- 1School of Bioscience and Biotechnology, Tokyo University of Technology, Hachioji, Japan
- 2Department of Applied Biological Science, Tokyo University of Science, Noda, Japan
- 3Research Institute for Science and Technology, Tokyo University of Science, Noda, Japan
Salinity stress, which induces both ionic and osmotic damage, impairs plant growth and causes severe reductions in crop yield. Plants are equipped with defense responses against salinity stress such as regulation of ion transport including Na+ and K+, accumulation of compatible solutes and stress-related gene expression. The initial Ca2+ influx mediated by plasma membrane ion channels has been suggested to be crucial for the adaptive signaling. NADPH oxidase (Nox)-mediated production of reactive oxygen species (ROS) has also been suggested to play crucial roles in regulating adaptation to salinity stress in several plant species including halophytes. Respiratory burst oxidase homolog (Rboh) proteins show the ROS-producing Nox activity, which are synergistically activated by the binding of Ca2+ to EF-hand motifs as well as Ca2+-dependent phosphorylation. We herein review molecular identity, structural features and roles of the Ca2+-permeable channels involved in early salinity and osmotic signaling, and comparatively discuss the interrelationships among spatiotemporal dynamic changes in cytosolic concentrations of free Ca2+, Rboh-mediated ROS production, and downstream signaling events during salinity adaptation in planta.
Introduction
Soil salinity impairs plant growth and development and is an important factor limiting crop productivity and yield worldwide (Rengasamy, 2006). Salinity stress reduces water potential, thereby preventing water uptake by roots and provoking a set of responses similar to those of a water deficit. Thus, by causing osmotic stress, salinity provokes the stomatal limitation of photosynthesis, loss of turgor, enhanced photorespiration, and excess production of ROS (Chaves et al., 2009). The ionic component of salinity stress is attributed to the direct toxic effects of Na+ and imbalances in the homeostasis of other ions such as K+ and Ca2+ (Munns and Tester, 2008).
To mount an effective response to cope with salinity stress, land plants have developed the ability to sense both osmotic stress and Na+ (Deinlein et al., 2014). Plants exhibit a rapid increase in the cytosolic Ca2+ concentration within seconds of being exposed to NaCl or mannitol (Knight et al., 1997). The Ca2+ rise originated within the roots (Tracy et al., 2008) is propagated systemically (Choi et al., 2014), and occurs in several cell types (Kiegle et al., 2000).
Reactive oxygen species such as (superoxide anion radical), H2O2 (hydrogen peroxide), and OH⋅ (hydroxyl radical) are highly toxic substances produced through aerobic respiration and photosynthesis that oxidize various biomolecules such as DNA, proteins and lipids, and disrupt the cell redox state. Therefore, plants have developed various systems to scavenge ROS (Choudhury et al., 2013). Direct exposure of plant tissues to H2O2 activates antioxidant enzymes as well as the expression of the corresponding genes (Mylona et al., 2007). ROS are also enzymatically produced and act as signaling molecules in plant responses to abiotic and/or biotic stresses (Kärkönen and Kuchitsu, 2015).
Respiratory burst oxidase homologs has been identified as ROS-producing Noxs that generate by oxidizing NADPH and transferring an electron to oxygen (Sumimoto, 2008). Noxs are rapidly activated in response to various stimuli to induce ROS production and are involved in the regulation of a wide range of physiological functions in plants (Marino et al., 2012). Rbohs play central roles in the Ca2+-ROS signaling network triggered by their phosphorylation during stress adaptation (Takeda et al., 2008; Kimura et al., 2012; Gilroy et al., 2014).
We here review molecular mechanisms and roles of PM Ca2+-permeable channels as putative osmosensors and enzymatic ROS production involved in early salinity and osmotic responses, and discuss the interrelationships among dynamic changes in [Ca2+]cyt, ROS production, downstream signaling events during salinity sensing and adaptation in plants including halophytes.
Candidates for Plant Ca2+-Permeable MS Channels as Osmosensors
Mechanical stimuli, such as touch, bending, and barriers for growth as well as osmotic potential changes, all elicit rapid and transient increases in [Ca2+]cyt. Expression of cellular Ca2+-binding regulatory proteins including CaM and CML are induced by mechanical stimuli (Braam and Davis, 1990). Early responses to salinity stress reflect turgor changes and involve Ca2+-mediated signaling (Donaldson et al., 2004). PM Ca2+-permeable MS channels are suggested to sense salinity stress-triggered osmotic potential change. The following protein families are suggested as putative plant PM Ca2+-permeable MS channels as osmosensors.
OSCA1
OSCA1 was recently identified as a novel putative PM hyper-osmolality-gated Ca2+-permeable channel in guard cells and roots of Arabidopsis (Yuan et al., 2014). OSCA1 comprises nine transmembrane α-helices, but shows no significant similarity to known ion channels. Electrophysiological analyses of OSCA1 expressed in HEK293 cells showed its hyper-osmolality-gated Ca2+-permeable channel activity (Yuan et al., 2014). In osca1, early osmotic signaling events in guard cells were impaired and downstream adaptation responses against osmotic stress were attenuated (Yuan et al., 2014).
MCA Family
The yeast Mid1 protein shows MS channel activity when expressed in mammalian cells (Kanzaki et al., 1999). MCAs were identified in Arabidopsis based on their ability to complement the yeast mutant mid1 (Nakagawa et al., 2007). MCAs comprise at least one transmembrane domain and contain an EF hand, but show no significant similarity to known ion channels. All MCAs are localized to the PM and mediate Ca2+ uptake, with the N-terminal and EF hand regions being necessary structures for transport. MCAs are present in all land plants, including ferns and mosses, but not in algae, animals, protists, or fungi, suggesting that the function of MCAs is fundamental to land plants (Kurusu et al., 2013).
Ca2+ current induced by trinitrophenol, a potent compound to generate membrane distortion to activate MS channels, is enhanced in the MCA1-overexpressing cells (Nakagawa et al., 2007), and MCA1 and MCA2 expressed in Xenopus oocytes showed MS cation channel activity (Furuichi et al., 2012). Touch response in the primary root is impaired in mca1, while mca2 showed lower root Ca2+ uptake (Nakagawa et al., 2007; Yamanaka et al., 2010), suggesting that MCA1 and MCA2 play different physiological roles. Hypo-osmotic shock-induced PM Ca2+ influx and ROS production are partially impaired in OsMCA1-suppressed rice cultured cells (Kurusu et al., 2012a). Tobacco MCA homologs are localized at the Hechtian strand that connects PM and cell wall (Kurusu et al., 2012b). These findings suggest that MCAs are involved in hypo-osmotic stress-induced PM Ca2+ influx (Kurusu et al., 2013). MCA1 and MCA2 form a tetramer (Nakano et al., 2011; Shigematsu et al., 2014), suggesting that MCA proteins may function as a tetramer to form a Ca2+-permeable pore.
