- 1National Center for Genetic Engineering and Biotechnology, National Science and Technology Development Agency, Pathum Thani, Thailand
- 2Department of Environment Studies, Panjab University, Chandigarh, India
- 3Research Institute, Meijo University, Nagoya, Japan
Acacia ampliceps (salt wattle), a leguminous shrub, has been introduced in salt-affected areas in the northeast of Thailand for the remediation of saline soils. However, the defense mechanisms underlying salt tolerance A. ampliceps are unknown. We investigated various physio-biochemical and morphological attributes of A. ampliceps in response to varying levels of salt treatment (200–600 mM NaCl). Seedlings of A. ampliceps (25 ± 2 cm in plant height) raised from seeds were treated with 200 mM (mild stress), 400 and 600 mM (extreme stress) of salt treatment (NaCl) under greenhouse conditions. Na+ and Ca2+ contents in the leaf tissues increased significantly under salt treatment, whereas K+ content declined in salt-stressed plants. Free proline and soluble sugar contents in plants grown under extreme salt stress (600 mM NaCl) for 9 days significantly increased by 28.7 (53.33 μmol g–1 FW) and 3.2 (42.11 mg g–1 DW) folds, respectively over the control, thereby playing a major role as osmotic adjustment. Na+ enrichment in the phyllode tissues of salt-stressed seedlings positively related to total chlorophyll (TC) degradation (R2 = 0.72). Photosynthetic pigments and chlorophyll fluorescence in salt-stressed plants increased under mild salt stress (200 mM NaCl). However, these declined under high levels of salinity (400–600 mM NaCl), consequently resulting in a reduced net photosynthetic rate (R2 = 0.81) and plant dry weight (R2 = 0.91). The study concludes that A. ampliceps has an osmotic adjustment and Na+ compartmentation as effective salt defense mechanisms, and thus it could be an excellent species to grow in salt-affected soils.
Introduction
Soil salinity is one of the major abiotic stresses that severely affect plant growth, development, and produce. It affects nearly 397 million hectares (Mha) of land world over, particularly in Asia-Pacific and Australia where around 195 Mha is salt-affected (Flowers et al., 1986; Rengasamy, 2006). Halophytic (salt-tolerant) species constituting only 1% of the world’s flora have evolved salt-defense mechanisms and show optimal growth in salt-affected soil (Flowers and Colmer, 2008). Such salt tolerance mechanisms include ion homeostasis system via salt glands/salt bladders, osmoregulation system such as free proline, glycinebetaine, mannitol and soluble sugars, hormonal regulation, and antioxidant machinery (Hasegawa et al., 2000; Flowers et al., 2010; Shabala, 2013; Shabala et al., 2014). Na+ homeostasis via antiporter proteins, i.e., Na+/H+ exchanger (NHXs) in halophytic species has been well established as a major channel to manage the Na+ influx from the soil solution into root cell and translocate via xylem loading to other organs, leading to increased Na+/K+ ratio (Flowers and Colmer, 2008; Li et al., 2008). Alternatively, free proline and total soluble sugar enrichments in halophytic plants have been reported as major osmolytes when plants are exposed to soil salinity (Yokota, 2003; Patel et al., 2010; Theerawitaya et al., 2014). In the case of physiological changes, photosynthetic pigment, i.e., chlorophyll a and b degrades depending on the degree of salt levels in the soil solution, especially in extreme salt stress (10 dS m–1) (Giri et al., 2003), causing to reduce net photosynthetic rate, especially in extreme salt stress (Takemura et al., 2000).
Halophytes have been regarded as potential new crops for use as forage, vegetable, and oilseed crop. However, the potential utilization of halophytic species to grow in salt-affected soil and to facilitate saline soil phytoremediation depends on several factors such as salt accumulation, relative growth rate and biomass conversion, multipurpose utilization, and economic returns to the farmers (Panta et al., 2014). Acacia ampliceps (salt wattle), a native of Australia, is a multipurpose plant species largely used for livestock feeding (based on 120–170 g kg–1 crude protein; Dynes and Schlink, 2002) and biomass production (23.34% dry biomass; Ashraf et al., 2012). In addition, it has the potential for saline soil remediation and grows well in the soil with ECe ≥ 16 dS m–1 (Ansari et al., 2007). Previously, using multivariate cluster analyses of biomass productivity of Acacia species in salt-affected soil, A. ampliceps or salt wattle has been found to have the maximum biomass production (39.69 ton ha–1) than the other species: A. karroo (2.39 ton ha–1), A. stenophylla (0.53 ton ha–1), A. seyal (0.27 ton ha–1), and A. asak (0.18 ton ha–1) (Aref et al., 2003). In Thailand, A. ampliceps, a leguminous shrub, has been introduced in salt-affected areas in the northeast of Thailand for the remediation of saline soils (Wongpokkhom et al., 2008). However, the defense mechanisms underlying salt tolerance in A. ampliceps are largely unknown. However, no study has been conducted to investigate physiological and biochemical mechanisms underlying salt tolerance mechanisms in A. ampliceps. We, therefore, conducted a series of experiments to investigate the biochemical, physiological, and morphological attributes of A. ampliceps grown in response to extreme salt concentrations under greenhouse conditions.
Materials and Methods
Plant Material and Salt Treatments
Seeds of salt wattle (Acacia ampliceps Maslin) provided by Land Development Department, Ministry of Agriculture and Cooperatives, Thailand, were disinfected with 15% sodium hypochlorite for 30 min followed by washing three times with tap water and then with distilled water. Disinfected seeds were germinated in the plastic tray (30 × 60 × 5 cm) containing 1 kg of garden soil (sandy loam; EC = 2.687 dS m–1; pH = 5.5; organic matter = 10.36%; total nitrogen = 0.17%; total phosphorus = 0.07%; total potassium = 1.19%) under greenhouse conditions. 15-day old seedlings were transferred into plastic bag (W × L × H; 4 × 4 × 12 cm) containing garden soil (as above) and grown under controlled environmental conditions (80 ± 5% relative humidity, 500–1,000 μmol m–2 s–1 photosynthetic photon flux density, 10 ± 2 h d–1 photoperiod from sunlight and 28 ± 2°C ambient temperature) for 7 weeks. The experiment was conducted in the greenhouse of the Thailand Science Park, Pathum Thani, Thailand (Latitude 14°01′12″N Longitude 100°31′12″E).
Uniform-sized seedlings (25 ± 2 cm in plant height) were selected as initial material. Sodium chloride (NaCl) concentration in the soil solution was adjusted to 0 (control), 200 (mild stress), 400, and 600 mM (high stress). After 9 days, growth characters, Na+, K+, and Ca2+ contents, soluble sugar content, free proline content, photosynthetic pigments, chlorophyll fluorescence, net photosynthetic rate (Pn), stomatal conductance (gs), and transpiration rate (E) in the phyllodes were measured (n = 6).
Growth Performances
Shoot height (SH), root length (RL), number of phyllodes (NL), number of roots (NR), fresh weight (FW), dry weight (DW), and phyllode leaf area (LA) of seedlings were measured. Salt wattle seedlings were dried at 80°C in a hot-air oven for 2 days and then incubated in desiccator before the measurement of DW. Phyllode area was measured using a Root/Leaf Area Meter DT-scan (Delta-Scan Version 2.03, Delta-T Devices, Ltd, Cambridge, UK).
