- 1Institute of Transformative Bio-Molecules (WPI-ITbM), Nagoya University, Nagoya, Japan
- 2Department of Biology, University of Washington, Seattle, WA, USA
- 3Howard Hughes Medical Institute, University of Washington, Seattle, WA, USA
Aboveground organs of plants are ultimately derived/generated from the shoot apical meristem (SAM), which is a proliferative tissue located at the apex of the stem. The SAM contains a population of stem cells that provide new cells for organ/tissue formation. The SAM is composed of distinct cell layers and zones with different properties. Primordia of lateral organs develop at the periphery of the SAM. The shoot apex is a dynamic and complex tissue, and as such intercellular communications among cells, layers and zones play significant roles in the coordination of cell proliferation, growth and differentiation to achieve elaborate morphogenesis. Recent findings have highlighted the importance of a number of signaling molecules acting in the cell wall space for the intercellular communication, including classic phytohormones and secretory peptides. Moreover, accumulating evidence has revealed that cell wall properties and their modifying enzymes modulate hormone actions. In this review, we outline how behaviors of signaling molecules and changes of cell wall properties are integrated for the shoot meristem regulation.
Introduction
Plants elongate stems upward, expand leaves widely to perceive the sunlight, and form flowers for reproduction. These aboveground organs are all developed from the shoot apical meristem (SAM), the proliferative tissue located at the top of the stem. The SAM contains a population of stem cells, which are characterized by two functions: providing new cells for organ/tissue formation and maintaining their own undifferentiated population. In typical eudicots, the SAM is composed of three clonally distinct cell layers, L1, L2, and L3, from outermost to inner cell layers (Steeves and Sussex, 1989; Bowman and Eshed, 2000). Cells in the L1 and L2 layers divide anticlinally, thus serving as the source of epidermal/subepidermal tissues that cover whole aerial parts. On the other hand, cells in the L3 layer divide in various planes to give rise to other inner tissues. The SAM can also be divided into three zones with different anatomical properties: the central zone (CZ) at the top, the peripheral zone (PZ), and the rib zone (RZ) beneath the CZ (Steeves and Sussex, 1989; Bowman and Eshed, 2000). The CZ contains less mitotically active stem cells, replenishing cells for the surrounding PZ and RZ, where cells divide more rapidly and give rise to lateral organs and stems. Primordia of leaves and flowers are formed successively at the periphery of the SAM with a constant divergent angle, resulting in beautifully arranged patterns of the lateral organs (phyllotaxis; Jean, 1994). During organ initiation, cells in a corresponding part of the PZ swell and divide to form a bulge-shaped primordium, and they further proliferate to form a lateral organ.
Intercellular communication plays crucial roles for the coordination among cells and tissues in the shoot apex to achieve elaborate morphogenesis of the plant body. Classically, a series of surgical experiments have predicted the presence of such signals (Steeves and Sussex, 1989). Recent molecular studies have revealed that not only small molecular weight phytohormones but also secretory peptides mediate intercellular signaling. Furthermore, accumulating evidence has highlighted the importance of the extracellular cell wall space for hormone actions. In this review, we outline intercellular communication in the shoot apex, especially focusing on events in the extracellular space, such as hormone transport, metabolism and the dynamics of the cell wall properties.
Auxin Transport through the Extracellular Space is Regulated by Cell Wall Properties
Among phytohormones, auxin has been the best characterized in shoot development (Davies, 1995). Auxin regulates a range of developmental processes in specific cells. The following two perception systems for auxin have been reported to mediate the auxin action. Firstly, a group of nuclear-localized receptors encoded by TIR1 (TRANSPORT INHIBITOR RESPONSE 1)/AFB (AUXIN F-BOX) genes perceive auxin. In the presence of auxin, the receptors activate ARF (AUXIN RESPONSE FACTOR) family transcription factors to regulate their target genes (Guilfoyle and Hagen, 2007; Salehin et al., 2015). Secondly, an extracellular receptor, ABP1 (AUXIN BINDING PROTEIN1), binds to auxin (Löbler and Klämbt, 1985). Auxin activates ROP (RHO OF PLANTS) signaling in an ABP1-dependent manner, which in turn regulates clathrin-coated vesicle trafficking and cytoskeleton dynamics (Robert et al., 2010; Xu et al., 2010; Nagawa et al., 2012; Grones and Friml, 2015). In the L1 layer of the SAM, auxin accumulation induces formation of lateral organ primordia. Thus the auxin distribution pattern determines the phyllotaxis. The accumulated auxin in the L1 layer is subsequently transported into underlying L2 and L3 tissues (Benková et al., 2003; Reinhardt et al., 2003; Heisler et al., 2005; Bayer et al., 2009). This auxin flow induces vascular differentiation that eventually connects a vascular network from the primordium to stem vasculatures (Bayer et al., 2009). In a growing primordium, auxin is again accumulated in a dotted pattern in the marginal epidermis, inducing formation of leaflets, lobes, serration and venation (Hay et al., 2006; Scarpella et al., 2006; Barkoulas et al., 2008).