MSL Family and Others
Genome-wide screening to search for plant MS channels homologous to eukaryotic and prokaryotic MS channels identified the MSL protein family (Haswell et al., 2011). MSLs are present in land plants, and electrophysiological studies suggest that MSL9 and MSL10 permeate Cl- rather than Ca2+ (Haswell et al., 2008). It remains unknown whether any of MSLs are involved in the mechanical stimuli-induced Ca2+ influx as Ca2+-permeable channels.
Piezo proteins in mouse and Drosophila are also pore-forming subunits of MS channels and respond to mechanical stimuli. Many eukaryotic species including plants but not yeast and bacteria have a single Piezo protein (Coste et al., 2010). Functional roles of plant Piezo protein have not yet been reported.
An Arabidopsis histidine kinase HK1 may share a similar function with yeast SLN1 as an osmosensor (Urao et al., 1999; Wohlbach et al., 2008). It is of interest to determine whether any of these putative MS channels or osmosensors interacts with each other physically or functionally to monitor mechanical stimuli under salinity stress.
Plant Signaling Networks Involving Ca2+ and ROS under Salinity Stress
Physiological Roles of Rboh/Nox in Early Salinity Responses in Arabidopsis
Reactive oxygen species are highly toxic substances that oxidize various biomolecules. Generation of OH⋅ has significant implications to cell metabolism and causes lipid peroxidation (Apel and Hirt, 2004). K+ deficiency causes an increase in production by Rboh/Nox in root cells, and enhanced activation of antioxidant enzymes under salinity stress involves cytosolic K+ retention, suggesting that optimizing the K+ nutritional status may reduce the toxic effect of ROS by regulating Nox activity, and is essential for plant performance against salinity (Shabala and Pottosin, 2014).
Exposure to salinity activates the SOS pathway, leading to Ca2+-dependent activation of SOS1, a PM Na+/H+ exchanger that enables adaptation through Na+ efflux (Shi et al., 2000; Chung et al., 2008). Expression of SOS1 is induced under salinity stress, which requires activation of the Ca2+ sensor SOS3/CBL4 (calcineurin B-like 4) (Shi et al., 2000). Nox-mediated ROS production stabilizes SOS1 transcripts (Chung et al., 2008). Growth of better-adapted secondary roots is impaired in sos1 (Huh et al., 2002) and involves production, possibly by Rboh/Nox (Roach and Kranner, 2011).
Arabidopsis contains 10 Nox genes, RbohA-J, which exhibit different patterns of expression and in response to environmental factors (Marino et al., 2012). Expression of RbohD and RbohF are highly induced under salinity stress (Ma et al., 2012). An RbohF mutant showed strong Na+ hypersensitivity in its shoots and lacked ROS accumulation in its vasculature (Jiang et al., 2012), suggesting that RbohF plays a role in the regulation of xylem loading of Na+ to protect leaves from salinity stress in Arabidopsis. ROS production by Rboh/Nox is also suggested to be coordinated by signaling pathways involving phospholipid and actin in salinity stress responses, and is required for heme oxygenase-mediated salt acclimation signaling (Xie et al., 2011).
The Interrelationships between Ca2+ Influx and Rboh/Nox-Mediated ROS Production in Early Salinity Responses
Plant Rbohs have two N-terminal EF-hand motifs. A heterologous expression system based on HEK293T cells is effective to quantitatively evaluate (Ogasawara et al., 2008) and reconstitute the ROS-producing activity of Rbohs (Drerup et al., 2013; Kimura et al., 2013). Rbohs are basically synergistically activated by the Ca2+-binding to the EF-hand motifs and phosphorylation (Ogasawara et al., 2008). Protein phosphorylation is a prerequisite for the Ca2+-dependent activation of Rbohs (Kimura et al., 2012). Arabidopsis CIPK26, binds to RbohF in planta (Kimura et al., 2013) and in the presence of PM-localized Ca2+ sensor proteins CBL1/CBL9, CIPK26 enhances the activity of RbohF (Drerup et al., 2013). Rbohs are phosphorylated and activated by two families of Ca2+-dependent protein kinases (CBL-CIPK complexes and CDPKs; Kobayashi et al., 2007; Drerup et al., 2013; Dubiella et al., 2013), and two families of Ca2+-independent protein kinases (RLCKs and SnRK2s; Sirichandra et al., 2009; Kadota et al., 2014).
Reactive oxygen species-activated Ca2+-permeable channels and Rbohs have been suggested to constitute a positive feedback mechanism that enhances Ca2+ and ROS signals in root cells (Takeda et al., 2008). RbohC/RHD2 affects mechanical stress-induced ROS generation, and regulates Arabidopsis root hair elongation in a Ca2+-dependent manner (Takeda et al., 2008; Monshausen et al., 2009). Hyperpolarization- and depolarization-activated PM Ca2+-permeable channels may play roles in salinity-induced [Ca2+]cyt increase (Tracy et al., 2008).
Salinity-induced elevation of [Ca2+]cyt corresponds to PM Ca2+ influx as well as Ca2+ release from intracellular Ca2+ stores, and plays important roles in ROS signaling and salt tolerance (Shabala and Newman, 2000; Kader and Lindberg, 2010). Salinity-induced Ca2+ influx currents were markedly suppressed in the rbohD/F double mutant in Arabidopsis, implicating the roles of RbohD and RbohF in salt-stimulated [Ca2+]cyt rise (Ma et al., 2012).
Plant annexins have been reported to form Ca2+-permeable channels in planar lipid bilayers (Laohavisit and Davies, 2011). Arabidopsis AtANN1 is localized at the PM and responsible for root epidermal PM Ca2+-permeable conductance, which is activated by extracellular OH⋅ (Lee et al., 2004; Laohavisit et al., 2012). High concentration of NaCl promotes extracellular formation of OH⋅ (Demidchik et al., 2010) and accumulation of AtANN1 in membranes (Lee et al., 2004), and promotes secondary root formation (Huh et al., 2002). AtANN1-mediated Ca2+ influx through the PM depends on extracellular Ca2+ and ROS, and the atann1 mutant shows reduced secondary root formation and reduced activation of NaCl-induced transcription under salinity stress conditions (Laohavisit et al., 2013), suggesting a role of AtANN1 in root cell adaptation to salinity in Arabidopsis.
Salinity-induced Na+ accumulation in the cytosol triggers [Ca2+]cyt elevation, leading to activation of Rboh/Nox (Lecourieux et al., 2006) and apoplastic H2O2 accumulation. By interacting with transition metals such as Fe in the cell wall, H2O2 forms OH⋅ (Kuchitsu et al., 1995; Rodrigo-Moreno et al., 2013). OH⋅ directly activates both outward-rectifying depolarization-activating K+ channels (GORKs) and K+-permeable NSCCs, resulting in K+ leakage from roots (Shabala and Pottosin, 2014). The absolute concentration of K+ is essential to confer salinity stress tolerance, and the loss of K+ is suggested to play a primary role in the activation of caspase-like proteases and PCD (Shabala, 2009).