Na+, K+, and Ca2+ Assay
Na+, K+, and Ca2+ were assayed following the modified method of Tanaka et al. (1999) and Hossain et al. (2006). In brief, phyllode tissues of salt-stressed seedlings were washed by deionized water to remove surface contaminating Na+. The tissues were ground into powder in liquid nitrogen, extracted with boiling distilled water, and centrifuged at 10,000 × g for 10 min. The supernatant was filtered through a 0.45 μm membrane filter (VertiPure™, Vertical®). Cellular Na+, K+, and Ca2+ concentrations were determined using HPLC (Waters Associates, Millford, MA, USA) coupled with 432 Conductivity Detector and WATER IC-PACK™ ion-exclusion column (Waters Associates, Millford, MA, USA). Nanopure water was used as mobile phase with 0.6 mL min–1 flow rate. Na+, K+, and Ca2+ (Sigma, USA) were used as standard.
Total Soluble Sugars Determination
Total soluble sugars (sucrose, glucose, and fructose) in the second fully expanded phyllode (from shoot tip) were analyzed according to the modified method of Karkacier et al. (2003). In a pre-cooled mortar, 100 mg tissue was ground with liquid nitrogen, extracted with 1 mL of deionized water, vigorously shaken for 15 s, sonicated for 15 min and then centrifuged at 12,000 rpm for 15 min. The supernatant was filtered through a 0.45 μm membrane filter (VertiPure™, Vertical®) and stored at –20°C prior to the measurement of total soluble sugars content using HPLC. A volume of 40 μL of crude extracts was automatically injected into a Waters HPLC fitted with a Waters 600 pump using a MetaCarb 87°C column equipped with a guard column. Deionised water was used as the mobile phase at a flow rate of 0.5 mL min–1. The online detection was performed using a Waters 410 differential refractrometer detector, and the data was analyzed by Empower® software. Sucrose, glucose, and fructose (Fluka, USA) were used as standards.
Free Proline Assay
Free proline in the second fully expanded phyllode (from shoot tip) was extracted and analyzed according to the method of Bates et al. (1973). Fifty milligram of fresh material was ground with liquid nitrogen in a mortar. The homogenate powder was mixed with 1 mL of aqueous sulfosalicylic acid (3%, w/v), and the solution was filtered through filter paper (Whatman No 1, England). The extracted solution was reacted with an equal volume of glacial acetic acid and ninhydrin reagent (1.25 mg ninhydrin in 30 mL glacial acetic acid and 20 mL 6 M H3PO4) and incubated at 95°C for 1 h. The reaction was terminated by placing the container in an ice bath. The reaction mixture was mixed vigorously with 2 mL of toluene. After cooling to 25°C, the chromophore was measured at 520 nm by UV-VIS spectro-photometer (HACH DR/4000; Model 48000, HACH Company, Loveland, CO, USA) using L-proline as a calibration standard.
Photosynthetic Pigments
Chlorophyll a (Chla), chlorophyll b (Chlb), and TC contents in the second fully expanded phyllode were analyzed following the method of Shabala et al. (1998). 100 milligram of phyllode tissue was homogenized in glass vials using 10 mL of 95.5% acetone and blended using a homogenizer. The glass vials were sealed with parafilm® to prevent evaporation and then stored at 4°C for 48 h. Chla and Chlb concentrations were measured at 662 nm and 644 nm using UV-VIS spectrophotometer against acetone (95.5%) as a blank.
Chlorophyll Fluorescence
Chlorophyll fluorescence emission was measured from the adaxial surface of the second fully expanded phyllode using a fluorescence monitoring system (FMS 2; Hansatech Instruments Ltd., Norfolk, UK) in the pulse amplitude modulation mode (Loggini et al., 1999). A phyllode, kept in dark for 30 min, was initially exposed to the modulated measuring beam of far-red light (LED source with typical peak at wavelength 735 nm). Original (F0) and maximum (Fm) fluorescence yields were measured under weak modulated red light (<0.5 μmol m–2 s–1) with 1.6 s pulses of saturating light (>6.8 μmol m–2 s–1 PAR) and calculated using FMS software for Windows®. The variable fluorescence yield (Fv) was calculated using the equation: Fv = Fm–F0. The ratio of variable to maximum fluorescence (Fv/Fm) was calculated as the maximum quantum yield of PSII photochemistry. The photon yield of PSII (ΦPSII) in the light was calculated as: ΦPSII = (Fm′-F)/Fm′ after 45 s of illumination, when steady state was achieved (Maxwell and Johnson, 2000).
Net Photosynthetic Rate (Pn), Stomatal Conductance (gs) and Transpiration Rate (E)
Net photosynthetic rate (Pn; μmol m–2s–1), stomatal conductance (gs; μmol CO2 m–2 s–1), and transpiration rate (E; mmol m–2 s–1) in the second fully expanded phyllode were measured using a portable photosynthesis system fitted with an infra-red gas analyzer (Model LI 6400, LI-COR® Inc., Lincoln, NE, USA). The gs and E were measured continuously by monitoring the content of the air entering and existing in the IRGA headspace chamber, according to Cha-um et al. (2007). The sample chamber was set at 500 μmol s–1 air-flow rate and 25°C of chamber temperature. The light intensity was adjusted to 1,000 μmol m–2 s–1 PPFD of 6400-02B red-blue LED light source.
Experiment Design and Statistical Analysis
The experiment was arranged as Completely Randomized Block Design (CRBD) with six replicates (n = 6). The mean values obtained were compared using Tukey’s HSD and analyzed by SPSS software.
Results
Morphological and Growth Performances
Leaf color of control (0 mM NaCl) and mild salt-stressed plants (200 mM NaCl) was green. In contrast, phyllodes and the bipinnate leaves (true older leaves) turned yellow in the plants grown under extreme salt stress (400–600 mM NaCl) for 9 days. The overall growth characters declined in plants subjected to extreme salt stress for 9 days (Figure 1). SH, RL, NL, NR, FW, DW, and phyllode LA of salt wattle grown under 200 mM NaCl stress increased; however, these declined significantly when plants were subjected to 400–600 mM NaCl for 9 days (Table 1 and Figures 2A–C). SH, RL, NL, NR, shoot FW, and root FW in extreme salt-stressed (600 mM NaCl) plants were sharply dropped by 25, 23, 60, 45, 78, and 74%, respectively, compared to plants growing under mild stress (200 mM NaCl; Table 1). In addition, the salt-sensitive parameters such as NL, FW, and DW were decreased in plants exposed to 400 mM NaCl for 9 days, similar result to 600 mM. The growth and development of salt wattle plants under mild salt stress were enhanced and better than those under control condition (0 mM NaCl; Table 1).
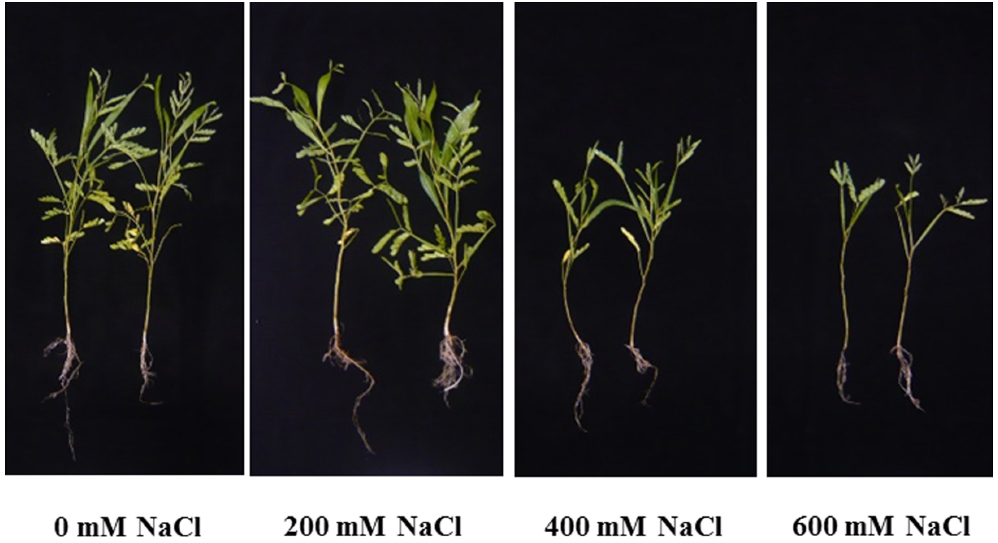
Figure 1. Plant morphological characters of Acacia ampliceps seedlings grown under salt stress conditions for 9 days.

