Puertas, M. C., Zabalza, A., Corpas, F. J., Gómez, M., Del Río, L. A., et al. (2006). Cadmium effect on oxidative metabolism of pea (Pisum sativum L.) roots. Imaging of reactive oxygen species and nitric oxide accumulation in vivo. Plant Cell Environ. 29, 1532–1534.
Ruduś, I., Sasiak, M., and Kêpczyñski, J. (2012). Regulation of ethylene biosynthesis at the level of 1-aminocyclopropane-1-carboxylate oxidase (ACO) gene. Acta Physiol. Plant. 35, 295–307. doi: 10.1007/s11738-012-1096-6
Schellingen, K., Van Der Straeten, D., Remans, T., Loix, C., Vangronsveld, J., and Cuypers, A. (2015a). Ethylene biosynthesis is involved in the early oxidative challenge induced by moderate Cd exposure in Arabidopsis thaliana. Environ. Exp. Bot. 117, 1–11. doi: 10.1016/j.envexpbot.2015.04.005
Schellingen, K., Van Der Straeten, D., Remans, T., Vangronsveld, J., Keunen, E., and Cuypers, A. (2015b). Ethylene signalling is mediating the early cadmium-induced oxidative challenge in Arabidopsis thaliana. Plant Sci. 239, 137–146. doi: 10.1016/j.plantsci.2015.07.015
Schellingen, K., Van Der Straeten, D., Vandenbussche, F., Prinsen, E., Remans, T., Vangronsveld, J., et al. (2014). Cadmium-induced ethylene production and responses in Arabidopsis thaliana rely on ACS2 and ACS6 gene expression. BMC Plant Biol. 14:214. doi: 10.1186/s12870-014-0214-6
Schnaubelt, D., Queval, G., Dong, Y., Diaz-Vivancos, P., Makgopa, M. E., Howell, G., et al. (2015). Low glutathione regulates gene expression and the redox potentials of the nucleus and cytosol in Arabidopsis thaliana. Plant Cell Environ. 38, 266–279. doi: 10.1111/pce.12252
Schützendübel, A., and Polle, A. (2002). Plant responses to abiotic stresses: heavy metal-induced oxidative stress and protection by mycorrhization. J. Exp. Bot. 53, 1351–1365. doi: 10.1093/jexbot/53.372.1351
Seth, C. S., Remans, T., Keunen, E., Jozefczak, M., Gielen, H., Opdenakker, K., et al. (2012). Phytoextraction of toxic metals: a central role for glutathione. Plant Cell Environ. 35, 334–346. doi: 10.1111/j.1365-3040.2011.02338.x
Shakeel, S. N., Gao, Z., Amir, M., Chen, Y.-F., Rai, M. I., Haq, N. U., et al. (2015). Ethylene regulates levels of ethylene receptor/CTR1 signaling complexes in Arabidopsis thaliana. J. Biol. Chem. 290, 12415–12424. doi: 10.1074/jbc.M115.652503
Sharma, S. S., and Dietz, K.-J. (2009). The relationship between metal toxicity and cellular redox imbalance. Trends Plant Sci. 14, 43–50. doi: 10.1016/j.tplants.2008.10.007
Skottke, K. R., Yoon, G. M., Kieber, J. J., and DeLong, A. (2011). Protein phosphatase 2A controls ethylene biosynthesis by differentially regulating the turnover of ACC synthase isoforms. PLoS Genet. 7:e1001370. doi: 10.1371/journal.pgen.1001370
Song, S., Qi, T., Wasternack, C., and Xie, D. (2014). Jasmonate signaling and crosstalk with gibberellin and ethylene. Curr. Opin. Plant Biol. 21, 112–119. doi: 10.1016/j.pbi.2014.07.005
Srivastava, A. K., Venkatachalam, P., Raghothama, K. G., and Sahi, S. V. (2007). Identification of lead-regulated genes by suppression subtractive hybridization in the heavy metal accumulator Sesbania drummondii. Planta 225, 1353–1365. doi: 10.1007/s00425-006-0445-3