Salinity also induces accumulation of polyamines. Polyamines could generate ROS as a substrate of amine oxidases in the apoplast (Kärkönen and Kuchitsu, 2015). Both OH⋅ and polyamines may provoke a substantial remodeling of PM conductance of cations and anions and affect Ca2+ signaling in plants including halophytes (Pottosin et al., 2014). Crosstalk between ROS and polyamines in the regulation of PM ion transport may reveal a novel function of ROS production as signaling molecules during salinity adaptation.
A Ca2+-ROS Signaling Network in Osmotic Responses in Roots under Salinity Stress
Osmotic-shock induces Ca2+ influx followed by ROS production in many cell types in plants. The Ca2+ influx and Nox-mediated ROS production triggered by osmotic shock both require extracellular Ca2+, suggesting that PM Ca2+ influx is a prerequisite for ROS production (Beffagna et al., 2005).
mid1-complementing activities (MCAs), putative PM Ca2+-permeable MS channel components are suggested to play a role in the regulation of mechanical responses via signal transduction pathways dependent on Ca2+ and ROS (Kurusu et al., 2013). The overexpression of OsMCA1 enhances Rboh/Nox-mediated ROS production in rice cultured cells (Kurusu et al., 2012a). MCA1 and ROS produced by RbohD and/or RbohF may play roles in the modulation of osmosensitive metabolic changes (Wormit et al., 2012). Overexpression of MCAs enhances the expression of touch-inducible gene TCH3/CML12 encoding a CML in Arabidopsis (Nakagawa et al., 2007).
Apoplastic ROS production plays a key role in regulating cell wall metabolism, e.g., cross-linking of polysaccharides and glycoproteins to control cell wall rigidity (O’Brien et al., 2012; Kärkönen and Kuchitsu, 2015). H2O2 is also transported to the cytosol by PM aquaporins (Dynowski et al., 2008) to modify the cysteine residues of target proteins, thereby promoting redox signaling (Spoel and Loake, 2011).
These findings suggest the following initial PM signaling mechanism in response to osmo-stimulation under salinity stress (Figure 1): Salinity stress causes osmotic shock to trigger the activation of PM MS Ca2+-permeable channels and Ca2+ influx. Elevated cytosolic Ca2+ leads to the activation of various Ca2+ sensor proteins including CaMs, CMLs, CBLs, and CDPKs. Rbohs are synergistically activated by Ca2+-binding to the EF-hand motifs and phosphorylation to produce ROS. Elevated cytosolic Ca2+ and apoplastic ROS may also activate not only ROS-activated PM Ca2+-permeable channels including annexins but also as-yet-unidentified Ca2+-permeable channels/transporters localized at the endomembranes to sustain further Ca2+ mobilization. The signaling network dependent on Ca2+ and ROS may play a crucial role in regulating downstream events such as the Ca2+-dependent activation of Na+/H+ exchangers, SOS1 and NHX1 (Reguera et al., 2014), Na+ efflux from the cytosol, regulation of xylem loading of Na+ (de Boer and Wegner, 1997), Na+ exclusion from leaves (Sunarpi et al., 2005), induction of osmolytes and osmo-protective proteins, and the retention of cytosolic ion balance. Once this signaling is over, elevated [Ca2+]cyt would be reverted by the orchestrated action of Ca2+ exchangers and Ca2+-ATPases at the PM and tonoplast (reviewed in Bose et al., 2011).
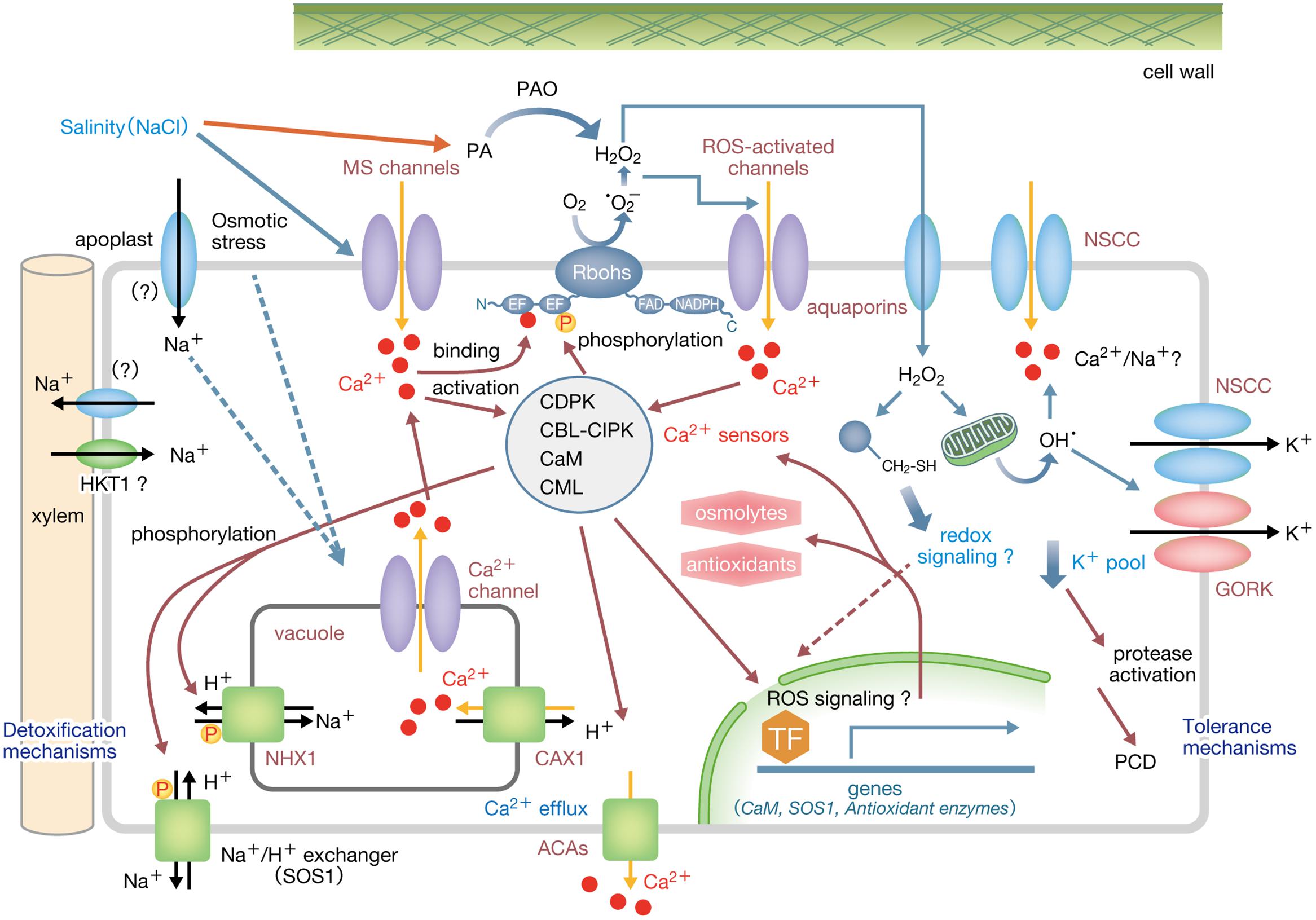
FIGURE 1. A possible Ca2+-ROS signaling network involved in osmotic responses under salinity stress in root cells. Salinity stress causes osmotic shock to trigger the activation of PM MS Ca2+-permeable channels and Ca2+ influx. Elevated [Ca2+]cyt leads to activation of various Ca2+ sensor proteins including CaMs, CMLs, CBLs, CDPKs as well as the ROS-producing enzyme, Noxs/Rbohs that are synergistically activated by Ca2+ binding and phosphorylation by Ca2+-dependent protein kinases such as CDPKs and CBL–CIPK complexes. Elevated apoplastic ROS activates ROS-activated PM Ca2+-permeable channels to trigger further Ca2+ influx, while Ca2+-permeable endomembrane channels/transporters may also been activated to sustain further Ca2+ mobilization. Elevated [Ca2+]cyt may trigger downstream events such as activation of SOS1 and NHX1, Na+/H+ exchangers that transport cytosolic Na+, regulation of xylem loading of Na+, induction of osmolytes and osmo-protective proteins, and the retention of cytosolic ion balance. Ca2+-dependent protein kinases activated by elevated [Ca2+]cyt mediate downstream events including expression of genes encoding proteins such as SOS1, antioxidant enzymes, osmolytes-biosynthetic enzymes as well as CaMs as signal amplifiers. Elevated [Ca2+]cyt is reverted by the orchestrated action of active transporters such as Ca2+ exchangers and Ca2+-ATPases at the PM and tonoplast. H2O2 is transported to the cytosol by PM aquaporins to modify the cysteine residues of target proteins, thereby promoting redox signaling to regulate gene expression. Broken arrows indicate hypothetical links. ACA, autoinhibited Ca2+-ATPase; PA, polyamine; PAO, polyamine oxidase.