Table 1. Shoot height (SH), root length (RL), number of phyllodes (NL), number of roots (NR), shoot fresh weight (SHFW), and root fresh weight (RTFW) of Acacia ampliceps seedlings grown under salt stress conditions for 9 days.
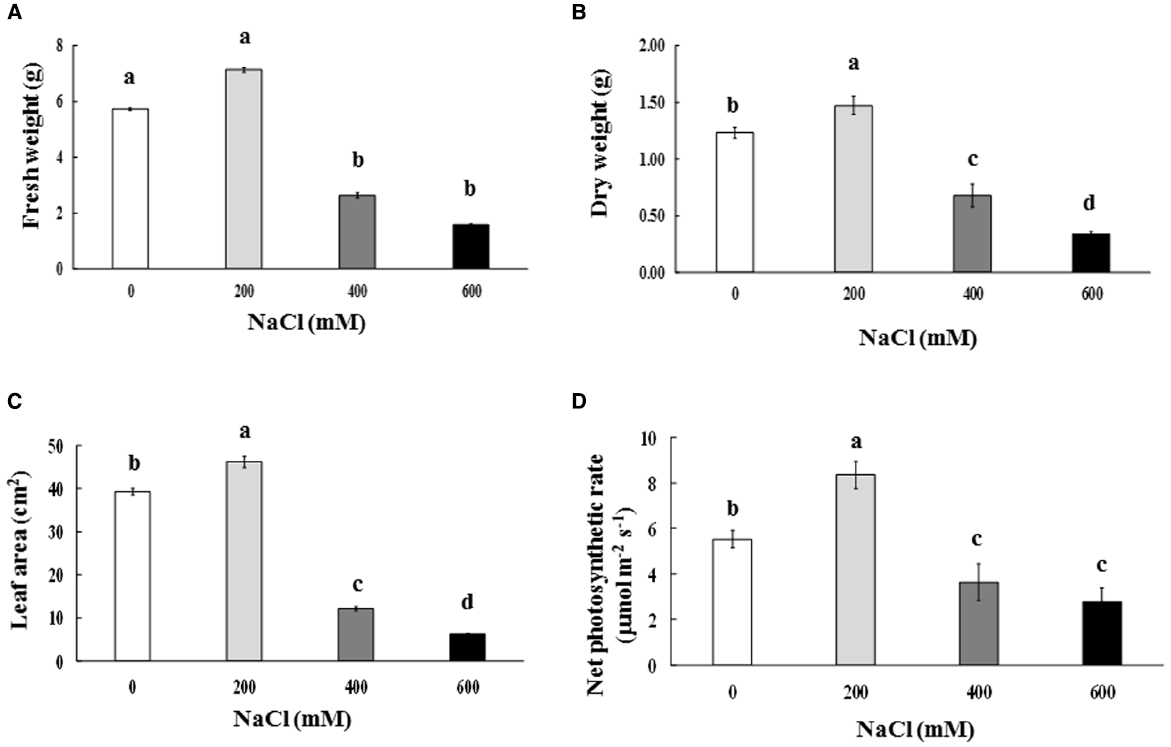
Figure 2. Fresh weight (A), dry weight (B), phyllode leaf area (C), and net photosynthetic rate (D) of Acacia ampliceps seedlings grown under salt stress conditions for 9 days. Data presented as mean ± SE. Different letters represent significant difference at p ≤ 0.01 according to Tukey’s HSD test.
Na+, K+, and Ca2+
Na+ and Ca2+ contents in the phyllode tissues of salt-stressed plants were continuously enriched, whereas K+ content declined significantly, depending on the NaCl concentration in the soil solution (Figures 3A–C). Na+/K+ in the phyllode leaves exhibited a trend similar to Na enrichment when plants were subjected to salt stress (Figure 3D). Under the extreme salt stress (600 mM NaCl), Na+ content in the phyllode tissues was peaked to ∼33 mg g–1 DW, thereby resulting in an increase in Na+/K+ ratio by 7.8 fold (Figure 3). In addition, Na+ and Ca2+ content in the phyllode tissues of mild salt-stressed plants (200 mM NaCl) was 16.1 and 9.5 mg g–1 DW, respectively.
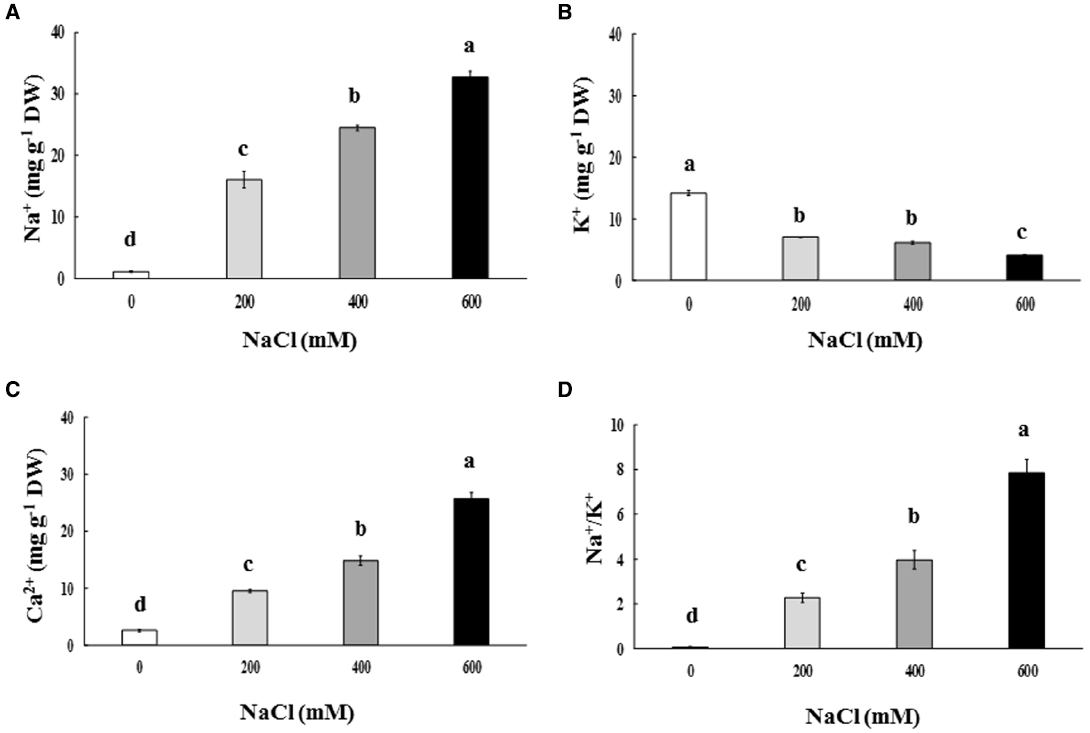
Figure 3. Na+(A), K+(B), and Ca2+ content (C) and Na+/K+ ratio (D) of Acacia ampliceps seedlings grown under salt stress conditions for 9 days. Data presented as mean ± SE. Different letters represent significant difference at p ≤ 0.01 according to Tukey’s HSD test.