Steffens, B. (2014). The role of ethylene and ROS in salinity, heavy metal, and flooding responses in rice. Front. Plant Sci. 5:685. doi: 10.3389/fpls.2014.00685
Sun, P., Tian, Q.-Y., Chen, J., and Zhang, W.-H. (2010). Aluminium-induced inhibition of root elongation in Arabidopsis is mediated by ethylene and auxin. J. Exp. Bot. 61, 347–356. doi: 10.1093/jxb/erp306
Sun, P., Tian, Q.-Y., Zhao, M.-G., Dai, X.-Y., Huang, J.-H., Li, L.-H., et al. (2007). Aluminum-induced ethylene production is associated with inhibition of root elongation in Lotus japonicus L. Plant Cell Physiol. 48, 1229–1235. doi: 10.1093/pcp/pcm077
Sun, X., and Guo, L. (2013). Relationship between cadmium-induced root subapical hair development and ethylene biosynthesis in oilseed rape seedlings. Acta Biol. Cracoviensia Ser. Bot. 55, 68–75. doi: 10.2478/abcsb-2013-0018
Suzuki, N., Miller, G., Morales, J., Shulaev, V., Torres, M. A., and Mittler, R. (2011). Respiratory burst oxidases: the engines of ROS signaling. Curr. Opin. Plant Biol. 14, 691–699. doi: 10.1016/j.pbi.2011.07.014
Takahashi, F., Yoshida, R., Ichimura, K., Mizoguchi, T., Seo, S., Yonezawa, M., et al. (2007). The mitogen-activated protein kinase cascade MKK3-MPK6 is an important part of the jasmonate signal transduction pathway in Arabidopsis. Plant Cell 19, 805–818. doi: 10.1105/tpc.106.046581
Thain, S. C., Vandenbussche, F., Laarhoven, L. J. J., Dowson-Day, M. J., Wang, Z.-Y., Tobin, E. M., et al. (2004). Circadian rhythms of ethylene emission in Arabidopsis. Plant Physiol. 136, 3751–3761. doi: 10.1104/pp.104.042523
Thao, N. P., Khan, M. I. R., Binh, N., Thu, A., Lan, X., Hoang, T., et al. (2015). Role of ethylene and its cross talk with other signaling molecules in plant responses to heavy metal stress. Plant Cell Environ. 169, 73–84. doi: 10.1104/pp.15.00663
Tian, Q., Zhang, X., Ramesh, S., Gilliham, M., Tyerman, S. D., and Zhang, W.-H. (2014). Ethylene negatively regulates aluminium-induced malate efflux from wheat roots and tobacco cells transformed with TaALMT1. J. Exp. Bot. 65, 2415–2426. doi: 10.1093/jxb/eru123
Trinh, N.-N., Huang, T.-L., Chi, W.-C., Fu, S.-F., Chen, C.-C., and Huang, H.-J. (2014). Chromium stress response effect on signal transduction and expression of signaling genes in rice. Physiol. Plant. 150, 205–224. doi: 10.1111/ppl.12088
Tsuchisaka, A., Yu, G., Jin, H., Alonso, J. M., Ecker, J. R., Zhang, X., et al. (2009). A combinatorial interplay among the 1-aminocyclopropane-1-carboxylate isoforms regulates ethylene biosynthesis in Arabidopsis thaliana. Genetics 183, 979–1003. doi: 10.1534/genetics.109.107102
Vahala, J., Schlagnhaufer, C. D., and Pell, E. J. (1998). Induction of an ACC synthase cDNA by ozone in light-grown Arabidopsis thaliana leaves. Physiol. Plant. 103, 45–50. doi: 10.1034/j.1399-3054.1998.1030106.x
Vandenbussche, F., Vaseva, I., Vissenberg, K., and Van Der Straeten, D. (2012). Ethylene in vegetative development: a tale with a riddle. New Phytol. 194, 895–909. doi: 10.1111/j.1469-8137.2012.04100.x
Vandenbussche, F., Vriezen, W. H., Smalle, J., Laarhoven, L. J. J., Harren, F. J. M., and Van Der Straeten, D. (2003). Ethylene and auxin control the Arabidopsis response to decreased light intensity. Plant Physiol. 133, 517–527. doi: 10.1104/pp.103.022665