Calcium-dependent protein kinases (Boudsocq and Sheen, 2013) and CBL-CIPK complexes (Weinl and Kudla, 2009) are suggested to transduce the osmotic signal to regulate downstream posttranslational modification and gene transcription. Furthermore, transcription factors such as calmodulin-binding transcription activators (CAMTAs; Pandey et al., 2013) are suggested to be directly activated by Ca2+/CaM. Interestingly, a recent comparative expression study in glycophytes and halophytes revealed that the induction of Ca2+/CaM-like proteins such as CDPKs are clearly enhanced in halophytes against salinity stress (Xu et al., 2013). Such Ca2+-binding proteins may play roles as important ‘amplifier’ of initial PM Ca2+ influx and Rboh-mediated ROS production in salinity stress signaling.
Ca2+ signaling also plays a critical role in salinity stress-triggered systemic signaling. A cation channel TPC1 is reported to be involved in the propagation of salinity stress-triggered systemic Ca2+ wave in roots, and may also be contributing to whole-plant resistance to salinity stress in Arabidopsis (Choi et al., 2014). Ca2+-ROS waves involving TPC1 may elicit systemic molecular responses in target organs and contribute to whole-plant stress tolerance (Choi et al., 2014; Gilroy et al., 2014).
Hydrogen peroxide transported from the apoplast to the cytosol by PM aquaporins may also interact with generated in the mitochondria under salinity stress to generate OH⋅ in the cytosol by Fenton reaction, which could activate NSCCs from the cytosolic site (Rodrigo-Moreno et al., 2013). As NSCCs are permeable to Na+, Ca2+, and K+, this activation may enhance Na+ uptake and K+ loss, further causing the cytosolic K+/Na+ imbalance. The resultant K+ loss from the cell may induce activation of caspase-like proteases and leading to PCD. Moreover, OH⋅-induced activation of NSCCs may further boost Ca2+ uptake, providing an additional positive feedback loop.
Possible Function of Initial ROS Production and Downstream Signaling Events during Stress Adaptation in Halophytes
The salt tolerance of halophytes is typically examined after long-term exposure to different NaCl concentrations (Tada et al., 2014). However, plant responses to salinity may be determined by rapid perception of salt shock that occurs within hours (Ellouzi et al., 2011; Wang et al., 2012).
Recent comparative studies of three Brassicaceae species, namely two halophytes, Cakile maritime and Thellungiella salsuginea, and a glycophyte, Arabidopsis thaliana, demonstrated that both osmotic potential changes and enhanced transient ROS production occurred in the halophyte roots within hours of exposure to NaCl, and subsequently enhanced the activation of antioxidant defenses as well as expression of transcription factors in the leaves and roots under long-term salinity stress. In contrast, the ROS accumulation continued in the glycophyte, Arabidopsis, during the entire observation period (Ellouzi et al., 2014), indicating that osmotic shock triggered by salinity stress may activate the initial production of ROS, and this phase is the basis of all functional changes leading to a second phase to activate defense mechanisms (Choudhury et al., 2013). The early production of H2O2 by RbohD and RbohF is suggested to be required for salinity-induced antioxidant defense responses in Arabidopsis under short-term stress treatments (Ben Rejeb et al., 2015). Interestingly, the Nox activity decreased under salinity stress in a glycophyte (B. juncea), while it was unaffected in a halophyte (S. portulacastrum) (Srivastava et al., 2015). These findings suggest that early production of low levels of H2O2 acts as an acclimation signal to trigger a preconditioning response by inducing antioxidant enzyme activities in order to efficiently cope with the subsequent production of ROS and lipid peroxidation, thereby preventing or minimizing salinity stress-derived injuries in several halophytes. Regulatory mechanisms of Nox-mediated ROS production in the salinity stress response have not yet been examined genetically in halophytes. Future studies on Rbohs/Noxs in various plant species including halophytes may reveal a novel function of ROS production as signaling molecules during salinity adaptation.
Halophytes do not always need to possess high antioxidant activity because of their higher capacity to prevent ROS generation (Bose et al., 2014). Salt-tolerant species equipped with efficient mechanisms for Na+ exclusion from the cytosol may not require a high level of antioxidant activity. Such exclusion is most probably achieved by the orchestrated action of several complementary mechanisms including SOS1-mediated Na+ exclusion from the cell (Shi et al., 2000), vacuolar Na+ sequestration by tonoplast NHXs (Blumwald, 2000) and efficient control of tonoplast fast (FV) and slow (SV) channels to prevent Na+ back-leak into the cytosol (Bonales-Alatorre et al., 2013a,b).
Although a rapid [Ca2+]cyt increase mediated by PM Ca2+-permeable MS channels and their interacting molecules is a hallmark response to osmotic stress (Monshausen and Gilroy, 2009; Kurusu et al., 2013), Ca2+-independent osmotic sensory mechanisms may also play a role. Effectors of ROS and signaling cascades downstream of Rbohs need to be elucidated to better understand their roles. The crosstalk of Rboh/Nox-mediated Ca2+-dependent ROS production with other signaling pathways involving ABA, MAPK, and NO as well as jasmonic acid may be important in transcriptional regulation.
In addition, mild salinity stress in the seedling stage induces osmotic priming in Arabidopsis (Sani et al., 2013). Since salinity stress has been shown to affect DNA methylation status of many promoters in soybean (Song et al., 2012), the promoter regions of Rboh in halophytes may have characteristic consensus sequences involved in chromatin modification, and may, thus, enhance plant salinity tolerance.