Free Proline and Total Soluble Sugar
Free proline content in the phyllodes of salt-stressed plants was increased significantly, especially in plants under extreme stress. It increased by 7.1 (13.18 μmol g–1 FW) and 28.7 fold (53.33 μmol g–1 FW) in plants growing under 400 and 600 mM NaCl, over that in the control plants (1.86 μmol g–1 FW; Figure 4A). Similarly, the total soluble sugar were continuously accumulated in the phyllodes of salt-stressed plants and peaked to 42.1 mg g–1 DW (∼3.2 fold of control) in plants grown under 600 mM NaCl (Figure 4B). Sucrose, glucose, and fructose contents in salt-stressed plants were increased in relation to degree of salt stress. The sucrose, glucose, and fructose in plants grown under 600 mM NaCl were 2.2, 3.7, and 5.1 fold of control, respectively (Table 2). The accumulation of glucose and fructose was comparatively less in plants under 200 mM NaCl, where it increased by 2.3 and 3.6 fold, respectively, over that in the control plants.
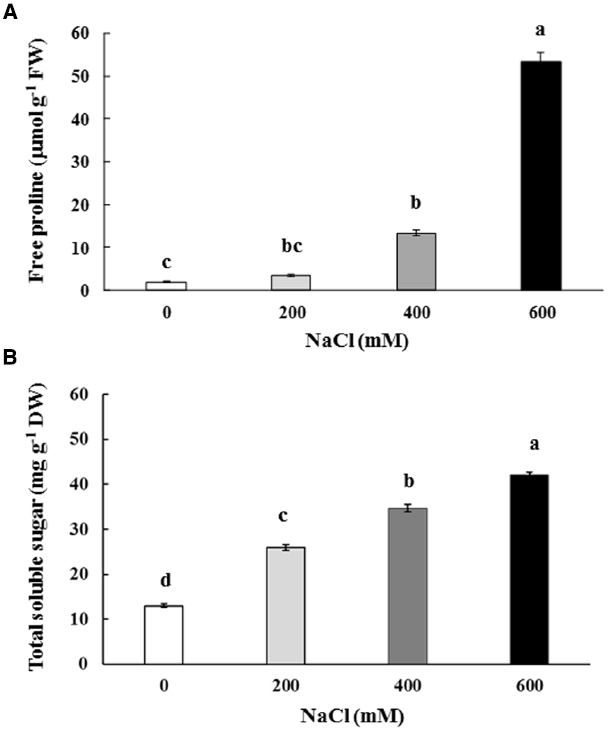
Figure 4. Free proline (A) and total soluble sugar (B) content of Acacia ampliceps seedlings grown under salt stress conditions for 9 days. Data presented as mean ± SE. Different letters represent significant difference at p ≤ 0.01 according to Tukey’s HSD test.

Table 2. Sucrose (Suc), glucose (Gluc), fructose (Fruc), stomatal conductance (gs), and transpiration rate (E) of Acacia ampliceps seedlings grown under salt stress conditions for 9 days.
Photosynthetic Abilities
Chla, Chlb, and TC in the phyllodes were enhanced in plants grown under mild salt stress (200 mM NaCl; Table 3). However, Chla, Chlb, and TC were degraded by 48.3, 29.9, and 39.8% of control, respectively, under extreme salt stress (600 mM NaCl; Table 3). In addition, Chla, a sensitive parameter, was significantly decreased when plants were exposed to 400 mM NaCl, whereas Chlb was maintained. The maximum quantum yield of PSII (Fv/Fm) and photon yield of PSII (ΦPSII) declined when plants were subjected to extreme salt stress (400–600 mM NaCl) for 9 days. Under extreme salt stress, Fv/Fm and ΦPSII were diminished by 17.0 and 13.5%, respectively, compared to that in mild salt-stressed plants (Table 3). Net photosynthetic rate (Pn) in mild salt-stressed plants was increased and then declined significantly at greater salt stress in a concentration-dependent manner (Figure 2D). Similarly, stomatal conductance (gs) and transpiration rate (E) in mild salt-stressed plants were peaked to 10.44 μmol H2O m–2 s–1 and 3.13 mmol H2O m–2 s–1, whereas these declined by 73.3 and 73.5%, respectively, when plants were exposed to 600 mM NaCl (Table 2).

Table 3. Chlorophyll a (Chla), chlorophyll b (Chlb), and total chlorophyll (TC) concentration, maximum quantum yield of PSII (Fv/Fm), and photon yield of PSII (ΦPSII) of Acacia ampliceps seedlings grown under salt stress conditions for 9 days.
Relationships Between Biochemical and Physiological Changes
Na+ enrichment in the phyllode tissues of salt-stressed plants was positively related to TC degradation (R2 = 0.72; Figure 5A). Consequently, Chla degradation was positively correlated with reduction in maximum quantum yield of PSII (Fv/Fm) (R2 = 0.59; Figure 5B). Likewise, the diminishing of PSII photon yield (ΦPSII) was strongly related to decreased net photosynthetic rate (Pn) (R2 = 0.81; Figure 5C), leading to declined plant DW (R2 = 0.91; Figure 5D).
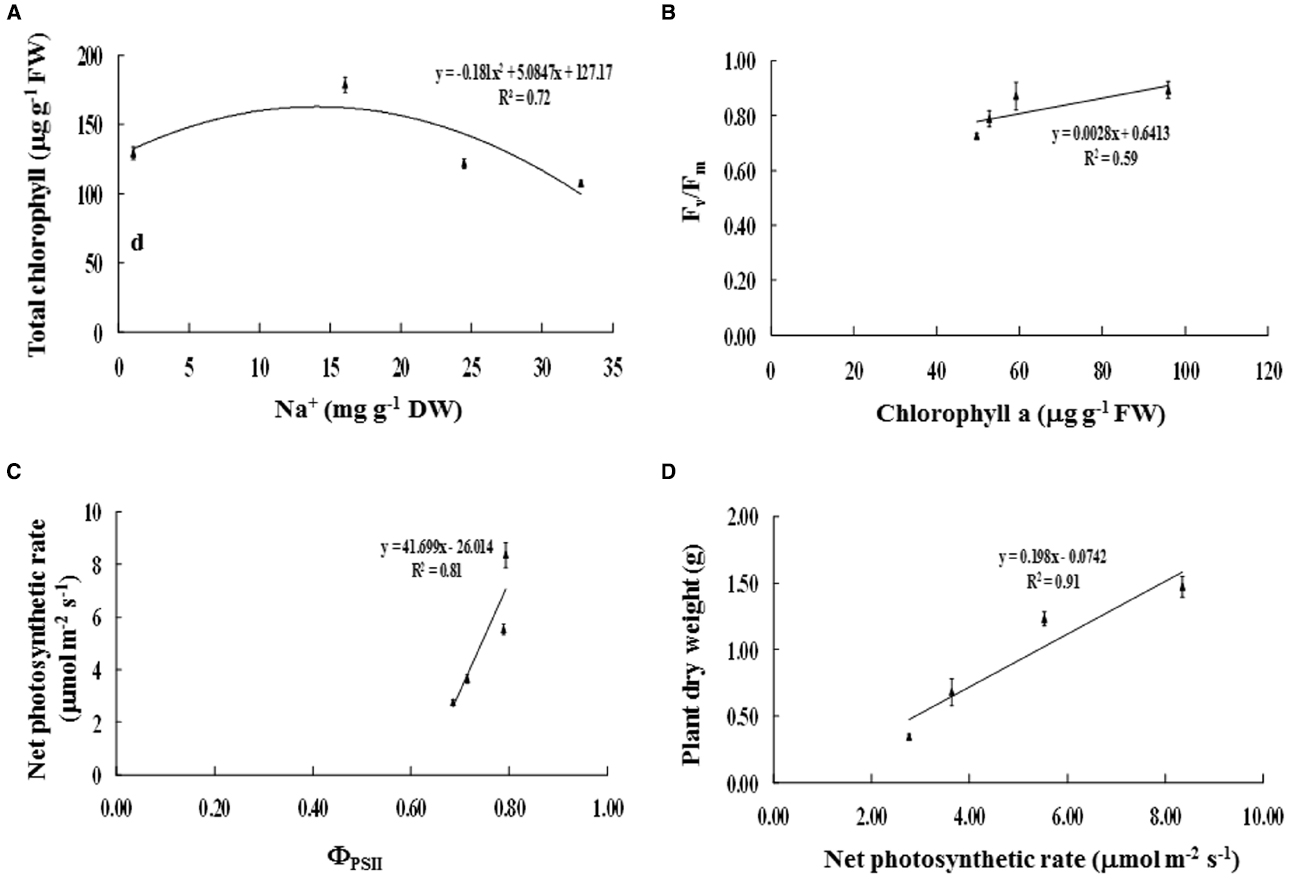
Figure 5. Relationships between (A) Na+ and total chlorophyll content, (B) chlorophyll a content and maximum quantum yield of PSII (Fv/Fm), (C) photon yield of PSII (ΦPSII) and net photosynthetic rate (Pn), and (D) net photosynthetic rate and plant dry weight of Acacia ampliceps seedlings grown under salt stress conditions for 9 days. Error bars represent ± SE.