Van de Poel, B., Bulens, I., Hertog, M. L., Nicolai, B. M., and Geeraerd, A. H. (2014). A transcriptomics-based kinetic model for ethylene biosynthesis in tomato (Solanum lycopersicum) fruit: development, validation and exploration of novel regulatory mechanisms. New Phytol. 202, 952–963. doi: 10.1111/nph.12685
Van de Poel, B., Bulens, I., Markoula, A., Hertog, M. L., Dreesen, R., Wirtz, M., et al. (2012). Targeted systems biology profiling of tomato fruit reveals coordination of the Yang cycle and a distinct regulation of ethylene biosynthesis during postclimacteric ripening. Plant Physiol. 160, 1498–1514. doi: 10.1104/pp.112.206086
Van de Poel, B., Smet, D., and Van Der Straeten, D. (2015). Ethylene and hormonal crosstalk in vegetative growth and development. Plant Physiol. 169, 61–72. doi: 10.1104/pp.15.00724
Van de Poel, B., and Van Der Straeten, D. (2014). 1-aminocyclopropane-1-carboxylic acid (ACC) in plants: more than just the precursor of ethylene! Front. Plant Sci. 5:640. doi: 10.3389/fpls.2014.00640
Vangronsveld, J., Herzig, R., Weyens, N., Boulet, J., Adriaensen, K., Ruttens, A., et al. (2009). Phytoremediation of contaminated soils and groundwater: lessons from the field. Environ. Sci. Pollut. Res. Int. 16, 765–794. doi: 10.1007/s11356-009-0213-6
Vassilev, A., Lidon, F., Scotti, P., Da Graca, M., and Yordanov, I. (2004). Cadmium-induced changes in chloroplast lipids and photosystem activities in barley plants. Biol. Plant. 48, 153–156. doi: 10.1023/B:BIOP.0000024295.27419.89
Verbruggen, N., Hermans, C., and Schat, H. (2009). Mechanisms to cope with arsenic or cadmium excess in plants. Curr. Opin. Plant Biol. 12, 364–372. doi: 10.1016/j.pbi.2009.05.001
Wang, H., Liang, X., Wan, Q., Wang, X., and Bi, Y. (2009). Ethylene and nitric oxide are involved in maintaining ion homeostasis in Arabidopsis callus under salt stress. Planta 230, 293–307. doi: 10.1007/s00425-009-0946-y
Weber, M., Trampczynska, A., and Clemens, S. (2006). Comparative transcriptome analysis of toxic metal responses in Arabidopsis thaliana and the Cd2+-hypertolerant facultative metallophyte Arabidopsis halleri. Plant Cell Environ. 29, 950–963. doi: 10.1111/j.1365-3040.2005.01479.x
Weyens, N., van der Lelie, D., Taghavi, S., Newman, L., and Vangronsveld, J. (2009). Exploiting plant-microbe partnerships to improve biomass production and remediation. Trends Biotechnol. 27, 591–598. doi: 10.1016/j.tibtech.2009.07.006
Xu, J., Li, Y., Wang, Y., Liu, H., Lei, L., Yang, H., et al. (2008). Activation of MAPK kinase 9 induces ethylene and camalexin biosynthesis and enhances sensitivity to salt stress in Arabidopsis. J. Biol. Chem. 283, 26996–27006. doi: 10.1074/jbc.M801392200
Xu, S. S., Lin, S. Z., and Lai, Z. X. (2015). Cadmium impairs iron homeostasis in Arabidopsis thaliana by increasing the polysaccharide contents and the iron-binding capacity of root cell walls. Plant Soil 392, 71–85. doi: 10.1007/s11104-015-2443-3
Yakimova, E. T., Kapchina-Toteva, V. M., and Woltering, E. J. (2007). Signal transduction events in aluminum-induced cell death in tomato suspension cells. J. Plant Physiol. 164, 702–708. doi: 10.1016/j.jplph.2006.03.018