Conflict of Interest Statement
The authors declare that the research was conducted in the absence of any commercial or financial relationships that could be construed as a potential conflict of interest.
Acknowledgments
We would like to thank Dr. Hidetoshi Iida (Tokyo Gakugei University, Tokyo, Japan) and Dr. Kenji Hashimoto (Tokyo University of Science, Chiba, Japan) for their critical comments of the manuscript. This work was supported in part by Grants-in-Aid from the Ministry of Education, Culture, Sports, Science and Technology for Young Scientists (B) (15K21450) to TK and on Innovative Areas (25114515) to KK.
Abbreviations
CaM, calmodulin; CBL, calcineurin B-like protein; CDPK, calcium-dependent protein kinase; CIPK, CBL-interacting protein kinase; CML, calmodulin-like protein; [Ca2+]cyt, cytosolic concentration of free Ca2+; HEK, human embryonic kidney; MCA, mid1-complementing activity; MS, mechanosensitive; MSL, MscS-like protein; Nox, NADPH oxidase; NSCC, non-selective cation channel; OSCA1, reduced hyperosmolarity-induced [Ca2+]i increase 1; PCD, programmed cell death; PM, plasma membrane; Rboh, respiratory burst oxidase homolog; RLCK, receptor-like cytoplasmic kinase; ROS, reactive oxygen species; SOS, salt overly sensitive; TPC1, two-pore channel 1.
References
Apel, K., and Hirt, H. (2004). Reactive oxygen species: metabolism, oxidative stress, and signal transduction. Annu. Rev. Plant Biol. 55, 373–399. doi: 10.1146/annurev.arplant.55.031903.141701
Beffagna, N., Buffoli, B., and Busi, C. (2005). Modulation of reactive oxygen species production during osmotic stress in Arabidopsis thaliana cultured cells: involvement of the plasma membrane Ca2+-ATPase and H+-ATPase. Plant Cell Physiol. 46, 1326–1339. doi: 10.1093/pcp/pci142
Ben Rejeb, K., Benzarti, M., Debez, A., Bailly, C., Savouré, A., and Abdelly, C. (2015). NADPH oxidase-dependent H2O2 production is required for salt-induced antioxidant defense in Arabidopsis thaliana. J. Plant Physiol. 174, 5–15. doi: 10.1016/j.jplph.2014.08.022
Blumwald, E. (2000). Sodium transport and salt tolerance in plants. Curr. Opin. Cell Biol. 12, 431–434. doi: 10.1016/S0955-0674(00)00112-5
Bonales-Alatorre, E., Pottosin, I., Shabala, L., Chen, Z. H., Zeng, F., Jacobsen, S. E., et al. (2013a). Differential activity of plasma and vacuolar membrane transporters contributes to genotypic differences in salinity tolerance in a halophyte species, Chenopodium quinoa. Int. J. Mol. Sci. 14, 9267–9285. doi: 10.3390/ijms14059267
Bonales-Alatorre, E., Shabala, S., Chen, Z. H., and Pottosin, I. (2013b). Reduced tonoplast fast-activating and slow-activating channel activity is essential for conferring salinity tolerance in a facultative halophyte, quinoa. Plant Physiol. 162, 940–952. doi: 10.1104/pp.113.216572
Bose, J., Pottosin, I. I., Shabala, S. S., Palmgren, M. G., and Shabala, S. (2011). Calcium efflux systems in stress signaling and adaptation in plants. Front. Plant Sci. 2:85. doi: 10.3389/fpls.2011.00085
Bose, J., Rodrigo-Moreno, A., and Shabala, S. (2014). ROS homeostasis in halophytes in the context of salinity stress tolerance. J. Exp. Bot. 65, 1241–1257. doi: 10.1093/jxb/ert430
Boudsocq, M., and Sheen, J. (2013). CDPKs in immune and stress signaling. Trends Plant Sci. 18, 30–40. doi: 10.1016/j.tplants.2012.08.008
Braam, J., and Davis, R. W. (1990). Rain-, wind-, and touch-induced expression of calmodulin and calmodulin-related genes in Arabidopsis. Cell 60, 357–364. doi: 10.1016/0092-8674(90)90587-5
Chaves, M. M., Flexas, J., and Pinheiro, C. (2009). Photosynthesis under drought and salt stress: regulation mechanisms from whole plant to cell. Ann. Bot. 103, 551–560. doi: 10.1093/aob/mcn125
Choi, W. G., Toyota, M., Kim, S. H., Hilleary, R., and Gilroy, S. (2014). Salt stress-induced Ca2+ waves are associated with rapid, long-distance root-to-shoot signaling in plants. Proc. Natl. Acad. Sci. U.S.A. 111, 6497–6502. doi: 10.1073/pnas.1319955111
Choudhury, S., Panda, P., Sahoo, L., and Panda, S. K. (2013). Reactive oxygen species signaling in plants under abiotic stress. Plant Signal. Behav. 8:e23681. doi: 10.4161/psb.23681
Chung, J. S., Zhu, J. K., Bressan, R. A., Hasegawa, P. M., and Shi, H. (2008). Reactive oxygen species mediate Na+-induced SOS1 mRNA stability in Arabidopsis. Plant J. 53, 554–565. doi: 10.1111/j.1365-313X.2007.03364.x
Coste, B., Mathur, J., Schmidt, M., Earley, T. J., Ranade, S., Petrus, M. J., et al. (2010). Piezo1 and Piezo2 are essential components of distinct mechanically activated cation channels. Science 330, 55–60. doi: 10.1126/science.1193270
de Boer, A. H., and Wegner, L. H. (1997). Regulatory mechanisms of ion channels in xylem parenchyma cells. J. Exp. Bot. 48, 441–449. doi: 10.1093/jxb/48.Special_Issue.441
Deinlein, U., Stephan, A. B., Horie, T., Luo, W., Xu, G., and Schroeder, J. I. (2014). Plant salt-tolerance mechanisms. Trends Plant Sci. 19, 371–379. doi: 10.1016/j.tplants.2014.02.001
Demidchik, V., Cuin, T. A., Svistunenko, D., Smith, S. J., Miller, A. J., Shabala, S., et al. (2010). Arabidopsis root K+-efflux conductance activated by hydroxyl radicals: single-channel properties, genetic basis and involvement in stress-induced cell death. J. Cell Sci. 123, 1468–1479. doi: 10.1242/jcs.064352
Donaldson, L., Ludidi, N., Knight, M. R., Gehring, C., and Denby, K. (2004). Salt and osmotic stress cause rapid increases in Arabidopsis thaliana cGMP levels. FEBS Lett. 569, 317–320. doi: 10.1016/j.febslet.2004.06.016
Drerup, M. M., Schlücking, K., Hashimoto, K., Manishankar, P., Steinhorst, L., Kuchitsu, K., et al. (2013). The Calcineurin B-like calcium sensors CBL1 and CBL9 together with their interacting protein kinase CIPK26 regulate the Arabidopsis NADPH oxidase RBOHF. Mol. Plant 6, 559–569. doi: 10.1093/mp/sst009