Discussion
The growth performance of A. ampliceps was better exhibited in the low-salt concentration; however, it declined depending on the degree of salt contamination in the soil. Previously, the survival percentage of A. ampliceps was reported to be the maximum (98.1%) with 127.2 cm SH, when grown in the saline soil habitat for 9 months (Shirazi et al., 2006). The mortality of A. ampliceps grown under hydroponic culture was only 7% (retaining 38% DW of control) when plants were exposed to 1.5% NaCl (257 mM) and increased to 47% (with only 22% DW of control) under 2.0% NaCl (342 mM) (Yokota, 2003). Our findings are corroborated by an earlier study reporting improved growth characters like, shoot FW, shoot DW, root FW, and root DW- of A. ampliceps under mild salt stress (ECe = 10 dS m–1) in hydroponic culture and then declined under severe salt stress (ECe = 20–30 dS m–1), especially at pH 9.5 (Azhar et al., 2013). In contrast, SH, RL, shoot FW, shoot DW, root FW, and root DW of A. ampliceps declined in plants exposed to 100–300 mM NaCl for 2 days (Abbas et al., 2013). Also, Marcar and Crawford (2011) observed a decline in SH in several provenances of A. ampliceps under 150 mol m–3 salt treatment and saline waterlogging conditions. Parallel to our findings, SH and stem diameter of A. ampliceps were increased in Riyadh field trials, leading to an increase in the final biomass yield at 39.69 ton ha–1 (Aref et al., 2003). In the salt-affected fields (ECe = 13.9 dS m–1), SH and the number of branch per plants were enhanced, depending on the cultivation period (6–24 months; Abbas et al., 2015). The number of leaves is a good indicator to identify the mortality of plants grown under salt stress. Mahmood et al. (2009) suggested that the number of leaves of A. ampliceps were decreased by 10, 21, and 37% over the control, when subjected to ECe 10, 20, and 30 dS m–1, respectively, for 2 years.
In our study, Na+ and Ca2+ in phyllodes of A. ampliceps were continuously enriched depending on the salt concentration in the soil solution, whereas K+ was declined, thereby resulting in increased Na+/K+ ratio. In halophytic species, Na+ was enriched in vacuole organelles, relating to activities of Na+/H+ antiporter (NHXs) and vacuolar H+-ATPase (VH+-ATPase) localization on vacuolar membrane (Barkla et al., 2002; Wang et al., 2015). These are in conformity with previous findings of Marcar et al. (1991) and Abbas et al. (2013) who reported increased Na:K or reduce K:Na ratio, respectively. Na+ content in new phyllode (young) of A. ampliceps grown under salt stress (ECe = 40 dS m–1 in summer season) was peaked and greater than that in older phyllodes (Shirazi et al., 2001). A similar observation (Na+ accumulation and K+ reduction) has been demonstrated in other acacia species (A. auriculiformis, A. mangium, A. longifolia, and A. senegal) grown under salt stress (Marcar et al., 1991; Hardikar and Pandey, 2008; Morais et al., 2012). Sunarpi et al. (2005) suggested that high-salt concentrations induce uptake and transport of Na+ and decrease K+ content in shoots. In vacuoles, K+ play pivotal role in turgor generation; however during salinity there occurs an efflux of K+ and influx of Na+ (Pottosin and Maňiz, 2002; Adams and Shin, 2014). Notwithstanding, Na+ has been found to help in turgor maintenance (Kronzucker et al., 2013).
Earlier studies have demonstrated that halophytes have constitutive tonoplast antiporters that facilitate Na+ accumulation in vacuole via SOS regulation system (Blumwald, 2000). In molecular biological studies, NHXs, vacuolar Na+/H+ antiporters to drive the Na+ into vacuole of halophytic species, Aeluropus littoralis (Zhang et al., 2008) and Thellungiella halophila (Vera-Estrella et al., 2005) have been well established. NHXs derived from halophyte species have been characterized, cloned, and transformed into tobacco (Jha et al., 2011), Arabidopsis (Li et al., 2007) and rice crop (Verma et al., 2007). We found an increase in Ca2+ in phyllode of A. ampliceps grown under salt stress, and it was in sharp contrast to reduced Ca2+ in other Acacia species when plants were subjected to salt stress (Rehman et al., 2000; Hardikar and Pandey, 2008; Patel et al., 2010). Shirazi et al. (2001) reported that Ca2+ accumulation peaked at ECe = 40 dS m–1 in summer season, and it was 1.47 fold greater than that in plant under low ECe. Ca2+ alleviates salinity, protects plants and confers tolerance to salinity in glycophytes (Greenway and Munns, 1980; Hadi and Karimi, 2012). Exogenous Ca2+ has been reported to mitigate salinity in halophytes (Grigore et al., 2012). Ca2+ plays a key role in stabilization of cell wall structure, maintenance of structural and functional integrity of cell membrane, ion transport regulation, and ion-exchange behavior, besides acting as a messenger in stress signaling (Hasegawa et al., 2000; Hadi and Karimi, 2012). We observed Ca2+ and Na+ enrichment under extreme salinity condition. The increased Ca2+ accumulation despite Na+ enrichment was in sharp contrast that Na+ interferes with Ca2+ uptake under salt stress (Rehman et al., 2000). Ca2+ accumulation under extreme salt condition might protect A. ampliceps from the toxic effects of Na+ by activating SOS pathways, which protect against salinity-induced injury to cell membranes. But how it occurs in A. ampliceps is still unknown.