The major natural auxin, indole-3-acetic acid (IAA), is synthesized by intracellular enzymes, which are expressed with specific spatio-temporal patterns (Zhao, 2008; Chandler, 2009). Then, intercellular transport of auxin from the biosynthesis sites determines its distribution pattern. Auxin transport is facilitated by plasma membrane-localized auxin efflux carrier protein, PIN1 (PIN-FORMED 1). Genetic and chemical perturbation of PIN1 impairs the local auxin accumulation and following developmental events: lateral organ initiation (Okada et al., 1991; Reinhardt et al., 2000), phyllotaxis (Guenot et al., 2012), vascular patterning (Scarpella et al., 2006), and leaf margin morphogenesis (Hay et al., 2006). In these events, PIN1 proteins localize at a specific part of the plasma membrane and the coherent PIN1 polarization in a group of cells causes directional auxin flow toward the point of its accumulation (Boutté et al., 2007; Nakamura et al., 2012; Luschnig and Vert, 2014). Studies on mechanisms for the PIN1 polarization proposed that PIN1 proteins preferentially localize toward the most auxin-rich neighboring cell or toward the cell wall where the auxin flux is the highest (Jönsson et al., 2006; Smith et al., 2006; Stoma et al., 2008; Bayer et al., 2009). It was also proposed that PIN1 proteins concentrate toward the cell wall that exhibits the highest stress (Heisler et al., 2010; Nakayama et al., 2012; Braybrook and Peaucelle, 2013). These two types of models have started to be integrated by recent studies and the details of this integration will be described in the later section of this review on physical properties of the cell wall. These findings highlight a novel role for cell walls in the regulation of auxin distribution. It has been discussed that, in the SAM and leaf primordia, PIN1 dynamics might be differently regulated between the epidermal L1 layer and the inner L2/L3 tissues (Tsugeki et al., 2009; O’Connor et al., 2014). Because the proposed models for stress responses only dealt with phenomenon in the L1 layer, it would be important to investigate whether these models can be applied to the L2/L3 tissues.
The auxin distribution pattern also depends on its influx into cells since mutations in auxin influx carrier genes lead to abnormal phyllotaxis (Cheng et al., 2007; Bainbridge et al., 2008). Interestingly, the auxin influx rate is affected by a chemical property of cell wall space. Major auxin influx carriers, AUX1/LAX (LIKE-AUX1) proteins, catalyze H+/IAA-symport (Yang et al., 2006; Boutté et al., 2007; Vieten et al., 2007). Therefore, this process largely depends on the pH difference across the plasma membrane. In addition, the auxin influx occurs by diffusion. Because the extracellular pH is acidic (∼5.5) and the cytosolic pH is neutral (∼7.0), a substantial amount of IAA is protonated in the extracellular space and is ionized in the cytosol. As only the protonated form is freely transported through lipophilic plasma membranes because of its neutral charge, the diffusion is unidirectional from outside of the cell to the inside. Plants indeed alter the extracellular pH to create the auxin gradient in hypocotyls by utilizing plasma membrane-localized H+-ATPases (Hohm et al., 2014).
Cytokinin also Acts for Intercellular Communication
Cytokinins (CKs) are adenine derivatives, which also act as a phytohormone playing significant roles in the SAM activity and organ morphogenesis. Indeed, two key transcription factors for the SAM regulation, SHOOT MERISTEMLESS (STM) and WUSCHEL (WUS), control the CK homeostasis and signaling, highlighting the importance of CK for the regulation (Yanai et al., 2005; Gordon et al., 2009). CK also impacts phyllotaxis (Giulini et al., 2004), lateral organ initiation (Yoshida et al., 2011), leaf margin morphogenesis (Greenboim-Wainberg et al., 2005; Shani et al., 2010; Efroni et al., 2013), and leaf vein patterning (Werner et al., 2003).