Dubiella, U., Seybold, H., Durian, G., Komander, E., Lassig, R., Witte, C. P., et al. (2013). Calcium-dependent protein kinase/NADPH oxidase activation circuit is required for rapid defense signal propagation. Proc. Natl. Acad. Sci. U.S.A. 110, 8744–8749. doi: 10.1073/pnas.1221294110
Dynowski, M., Schaaf, G., Loque, D., Moran, O., and Ludewig, U. (2008). Plant plasma membrane water channels conduct the signalling molecule H2O2. Biochem. J. 414, 53–61. doi: 10.1042/BJ20080287
Ellouzi, H., Ben Hamed, K., Hernández, I., Cela, J., Müller, M., Magné, C., et al. (2014). A comparative study of the early osmotic, ionic, redox and hormonal signaling response in leaves and roots of two halophytes and a glycophyte to salinity. Planta 240, 1299–1317. doi: 10.1007/s00425-014-2154-7
Ellouzi, H., Hamed, K. B., Cela, J., Munné-Bosch, S., and Abdelly, C. (2011). Early effects of salt stress on the physiological and oxidative status of Cakile maritima (halophyte) and Arabidopsis thaliana (glycophyte). Physiol. Plant. 142, 128–143. doi: 10.1111/j.1399-3054.2011.01450.x
Furuichi, T., Iida, H., Sokabe, M., and Tatsumi, H. (2012). Expression of Arabidopsis MCA1 enhanced mechanosensitive channel activity in the Xenopus laevis oocyte plasma membrane. Plant Signal. Behav. 7, 1022–1026. doi: 10.4161/psb.20783
Gilroy, S., Suzuki, N., Miller, G., Choi, W. G., Toyota, M., Devireddy, A. R., et al. (2014). A tidal wave of signals: calcium and ROS at the forefront of rapid systemic signaling. Trends Plant Sci. 19, 623–630. doi: 10.1016/j.tplants.2014.06.013
Haswell, E. S., Peyronnet, R., Barbier-Brygoo, H., Meyerowitz, E. M., and Frachisse, J. M. (2008). Two MscS homologs provide mechanosensitive channel activities in the Arabidopsis root. Curr. Biol. 18, 730–734. doi: 10.1016/j.cub.2008.04.039
Haswell, E. S., Phillips, R., and Rees, D. C. (2011). Mechanosensitive channels: what can they do and how do they do it? Structure 19, 1356–1369. doi: 10.1016/j.str.2011.09.005
Huh, G. H., Damsz, B., Matsumoto, T. K., Reddy, M. P., Rus, A. M., Ibeas, J. I., et al. (2002). Salt causes ion disequilibrium-induced programmed cell death in yeast and plants. Plant J. 29, 649–659. doi: 10.1046/j.0960-7412.2001.01247.x
Jiang, C., Belfield, E. J., Mithani, A., Visscher, A., Ragoussis, J., Mott, R., et al. (2012). ROS-mediated vascular homeostatic control of root-to-shoot soil Na delivery in Arabidopsis. EMBO J. 31, 4359–4370. doi: 10.1038/emboj.2012.273
Kader, M. A., and Lindberg, S. (2010). Cytosolic calcium and pH signaling in plants under salinity stress. Plant Signal. Behav. 5, 233–238. doi: 10.4161/psb.5.3.10740
Kadota, Y., Sklenar, J., Derbyshire, P., Stransfeld, L., Asai, S., Ntoukakis, V., et al. (2014). Direct regulation of the NADPH oxidase RBOHD by the PRR-associated kinase BIK1 during plant immunity. Mol. Cell 54, 43–55. doi: 10.1016/j.molcel.2014.02.021
Kanzaki, M., Nagasawa, M., Kojima, I., Sato, C., Naruse, K., Sokabe, M., et al. (1999). Molecular identification of a eukaryotic, stretch-activated nonselective cation channel. Science 285, 882–886. doi: 10.1126/science.285.5429.882
Kärkönen, A., and Kuchitsu, K. (2015). Reactive oxygen species in cell wall metabolism and development in plants. Phytochemistry 112, 22–32. doi: 10.1016/j.phytochem.2014.09.016
Kiegle, E., Moore, C. A., Haseloff, J., Tester, M. A., and Knight, M. R. (2000). Cell-type-specific calcium responses to drought, salt and cold in the Arabidopsis root. Plant J. 23, 267–278. doi: 10.1046/j.1365-313x.2000.00786.x
Kimura, S., Kawarazaki, T., Nibori, H., Michikawa, M., Imai, A., Kaya, H., et al. (2013). The CBL-interacting protein kinase CIPK26 is a novel interactor of Arabidopsis NADPH oxidase AtRbohF that negatively modulates its ROS-producing activity in a heterologous expression system. J. Biochem. 153, 191–195. doi: 10.1093/jb/mvs132
Kimura, S., Kaya, H., Kawarazaki, T., Hiraoka, G., Senzaki, E., Michikawa, M., et al. (2012). Protein phosphorylation is a prerequisite for the Ca2+-dependent activation of Arabidopsis NADPH oxidases and may function as a trigger for the positive feedback regulation of Ca2+ and reactive oxygen species. Biochim. Biophys. Acta 1823, 398–405. doi: 10.1016/j.bbamcr.2011.09.011
Knight, H., Trewavas, A. J., and Knight, M. R. (1997). Calcium signalling in Arabidopsis thaliana responding to drought and salinity. Plant J. 12, 1067–1078. doi: 10.1046/j.1365-313X.1997.12051067.x
Kobayashi, M., Ohura, I., Kawakita, K., Yokota, N., Fujiwara, M., Shimamoto, K., et al. (2007). Calcium-dependent protein kinases regulate the production of reactive oxygen species by potato NADPH oxidase. Plant Cell 19, 1065–1080. doi: 10.1105/tpc.106.048884
Kuchitsu, K., Kosaka, H., Shiga, T., and Shibuya, N. (1995). EPR evidence for generation of hydroxyl radical triggered by N-acetylchitooligosaccharide elicitor and a protein phosphatase inhibitor in suspension-cultured rice cells. Protoplasma 188, 138–142. doi: 10.1007/BF01276805
Kurusu, T., Kuchitsu, K., Nakano, M., Nakayama, Y., and Iida, H. (2013). Plant mechanosensing and Ca2+ transport. Trends Plant Sci. 18, 227–233. doi: 10.1016/j.tplants.2012.12.002
Kurusu, T., Nishikawa, D., Yamazaki, Y., Gotoh, M., Nakano, M., Hamada, H., et al. (2012a). Plasma membrane protein OsMCA1 is involved in regulation of hypo-osmotic shock-induced Ca2+ influx and modulates generation of reactive oxygen species in cultured rice cells. BMC Plant Biol. 12:11. doi: 10.1186/1471-2229-12-11