Yamagami, T., Tsuchisaka, A., Yamada, K., Haddon, W. F., Harden, L. A., and Theologis, A. (2003). Biochemical diversity among the 1-amino-cyclopropane-1-carboxylate synthase isozymes encoded by the Arabidopsis gene family. J. Biol. Chem. 278, 49102–49112. doi: 10.1074/jbc.M308297200
Yamauchi, M., and Peng, X. X. (1995). Iron toxicity and stress-induced ethylene production in rice leaves. Plant Soil 173, 21–28. doi: 10.1007/BF00155514
Yang, S. F., and Hoffman, N. E. (1984). Ethylene biosynthesis and its regulation in higher plants. Annu. Rev. Plant Physiol. 35, 155–189. doi: 10.1146/annurev.pp.35.060184.001103
Yoo, S.-D., Cho, Y., and Sheen, J. (2009). Emerging connections in the ethylene signaling network. Trends Plant Sci. 14, 270–279. doi: 10.1016/j.tplants.2009.02.007
Yoo, S.-D., Cho, Y.-H., Tena, G., Xiong, Y., and Sheen, J. (2008). Dual control of nuclear EIN3 by bifurcate MAPK cascades in C2H4 signalling. Nature 451, 789–795. doi: 10.1038/nature06543
Yoon, G. M., and Kieber, J. J. (2013). 1-Aminocyclopropane-1-carboxylic acid as a signalling molecule in plants. AoB Plants 5, plt017. doi: 10.1093/aobpla/plt017
Yoshida, S., Tamaoki, M., Ioki, M., Ogawa, D., Sato, Y., Aono, M., et al. (2009). Ethylene and salicylic acid control glutathione biosynthesis in ozone-exposed Arabidopsis thaliana. Physiol. Plant. 136, 284–298. doi: 10.1111/j.1399-3054.2009.01220.x
Zhang, Y., He, Q., Zhao, S., Huang, L., and Hao, L. (2014). Arabidopsis ein2-1 and npr1-1 response to Al stress. Bull. Environ. Contam. Toxicol. 93, 78–83. doi: 10.1007/s00128-014-1249-y
Zhao, Q., and Guo, H.-W. (2011). Paradigms and paradox in the ethylene signaling pathway and interaction network. Mol. Plant 4, 626–634. doi: 10.1093/mp/ssr042
Keywords: ethylene, metals, oxidative stress, signal transduction, crosstalk
Citation: Keunen E, Schellingen K, Vangronsveld J and Cuypers A (2016) Ethylene and Metal Stress: Small Molecule, Big Impact. Front. Plant Sci. 7:23. doi: 10.3389/fpls.2016.00023
Received: 31 August 2015; Accepted: 08 January 2016;
Published: 02 February 2016.
Edited by:
Francisco Javier Romera, University of Córdoba, SpainReviewed by:
Agnieszka Sirko, Polish Academy of Sciences, PolandCopyright © 2016 Keunen, Schellingen, Vangronsveld and Cuypers. This is an open-access article distributed under the terms of the Creative Commons Attribution License (CC BY). The use, distribution or reproduction in other forums is permitted, provided the original author(s) or licensor are credited and that the original publication in this journal is cited, in accordance with accepted academic practice. No use, distribution or reproduction is permitted which does not comply with these terms.
*Correspondence: Els Keunen, ZWxzLmtldW5lbkB1aGFzc2VsdC5iZQ==
Disclaimer: All claims expressed in this article are solely those of the authors and do not necessarily represent those of their affiliated organizations, or those of the publisher, the editors and the reviewers. Any product that may be evaluated in this article or claim that may be made by its manufacturer is not guaranteed or endorsed by the publisher.
Research integrity at Frontiers
Learn more about the work of our research integrity team to safeguard the quality of each article we publish.