Cytokinins is transported not only locally among neighboring cells but also distantly between shoot and root. CK moves from shoot to root symplastically via phloem and from root to shoot apoplastically via xylem (Kudo et al., 2010). The intercellular CK movement is facilitated by both transporters and passive diffusion. ENT (EQUILIBRATIVE NUCLEOSIDE TRANSPORTER) and PUP (PURINE PERMEASE) proteins act as importers (Bürkle et al., 2003; Li et al., 2003; Hirose et al., 2005; Sun et al., 2005; Cedzich et al., 2008; Qi and Xiong, 2013). On the other hand, ABCG14 (ATP-BINDING CASSETTE TRANSPORTER SUBFAMILY G14) was implicated as an exporter candidate. Some of ENTs and PUPs are expressed in shoot tissues and their mutations change shoot morphologies. However, a large and complex redundancy among these family members hampers comprehensive analysis to further investigate roles for these transporters. The ABCG14 loss-of-function mutation alters the SAM size and growth rate. Interestingly, grafting experiments indicate that the ABCG14 expression in root is essential for shoot development (Ko et al., 2014), demonstrating the significance of the CK translocation between shoot and root for the shoot development. On the other hand, the importance of the CK transporters for the local movement is still unclear. At least, in contrast to auxin transport, highly directional transport is not assumed and simple diffusion of CK is predicted owing to its high membrane permeability (Laloue et al., 1981; Kudoyarova et al., 2014). Accordingly, recent mathematical models have reconstituted expression patterns of CK-responsive genes for the SAM regulation and vascular formation by assuming only simple and constant diffusion for the CK transport (Chickarmane et al., 2012; De Rybel et al., 2014).
Enzymes responsible for CK biosynthesis are localized in cytosol and intracellular organelles (Kieber and Schaller, 2014), while the degradation is catalyzed by CKX (CYTOKININ DEHYDROGENASE) family members, some of which are reported to act as extracellular proteins (Motyka et al., 2003; Werner et al., 2003; Kieber and Schaller, 2014). In addition, CKX members show different substrate preferences and expression patterns (Werner et al., 2003; Gajdošová et al., 2011; Köllmer et al., 2014). These imply that each CKX degrades a specific pool of CK according to their particular spatial distribution and substrate preference. While it was shown that the mutant of a putative extracellular CKX, CKX5, displays defects of the SAM and stem morphologies (Bartrina et al., 2011), the biological significance of the extracellular CK degradation is still largely obscure. It was reported that, in moss, the extracellular amount of CK correlates with some developmental alterations rather than its intracellular amount (von Schwartzenberg et al., 2007).
Crosstalks between Auxin- and CK-Related Regulations of SAM Development
As mentioned above, auxin and CK play important roles in the shoot apex development. Interestingly, in recent years, crosstalk between these hormone pathways has been revealed (Su et al., 2011; Murray et al., 2012; Schaller et al., 2015). For example, PIN1-dependent auxin accumulation leads to the down-regulation of STM that promotes CK biosynthesis (Furutani et al., 2004; Heisler et al., 2005; Yanai et al., 2005). Auxin also modulates the cellular responses to CK by changing the expression of negative regulators of CK signaling cascade (Zhao et al., 2010; Besnard et al., 2014); up-regulating ARR7 (ARABIDOPSIS RESPONSE REGULATOR 7) and ARR15 and down-regulating AHP6 (ARABIDOPSIS HISTIDINE PHOSPHOTRANSFER PROTEIN 6). On the other hand, CK affects the PIN1 expression level in the SAM, suggesting that CK may modulate the auxin transport rate (Lee et al., 2009). In addition, the PIN1 polarization is influenced by the CK in the roots (Bishopp et al., 2011; Marhavý et al., 2014), though it remains to be examined whether the same mechanism also functions in the SAM. Mutual regulation between auxin and CK provides robustness and dynamics of the SAM homeostasis (Besnard et al., 2014). Meanwhile, these complex systems make it difficult to understand the whole picture only by traditional types of experiments and observations. Computer-based mathematical modeling approaches may provide solutions to this issue. Indeed, a successful case has been reported on the mutual regulation between auxin and CK in embryogenesis (De Rybel et al., 2014).
Peptide Hormones and Receptors Regulating SAM Development
Peptide hormones are another important class of signaling molecules mediating intercellular communication through the cell wall space. Since the discovery of 18 amino-acid systemin as a defense response signal in tomato (Pearce et al., 1991), a variety of signaling peptides have been identified in plants. All of these peptides are produced via proteolytic cleavage of precursor proteins encoded by specific genes. Although the systemin was shown to localize in the cytosol (Narváez-Vásquez and Ryan, 2004), most of the others are presumed to be secreted into the apoplasts by the N-terminal signal peptide (Matsubayashi, 2014). These secreted peptides bind to specific cell-surface-localized receptors at target cells and affect cellular behaviors such as cell division, cell morphogenesis and cell fate determination. Peptide hormone genes are classified into distinct families based on the similarity in their mature peptide sequences. Each family contains multiple genes, which produce structurally similar or even identical mature peptides. Post-translational modification is also critical for their bioactivities. Secreted peptides often contain proline, tyrosine and/or cysteine residues post-translationally modified by specific enzymes (Komori et al., 2009; Ogawa-Ohnishi et al., 2013; Matsubayashi, 2014). Also, some proteases are responsible for the proteolytic cleavage in the maturation steps (Berger and Altmann, 2000; Srivastava et al., 2008; Tamaki et al., 2013; Engineer et al., 2014).