Kurusu, T., Yamanaka, T., Nakano, M., Takiguchi, A., Ogasawara, Y., Hayashi, T., et al. (2012b). Involvement of the putative Ca2+-permeable mechanosensitive channels, NtMCA1 and NtMCA2, in Ca2+ uptake, Ca2+-dependent cell proliferation and mechanical stress-induced gene expression in tobacco (Nicotiana tabacum) BY-2 cells. J. Plant Res. 125, 555–568. doi: 10.1007/s10265-011-0462-6
Laohavisit, A., and Davies, J. M. (2011). Annexins. New Phytol. 189, 40–53. doi: 10.1111/j.1469-8137.2010.03533.x
Laohavisit, A., Richards, S. L., Shabala, L., Chen, C., Colaço, R. D., Swarbreck, S. M., et al. (2013). Salinity-induced calcium signaling and root adaptation in Arabidopsis require the calcium regulatory protein annexin1. Plant Physiol. 163, 253–262. doi: 10.1104/pp.113.217810
Laohavisit, A., Shang, Z., Rubio, L., Cuin, T. A., Véry, A. A., Wang, A., et al. (2012). Arabidopsis annexin1 mediates the radical-activated plasma membrane Ca2+- and K+-permeable conductance in root cells. Plant Cell 24, 1522–1533. doi: 10.1105/tpc.112.097881
Lecourieux, D., Ranjeva, R., and Pugin, A. (2006). Calcium in plant defence-signalling pathways. New Phytol. 171, 249–269. doi: 10.1111/j.1469-8137.2006.01777.x
Lee, S., Lee, E. J., Yang, E. J., Lee, J. E., Park, A. R., Song, W. H., et al. (2004). Proteomic identification of annexins, calcium-dependent membrane binding proteins that mediate osmotic stress and abscisic acid signal transduction in Arabidopsis. Plant Cell 16, 1378–1391. doi: 10.1105/tpc.021683
Ma, L., Zhang, H., Sun, L., Jiao, Y., Zhang, G., Miao, C., et al. (2012). NADPH oxidase AtrbohD and AtrbohF function in ROS-dependent regulation of Na+/K+ homeostasis in Arabidopsis under salt stress. J. Exp. Bot. 63, 305–317. doi: 10.1093/jxb/err280
Marino, D., Dunand, C., Puppo, A., and Pauly, N. (2012). A burst of plant NADPH oxidases. Trends Plant Sci. 17, 9–15. doi: 10.1016/j.tplants.2011.10.001
Monshausen, G. B., Bibikova, T. N., Weisenseel, M. H., and Gilroy, S. (2009). Ca2+ regulates reactive oxygen species production and pH during mechanosensing in Arabidopsis roots. Plant Cell 21, 2341–2356. doi: 10.1105/tpc.109.068395
Monshausen, G. B., and Gilroy, S. (2009). Feeling green: mechanosensing in plants. Trends Cell Biol. 19, 228–235. doi: 10.1016/j.tcb.2009.02.005
Munns, R., and Tester, M. (2008). Mechanisms of salinity tolerance. Annu. Rev. Plant Biol. 59, 651–681. doi: 10.1146/annurev.arplant.59.032607.092911
Mylona, P. V., Polidoros, A. N., and Scandalios, J. G. (2007). Antioxidant gene responses to ROS-generating xenobiotics in developing and germinated scutella of maize. J. Exp. Bot. 58, 1301–1312. doi: 10.1093/jxb/erl292
Nakagawa, Y., Katagiri, T., Shinozaki, K., Qi, Z., Tatsumi, H., Furuichi, T., et al. (2007). Arabidopsis plasma membrane protein crucial for Ca2+ influx and touch sensing in roots. Proc. Natl. Acad. Sci. U.S.A. 104, 3639–3644. doi: 10.1073/pnas.0607703104
Nakano, M., Iida, K., Nyunoya, H., and Iida, H. (2011). Determination of structural regions important for Ca2+ uptake activity in Arabidopsis MCA1 and MCA2 expressed in yeast. Plant Cell Physiol. 52, 1915–1930. doi: 10.1093/pcp/pcr131
O’Brien, J. A., Daudi, A., Butt, V. S., and Bolwell, G. P. (2012). Reactive oxygen species and their role in plant defence and cell wall metabolism. Planta 236, 765–779. doi: 10.1007/s00425-012-1696-9
Ogasawara, Y., Kaya, H., Hiraoka, G., Yumoto, F., Kimura, S., Kadota, Y., et al. (2008). Synergistic activation of the Arabidopsis NADPH oxidase AtrbohD by Ca2+ and phosphorylation. J. Biol. Chem. 283, 8885–8892. doi: 10.1074/jbc.M708106200
Pandey, N., Ranjan, A., Pant, P., Tripathi, R. K., Ateek, F., Pandey, H. P., et al. (2013). CAMTA 1 regulates drought responses in Arabidopsis thaliana. BMC Genomics 14:216. doi: 10.1186/1471-2164-14-216
Pottosin, I., Velarde-Buendía, A. M., Bose, J., Zepeda-Jazo, I., Shabala, S., and Dobrovinskaya, O. (2014). Cross-talk between reactive oxygen species and polyamines in regulation of ion transport across the plasma membrane: implications for plant adaptive responses. J. Exp. Bot. 65, 1271–1283. doi: 10.1093/jxb/ert423
Reguera, M., Bassil, E., and Blumwald, E. (2014). Intracellular NHX-type cation/H+ antiporters in plants. Mol. Plant 7, 261–263. doi: 10.1093/mp/sst091
Rengasamy, P. (2006). World salinization with emphasis on Australia. J. Exp. Bot. 57, 1017–1023. doi: 10.1093/jxb/erj108
Roach, T., and Kranner, I. (2011). Extracellular superoxide production associated with secondary root growth following desiccation of Pisum sativum seedlings. J. Plant Physiol. 168, 1870–1873. doi: 10.1016/j.jplph.2011.04.010
Rodrigo-Moreno, A., Andrés-Colás, N., Poschenrieder, C., Gunsé, B., Peñarrubia, L., and Shabala, S. (2013). Calcium- and potassium-permeable plasma membrane transporters are activated by copper in Arabidopsis root tips: linking copper transport with cytosolic hydroxyl radical production. Plant Cell Environ. 36, 844–855. doi: 10.1111/pce.12020
Sani, E., Herzyk, P., Perrella, G., Colot, V., and Amtmann, A. (2013). Hyperosmotic priming of Arabidopsis seedlings establishes a long-term somatic memory accompanied by specific changes of the epigenome. Genome Biol. 14:R59. doi: 10.1186/gb-2013-14-6-r59
Shabala, S. (2009). Salinity and programmed cell death: unravelling mechanisms for ion specific signalling. J. Exp. Bot. 60, 709–712. doi: 10.1093/jxb/erp013
Shabala, S., and Newman, I. (2000). Salinity effects on the activity of plasma membrane H+ and Ca2+ transporters in bean leaf mesophyll: masking role of the cell wall. Ann. Bot. 85, 681–686. doi: 10.1006/anbo.2000.1131
Shabala, S., and Pottosin, I. (2014). Regulation of potassium transport in plants under hostile conditions: implications for abiotic and biotic stress tolerance. Physiol. Plant. 151, 257–279. doi: 10.1111/ppl.12165