Free proline and total soluble sugar in phyllodes of A. ampliceps grown under salt stress was enriched proportional to salt concentration in the soil. It suggests a positive role of proline in osmotic adjustment under salt stress. These findings are corroborated by similar observation made earlier in leaf and root tissues of A. ampliceps, A. mangium, and A. auriculiformis grown under salt stress in a greenhouse, depending on the degree of NaCl or ECe strength (Diouf et al., 2005; Patel et al., 2010). Halophytes accumulate more proline than glycophytes, and it has been related to the suppression of proline catabolism by proline-oxidizing enzyme, PDH (proline dehydrogenase), and enhanced synthesis of proline via pyrroline-5-carboxylate synthetase (P5CS; Taji et al., 2004; Kant et al., 2006).
Previously, studies have demonstrated that total soluble sugar, i.e., sucrose, glucose, and fructose, content was enriched in Nipa palm exposed to 16.6 dS m–1 ECe as NaCl stress (Theerawitaya et al., 2014) and in the cotyledons of quinoa halophytes exposed to 200 mM NaCl for 4 days (Rosa et al., 2009a). Sucrose, the major carbon source in growing seedlings, along with glucose acts as osmoprotectant and helps in maintaining homoeostasis and biomembrane integrity during abiotic stresses (Rosa et al., 2009b). In our study, the sugar content positively correlated with Na+ levels in phyllodes, thereby suggesting a role in osmotic adjustment at the cellular level in salt defense mechanism as osmoprotectant (Ghosh et al., 2001). In plants, sugars do not only function as substrates for energy and carbon metabolism, but also act as osmolytes and messengers in signal transduction, and modulate growth, development, and gene expression (Rolland et al., 2002).
Na+ compartmentation in the vacuole organelles of mangrove species, Bruguiera sexangula has been proofed by ion accumulation, relating to increase in vacuole size and to regulate the activities of Na+/H+ exchanger and H+-ATPase (Kura-Hotta et al., 2001; Mimura et al., 2003). In the case of mild salt stress, Na+ compartmentation in A. ampliceps may play a key role as the salt defense mechanism to reduce ion toxic symptoms. Therefore, ion toxicity was found in the older phyllode, i.e., chlorosis of A. ampliceps when subjected to 600 mM NaCl for 9 days. In mangrove Bruguiera parviflora, chloroplast ultrastructure in plant grown under 400 mM NaCl was degraded as well as, pigment protein- and thylakoid protein-complexes in both content and activity were reduced, depending on the degree of salt treatment, (Parida et al., 2003) consequently to a decline in the net photosynthetic rate (Li et al., 2008). In the present study, chlorophyll pigment, chlorophyll fluorescence, and net photosynthetic rate of A. ampliceps were enhanced under the mild salt stress (200 mM NaCl) and then declined when subjected to severe salt stress (≥400 NaCl). Chla and Chlb pigments in salt-stressed A. auriculiformis were degraded in relation to the level of salinity (ranging from 1.2 to 10 dS m–1). Parallel to our findings, Fv/Fm, ΦPSII, Pn, gs, and E in Nipa palm (a halophytic species) were promoted by a low salt concentration (ECe = 8.9 dS m–1) and then significantly dropped, especially at the high concentration (ECe = 57.2 dS m–1) (Theerawitaya et al., 2014).
Conclusion
Na+ and Ca2+ in the phyllodes of A. ampliceps under extreme salt stress (600 mM NaCl) were enriched, while K+ declined, consequently resulting in increased Na:K ratio. Na+ enrichment in phyllode tissues of salt-stressed plants may get stored in vacuolar organelles as Na+ compartmentation. Alternatively, free proline and soluble sugar were enriched in cellular levels of leaf tissues to play a key role in osmoregulation of salt defense mechanism to protect A. ampliceps from salt-induced toxicity. Therefore, chlorophyll degradation, chlorophyll fluorescence diminishing, and net photosynthetic rate reduction in A. ampliceps under severe salt stress were evidently observed, thereby leading to overall growth inhibition.
Conflict of Interest Statement
The authors declare that the research was conducted in the absence of any commercial or financial relationships that could be construed as a potential conflict of interest.
Acknowledgments
This research was supported by the National Center for Genetic Engineering and Biotechnology, and partially funded by National Science and Technology Development Agency (NSTDA) for postdoctoral position to CT and TWAS Postdoctoral Fellowship Programme to HPS. We also thank Land Development Department, Ministry of Agriculture and Cooperatives, Thailand, for providing the seeds of Acacia ampliceps for the study.
References
Abbas, G., Saqib, M., Akhtar, J., and Basra, S. M. A. (2013). Salinity tolerance potential of two Acacia species at early seedling stage. Pak. J. Agric Sci. 50, 683–688.
Abbas, G., Saqib, M., Akhtar, J., Murtaza, G., Shahid, M., and Hussain, A. (2015). Relationship between rhizosphere acidification and phytoremediation in two Acacia species. J. Soil Sedim. doi: 10.1007/s11368-014-1051-9
Adams, E., and Shin, R. (2014). Transport, signaling, and homeostasis of potassium and sodium in plants. J. Integr. Plant Biol. 56, 231–249.
Ansari, R., Khan, M. A., and Gul, B. (2007). “Gainful utilization of salt affected lands: prospects and precautions,” in Crop and Forage Production Using Saline Waters, Vol. 10, eds M. Kafi and M. A. Khan (New Delhi: NAM S&T Centre), 103–108.
Aref, I. M., El-Juhany, L. I., and Hegazy, S. S. (2003). Comparison of the growth and biomass production of six Acacia species in Riyadh, Saudi Arabia after 4 years of irrigated cultivation. J. Arid. Environ. 54, 783–792. doi: 10.1006/jare.2002.1067
Ashraf, M. A., Awan, A. R., and Mahmood, K. (2012). Rehabilitation of saline ecosystems through cultivation of salt tolerant plants. Pak. J. Bot. 44, 69–75.
Azhar, M. F., Shah, A., H., Siddiui, M. A., and Anjum, K. (2013). Growth performance of Acacia ampliceps Maslin under combined effect of different salinity and pH levels. World Appli. Sci. J. 28, 562–567.
Barkla, B. J., Vera-Estrella, R., Camacho-Emiterio, J., and Pantoja, O. (2002). Na+/H+ exchange in the halophyte Mesembryanthemum crystallinum is associated with cellular sites of Na+ storage. Funct. Plant Biol. 29, 1017–1024. doi: 10.1071/FP02045
Bates, L. S., Waldren, R. P., and Teare, I. D. (1973). Rapid determination of free proline for water-stress studies. Plant Soil 39, 205–207. doi: 10.1007/BF00018060
Blumwald, E. (2000). Sodium transport and salt tolerance in plants. Curr. Opin. Cell Biol. 12, 431–434. doi: 10.1016/S0955-0674(00)00112-5
Cha-um, S., Supaibulwatana, K., and Kirdmanee, C. (2007). Glycinebetaine accumulation, physiological characterizations and growth efficiency in salt-tolerant and salt-sensitive lines of indica rice (Oryza sativa L. ssp. indica) in response to salt stress. J. Agron. Crop Sci. 193, 157–166. doi: 10.1111/j.1439-037X.2007.00251.x
Diouf, D., Dupionnois, R., Ba, A. T., Neyra, M., and Lesueur, D. (2005). Symbiosis of Acacia auriculiformis and Acacia mangium with mycorrhizal fungi and Bradyrhizobium spp. improves salt tolerance in greenhouse conditions. Funct. Plant Biol. 32, 1143–1152. doi: 10.1071/FP04069
Dynes, R. A., and Schlink, A. C. (2002). Livestock potential of Australia species of Acacia. Conserv. Sci. 4, 117–124.
Flowers, T. J., and Colmer, T. D. (2008). Salinity tolerance in halophytes. New Phytol. 179, 945–963. doi: 10.1111/j.1469-8137.2008.02531.x
Flowers, T. J., Galal, H. K., and Bromham L. (2010). Evolution of halophytes: multiple origins of salt tolerance in land plants. Funct. Plant Biol. 37, 604–612. doi: 10.1071/FP09269
Flowers, T. J., Hajibagheri, M. A., and Clipson, N. J. W. (1986). Halophytes. Q. Rev. Biol. 61, 313–337. doi: 10.1086/415032
Ghosh, S., Bagchi, S., and Majumder, A. L. (2001). Chloroplast fructose-1,6-biphosphatase from Oryza differ in salt tolerance property from the Porteresia enzyme and is protected by osmolytes. Plant Sci. 160, 1171–1181. doi: 10.1016/S0168-9452(01)00361-2
Giri, B., Kapoor, R., and Mukerji, K. G. (2003). Influence of arbuscular mycorrhizal fungi and salinity on growth, biomass, and mineral nutrition of Acacia auriculiformis. Biol. Fertil. Soil 38, 170–175. doi: 10.1007/s00374-003-0636-z
Greenway, M., and Munns, R. (1980). Mechanisms of salt tolerance in non-halophytes. Ann. Rev. Plant Physiol. 31, 149–190. doi: 10.1146/annurev.pp.31.060180.001053
Grigore, M. N., Boscaiu, M., Llinares, J., and Vicente, O. (2012). Mitigation of salt stress-induced inhibition of Plantago crassifolia reproductive development by supplemental calcium or magnesium. Not. Bot. Horti Agrobot. Cluj Nap. 40, 58–66.
Hadi, M. R., and Karimi, N. (2012). The role of calcium in plants’ salt tolerance. J. Plant Nutr. 35, 2037–2054. doi: 10.1080/01904167.2012.717158
Hardikar, S. A., and Pandey, A. N. (2008). Growth, water status and nutrient accumulation of seedlings of Acacia senegal (L.) Willd. in response to soil salinity. Anal. Biol. 30, 17–28.
Hasegawa, P. H., Bressan, R. A., Zhu, J. K., and Bohnert, H. J. (2000). Plant cellular and molecular responses to high salinity. Ann. Rev. Plant Physiol. Plant Mol. Biol. 51, 463–499. doi: 10.1146/annurev.arplant.51.1.463
Hossain, G. S., Waditee, R., Hibino, T., Tanaka, Y., and Takabe, T. (2006). Root specific expression of Na+/H+ antiporter gene from Synechocystis sp. PCC6803 confers salt tolerance of tobacco plant. Plant Biotechnol. 23, 275–281. doi: 10.5511/plantbiotechnology.23.275
Jha, A., Joshi, M., Yadav, N. S., Agarwal, P. K., and Jha, B. (2011). Cloning and characterization of the Salicornia brachiata Na+/H+ antiporter gene SbNHX1 and its expression by abiotic stress. Mol. Biol. Rep. 38, 1965–1973. doi: 10.1007/s11033-010-0318-5
Kant, S., Kant, P., Raveh, E., and Barak, S. (2006). Evidence that differential gene expression between the halophytes, Thellungiella halophila and Arabidopsis thaliana is responsible for higher levels of the compatible osmolyte proline and tight control of Na+ uptake in T. halophila. Plant Cell Environ. 29, 1220–1234. doi: 10.1111/j.1365-3040.2006.01502.x
Karkacier, M., Ebras, M., Uslu, M. K., and Aksu, M. (2003). Comparison of different extraction and detection methods for sugars using amino-bonded phase HPLC. J. Chromatogr. Sci. 41, 331–333. doi: 10.1093/chromsci/41.6.331
Kronzucker, H. J., Coskun, D., Schulze, L. M, Wong, J. R., and Britto, D. T. (2013). Sodium as a nutrient and toxicant. Plant Soil 369, 1–23. doi: 10.1007/s11104-013-1801-2
Kura-Hotta, M., Mimura, M., Tsujimura, T., Washitani-Nemoto, S., and Mimura, T. (2001). High salt-treatment-induced Na+ extrusion and low salt-treatment-induced Na+ accumulation in suspension-cultured cells of the mangrove plant, Bruguiera sexangula. Plant Cell Environ. 24, 1105–1112. doi: 10.1046/j.0016-8025.2001.00761.x
Li, J., Jiang, G., Huang, P., Ma, J., and Zhang, F. (2007). Overexpression of the Na+/H+ antiporter gene from Suaeda salsa confers cold and salt tolerance to transgenic Arabidopsis thaliana. Plant Cell Tiss. Org. Cult. 90, 41–48. doi: 10.1007/s11240-007-9246-z
Li, N., Chen, S., Zhou, X., Li, C., Shao, J., Wang, R., et al. (2008). Effect of NaCl on photosynthesis, salt accumulation, and compartmentation in two mangrove species, Kandelia candel and Bruguiera gymnorrhiza. Aqua. Bot. 88, 303–310. doi: 10.1016/j.aquabot.2007.12.003
Loggini, B., Scartazza, A., Brugnoli, E., and Navari-Izzo, F. (1999). Antioxidant defense system, pigment composition, and photosynthetic efficiency in two wheat cultivars subjected to drought. Plant Physiol. 119, 1091–1099. doi: 10.1104/pp.119.3.1091
Mahmood, K., Sarwar, G., Hussain, N., Schmeisky, H., and Muhammad, S. (2009). Effect of soil salinity and sodicity on growth parameters of Acacia ampliceps. Pak. J. Agric. Res. 22, 132–139.
Marcar, N. E., and Crawford, D. F. (2011). Intra-specific variation for response to salt and waterlogging in Acacia ampliceps Maslin seedlings. New For. 41, 207–219. doi: 10.1007/s11056-010-9221-0
Marcar, N. E., Dart, P., and Sweeney, C. (1991). Effect of root-zone salinity on growth and chemical composition of Acacia ampliceps B. R. Maslin, A. auriculiformis A. Cunn. Ex Benth. and A. mangium Willd. at two nitrogen levels. New Phytol. 119, 567–573. doi: 10.1111/j.1469-8137.1991.tb01049.x
Maxwell, K., and Johnson, G. N. (2000). Chlorophyll fluorescence-a practical guide. J. Exp. Bot. 51, 659–668. doi: 10.1093/jexbot/51.345.659
Mimura, T., Kura-Hotta, M., Tsujimura, T., Ohnishi, M., Miura, M., Okazaki, Y., et al. (2003). Rapid increase of vacuolar volume in response to salt stress. Planta 216, 397–402. doi: 10.1007/s00425-002-0878-2
Morais, M. C., Panuccio, M. R., Muscolo, A., and Freitas, H. (2012). Salt tolerance traits increase the invasive success of Acacia longifolia in Portuguese coastal dunes. Plant Physiol. Biochem. 55, 60–65. doi: 10.1016/j.plaphy.2012.03.013
Panta, S., Flowers, T., Lane, P., Doyle, R., Haros, G., and Shabala, S. (2014). Halophyte agriculture: success stories. Environ. Exp. Bot. 107, 71–83. doi: 10.1016/j.envexpbot.2014.05.006
Parida, A. K., Das, A. B., and Mittra, B. (2003). Effects of NaCl stress on the structure, pigment complex composition, and photosynthetic activity of mangrove Bruguiera parviflora. Photosynthetica 41, 191–200. doi: 10.1023/B:PHOT.0000011951.37231.69
Patel, A. D., Jadeja, H., and Pandey, A. N. (2010). Effects of salinization of soil on growth, water status and nutrient accumulation in seedlings of Acacia auriculiformis (Fabaceae). J. Plant Nutr. 33, 914–932. doi: 10.1080/01904161003669939
Pottosin, I., and Maňiz, J. (2002). Higher plant vacuolar ionic transport in the cellular context. Acta Bot. Maxicana. 60, 37–77.