Shi, H., Ishitani, M., Kim, C., and Zhu, J. K. (2000). The Arabidopsis thaliana salt tolerance gene SOS1 encodes a putative Na+/H+ antiporter. Proc. Natl. Acad. Sci. U.S.A. 97, 6896–6901. doi: 10.1073/pnas.120170197
Shigematsu, H., Iida, K., Nakano, M., Chaudhuri, P., Iida, H., and Nagayama, K. (2014). Structural characterization of the mechanosensitive channel candidate MCA2 from Arabidopsis thaliana. PLoS ONE 9:e87724. doi: 10.1371/journal.pone.0087724
Sirichandra, C., Gu, D., Hu, H. C., Davanture, M., Lee, S., Djaoui, M., et al. (2009). Phosphorylation of the Arabidopsis AtrbohF NADPH oxidase by OST1 protein kinase. FEBS Lett. 583, 2982–2986. doi: 10.1016/j.febslet.2009.08.033
Song, Y., Ji, D., Li, S., Wang, P., Li, Q., and Xiang, F. (2012). The dynamic changes of DNA methylation and histone modifications of salt responsive transcription factor genes in soybean. PLoS ONE 7:e41274. doi: 10.1371/journal.pone.0041274
Spoel, S. H., and Loake, G. J. (2011). Redox-based protein modifications: the missing link in plant immune signalling. Curr. Opin. Plant Biol. 14, 358–364. doi: 10.1016/j.pbi.2011.03.007
Srivastava, A. K., Srivastava, S., Lokhande, V. H., D’souza, S. F., and Suprasanna, P. (2015). Salt stress reveales differential antioxidant and energetics responses in glycophyte (Brassica juncea L.) and halophyte (Sesuvium portulacastrum L.). Front. Environ. Sci. 3:19. doi: 10.3389/fenvs.2015.00019
Sumimoto, H. (2008). Structure, regulation and evolution of Nox-family NADPH oxidases that produce reactive oxygen species. FEBS J. 275, 3249–3277. doi: 10.1111/j.1742-4658.2008.06488.x
Sunarpi, Horie, T., Motoda, J., Kubo, M., Yang, H., Yoda, K., et al. (2005). Enhanced salt tolerance mediated by AtHKT1 transporter-induced Na+ unloading from xylem vessels to xylem parenchyma cells. Plant J. 44, 928–938. doi: 10.1111/j.1365-313X.2005.02595.x
Tada, Y., Komatsubara, S., and Kurusu, T. (2014). Growth and physiological adaptation of whole plants and cultured cells from a halophyte turf grass under salt stress. AoB Plants 6:plu041. doi: 10.1093/aobpla/plu041
Takeda, S., Gapper, C., Kaya, H., Bell, E., Kuchitsu, K., and Dolan, L. (2008). Local positive feedback regulation determines cell shape in root hair cells. Science 319, 1241–1244. doi: 10.1126/science.1152505
Tracy, F. E., Gilliham, M., Dodd, A. N., Webb, A. A., and Tester, M. (2008). NaCl-induced changes in cytosolic free Ca2+ in Arabidopsis thaliana are heterogeneous and modified by external ionic composition. Plant Cell Environ. 31, 1063–1073. doi: 10.1111/j.1365-3040.2008.01817.x
Urao, T., Yakubov, B., Satoh, R., Yamaguchi-Shinozaki, K., Seki, M., Hirayama, T., et al. (1999). A transmembrane hybrid-type histidine kinase in Arabidopsis functions as an osmosensor. Plant Cell 11, 1743–1754. doi: 10.1105/tpc.11.9.1743
Wang, Q., Wu, C., Xie, B., Liu, Y., Cui, J., Chen, G., et al. (2012). Model analysing the antioxidant responses of leaves and roots of switchgrass to NaCl-salinity stress. Plant Physiol. Biochem. 58, 288–296. doi: 10.1016/j.plaphy.2012.06.021
Weinl, S., and Kudla, J. (2009). The CBL-CIPK Ca2+-decoding signaling network: function and perspectives. New Phytol. 184, 517–528. doi: 10.1111/j.1469-8137.2009.02938.x
Wohlbach, D. J., Quirino, B. F., and Sussman, M. R. (2008). Analysis of the Arabidopsis histidine kinase ATHK1 reveals a connection between vegetative osmotic stress sensing and seed maturation. Plant Cell 20, 1101–1117. doi: 10.1105/tpc.107.055871
Wormit, A., Butt, S. M., Chairam, I., McKenna, J. F., Nunes-Nesi, A., Kjaer, L., et al. (2012). Osmosensitive changes of carbohydrate metabolism in response to cellulose biosynthesis inhibition. Plant Physiol. 159, 105–117. doi: 10.1104/pp.112.195198
Xie, Y. J., Xu, S., Han, B., Wu, M. Z., Yuan, X. X., Han, Y., et al. (2011). Evidence of Arabidopsis salt acclimation induced by up-regulation of HY1 and the regulatory role of RbohD-derived reactive oxygen species synthesis. Plant J. 66, 280–292. doi: 10.1111/j.1365-313X.2011.04488.x
Xu, P., Liu, Z., Fan, X., Gao, J., Zhang, X., Zhang, X., et al. (2013). De novo transcriptome sequencing and comparative analysis of differentially expressed genes in Gossypium aridum under salt stress. Gene 525, 26–34. doi: 10.1016/j.gene.2013.04.066
Yamanaka, T., Nakagawa, Y., Mori, K., Nakano, M., Imamura, T., Kataoka, H., et al. (2010). MCA1 and MCA2 that mediate Ca2+ uptake have distinct and overlapping roles in Arabidopsis. Plant Physiol. 152, 1284–1296. doi: 10.1104/pp.109.147371
Keywords: Ca2+-permeable channels, NADPH oxidases (Noxs), osmotic stress, reactive oxygen species (ROS), salinity stress
Citation: Kurusu T, Kuchitsu K and Tada Y (2015) Plant signaling networks involving Ca2+ and Rboh/Nox-mediated ROS production under salinity stress. Front. Plant Sci. 6:427. doi: 10.3389/fpls.2015.00427
Received: 31 March 2015; Accepted: 26 May 2015;
Published online: 10 June 2015.
Edited by:
Wim Van den Ende, KU Leuven, BelgiumReviewed by:
Eric Van Der Graaff, Copenhagen University, DenmarkSergey Shabala, University of Tasmania, Australia
Copyright © 2015 Kurusu, Kuchitsu and Tada. This is an open-access article distributed under the terms of the Creative Commons Attribution License (CC BY). The use, distribution or reproduction in other forums is permitted, provided the original author(s) or licensor are credited and that the original publication in this journal is cited, in accordance with accepted academic practice. No use, distribution or reproduction is permitted which does not comply with these terms.
*Correspondence: Takamitsu Kurusu, School of Bioscience and Biotechnology, Tokyo University of Technology, 1404-1 Katakura, Hachioji, Tokyo 192-0982, Japan, kurusutkmt@stf.teu.ac.jp