Rehman, S., Harria, P. J. C., Bourne, W., and Wilkin, J. (2000). The relationship between ions, vigour and salinity tolerance of Acacia seeds. Plant Soil 230, 229–233. doi: 10.1023/A:1004701231183
Rengasamy, P. (2006). World salinization with emphasis on Australia. J. Exp. Bot. 57, 1017–1023. doi: 10.1093/jxb/erj108
Rolland, F., Moore, B., and Sheen, J. (2002). Sugar sensing and signaling in plants. Plant Cell 14, S185–S205. doi: 10.3389/fpls.2014.00113
Rosa, M., Hilal, M., González, J. A., and Prado, F. E. (2009b). Low-temperature effect on enzyme activities involved in sucrose-starch partitioning in salt-stressed and salt-acclimated cotyledons of quinoa (Chenopodium quinoa Willd.) seedlings. Plant Physiol. Biochem. 47, 300–330. doi: 10.1016/j.plaphy.2008.12.001
Rosa, M., Prado, C., Podazza, G., Interdonato, R., González, J. A., Hilal, M., et al. (2009a). Soluble sugars, metabolism, sensing and abiotic stress: a complex network in the life of plants. Plant Signal. Behav. 4, 388–393. doi: 10.4161/psb.4.5.8294
Shabala, S. (2013). Learning from halophytes: physiological basis and strategies to improve stress tolerance in crops. Ann. Bot. 112, 1209–1221. doi: 10.1093/aob/mct205
Shabala, S., Bose, J., and Hedrich, R. (2014). Salt bladders: do they matter? Trends Plant Sci. 19, 687–691. doi 10.1016/j.tplants.2014.09.001
Shabala, S. N., Shabala, S. I., Martynenko, A. I., Babourina, O., and Newman, I. A. (1998). Salinity effect on bioelectric activity, growth, Na+ accumulation and chlorophyll fluorescence of maize leaves: a comparative survey and prospects for screening. Aust. J. Plant Physiol. 25, 609–616. doi: 10.1071/PP97146
Shirazi, M. U., Khan, M. A., Ali, M., Mujtaba, S. M., Mumtaz, S., Ali, M., et al. (2006). Growth performance and nutrient contents of some salt tolerant multipurpose tree species growing under saline environment. Pak. J. Bot. 38, 1381–1388.
Shirazi, M. U., Khanzada, B., Alam, S. M., Ansari, R., Mujtaba, S. M., Ali, M., et al. (2001). Seasonal nutrient variation in two Acacia species growing under saline environment. Pak. J. Biol. Sci. 4, 514–517. doi: 10.3923/pjbs.2001.514.517
Sunarpi, Horie, T., Motoda, J., Kubo, M., Yang, H., Yoda, K., et al. (2005). Enhanced salt tolerance mediated by AtHKT1 transporter-induced Na+ unloading from xylem vessels to xylem parenchyma cells. Plant J. 44, 928–938. doi: 10.1111/j.1365-313X.2005.02595.x
Taji, T., Seki, M., Satou, M., Sakurai, T., Kobayashi, M., Ishiyama, K., et al. (2004). Comparative genomics in salt tolerance between Arabidopsis and Arabidopsis-related halophyte salt cress using Arabidopsis microarray. Plant Physiol. 135, 1697–1709. doi: 10.1104/pp.104.039909
Takemura, T., Hanagata, N., Suugihara, K., Baba, S., Karube, I., and Dubinsky, Z. (2000). Physiological and biochemical responses to salt stress in the mangrove, Bruguiera gymnorrhiza. Aqua. Bot. 68, 15–28. doi: 10.1016/S0304-3770(00)00106-6
Tanaka, K., Ohta, K., Haddad, P. R., Fritz, J. S., Lee, K. P., Hasebe, K., et al. (1999). Acid-rain monitoring in East Asia with a portable-type ion-exclusion-cation-exchange chromatographic analyzer. J. Chromatog. 850, 311–317. doi: 10.1016/S0021-9673(99)00286-1
Theerawitaya, C., Samphumphuang, T., Cha-um, S., Yamada, N., and Takabe, T. (2014). Responses of Nipa palm (Nypa fruticans) seedlings, a mangrove species, to salt stress in pot culture. Flora 209, 597–603. doi: 10.1016/j.flora.2014.08.004
Vera-Estrella, R., Barkla, B. J., García-Ramírez, L., and Pantoja, O. (2005). Salt stress in Thellungiella halophila activates Na+ transport mechanisms required for salinity tolerance. Plant Physiol. 139, 1507–1517. doi: 10.1104/pp.105.067850
Verma, D., Singla-Pareek, S. L., Rajagopal, D., Reddy, M. K., and Sopory, S. K. (2007). Functional validation of a novel isoform of Na+/H+ antiporter from Pennisetum glaucum for enhancing salinity tolerance in rice. J. Biosci. 32, 621–628. doi: 10.1007/s12038-007-0061-9
Wang, J., Li, S., Meng, Y., Ma, X., Lai, Y., Yang, K., et al. (2015). Transcriptomic profiling of the salt-stress response in the halophyte Halogeton glomeratus. BMC Genome. 16:169. doi: 10.1186/s12864-015-1373-z
Wongpokkhom, N., Kheoruenromne, I., Suddhiprakarn, A., and Gilkes, R. J. (2008). Micromorphological properties of salt affected soils in Northeast Thailand. Geoderma 144, 158–170. doi: 10.1016/j.geoderma.2007.10.026
Yokota, S. (2003). Relationship between salt tolerance and proline accumulation in Australian Acacia species. J. For. Res. 8, 89–93. doi: 10.1007/s103100300010
Keywords: halophytic species, net photosynthetic rate, osmotic adjustment, Na+/K+ ratio, free proline, soluble sugar
Citation: Theerawitaya C, Tisarum R, Samphumphuang T, Singh HP, Cha-Um S, Kirdmanee C and Takabe T (2015) Physio-biochemical and morphological characters of halophyte legume shrub, Acacia ampliceps seedlings in response to salt stress under greenhouse. Front. Plant Sci. 6:630. doi: 10.3389/fpls.2015.00630
Received: 28 March 2015; Accepted: 30 July 2015;
Published: 31 August 2015.
Edited by:
Tetsuo Takano, The University of Tokyo, JapanReviewed by:
Nabil I. Elsheery, Tanta University, EgyptGiridara K. Surabhi, Regional Plant Resource Centre, India
Copyright © 2015 Theerawitaya, Tisarum, Samphumphuang, Singh, Cha-Um, Kirdmanee and Takabe. This is an open-access article distributed under the terms of the Creative Commons Attribution License (CC BY). The use, distribution or reproduction in other forums is permitted, provided the original author(s) or licensor are credited and that the original publication in this journal is cited, in accordance with accepted academic practice. No use, distribution or reproduction is permitted which does not comply with these terms.
*Correspondence: Suriyan Cha-Um, National Center for Genetic Engineering and Biotechnology, National Science and Technology Development Agency, 113 Thailand Science Park, Pahonyothin Road, Khlong Nuang, Khlong Luang, Pathum Thani 12120, Thailand, suriyanc@biotec.or.th