In the SAM, CLAVATA3 (CLV3) peptide, a member of CLE (CLV3/ESR-related) peptide family, mediates intercellular communications maintaining stem cell homeostasis (Cock and McCormick, 2001). The structure of the mature CLV3 peptide was determined as a 13 amino-acid peptide containing two hydroxyproline residues, one of which was further triarabinosylated (Ohyama et al., 2009). The CLV3 peptide is produced by stem cells in the CZ and perceived by its receptors at neighboring cells (Fletcher et al., 1999; Brand et al., 2000; Schoof et al., 2000). Leucine-rich repeat (LRR)-type receptors participate in the CLV3 signaling pathway. CLV1 is an LRR receptor kinase (LRR-RK), which binds to CLV3 (Ogawa et al., 2008; Ohyama et al., 2009). The BAM (BARELY ANY MEISTEM) proteins are close relatives of CLV1. Genetic and biochemical studies implied that BAMs also possess the binding capability to CLV3 peptides (DeYoung et al., 2006; DeYoung and Clark, 2008; Shinohara et al., 2012). In addition, other membrane-localized proteins including CLV2, CRN (CORYNE)/SOL2 (SUPPRESSOR OF LLP1 2) and RPK2 (RECEPTOR-LIKE PROTEIN KINASE 2) are involved in the CLV3 pathway (Miwa et al., 2008; Müller et al., 2008; Kinoshita et al., 2010). These proteins form various combinations of receptor complexes to mediate CLV3 signaling (Bleckmann et al., 2010; Guo et al., 2010; Kinoshita et al., 2010; Zhu et al., 2010). The CLV3 signal represses the expression of WUS that encodes a transcription factor promoting the proliferation of CLV3-producing stem cells (Mayer et al., 1998; Brand et al., 2000; Schoof et al., 2000). This local feedback is integrated with CK and auxin signaling, thus maintaining the dynamic SAM architecture (Leibfried et al., 2005; Gordon et al., 2009; Zhao et al., 2010; Chickarmane et al., 2012).
Phytosulfokine (PSK) is a disulfated five amino-acid peptide identified first in the Asparagus cell culture as a factor that promotes cell proliferation (Matsubayashi and Sakagami, 1996). Five genes produce the identical PSK peptides in Arabidopsis and are expressed in almost all tissues (Matsubayashi et al., 2006). PLANT PEPTIDE CONTAINING SULFATED TYROSINE 1 (PSY1), 18 amino acid glycopeptide, displays a biological activity similar to PSK (Amano et al., 2007). A PSK receptor (PSKR) was biochemically identified from carrot Daucus carota microsomal fractions (Matsubayashi et al., 2002). Arabidopsis homologs of DcPSKR act as receptors for PSK or PSY1, being expressed in a broad range of tissues including the SAM (Matsubayashi et al., 2006; Amano et al., 2007). Genetic studies of the receptors highlight the importance of PSK/PSY1 signaling in the cell proliferation, expansion and wound repair (Matsubayashi et al., 2006; Amano et al., 2007).
ERECTA (ER)-family LRR-RKs regulate various aspects of growth and development such as stem growth, stomatal patterning and the SAM regulation (Torii et al., 1996; Shpak et al., 2004; Uchida et al., 2011, 2013; Chen et al., 2013). The loss of the entire family activities impairs the SAM homeostasis, leading to an increase in stem cell population (Uchida et al., 2013). Because the SAM of er-family mutant shows enhanced CK response and abnormal auxin accumulation pattern (Chen et al., 2013; Uchida et al., 2013), ER-family signaling is thought to have crosstalks with CK and auxin pathways. Although a ligand for the ER family in the SAM regulation is still unknown, the ligands for stem growth and stomatal development have been identified: All of them belong to the same family of peptide hormones, the EPF (EPIDERMAL PATTERNING FACTOR)/EPFL (EPF-LIKE) family (Hara et al., 2007, 2009; Hunt and Gray, 2009; Abrash and Bergmann, 2010; Hunt et al., 2010; Kondo et al., 2010; Sugano et al., 2010; Abrash et al., 2011; Uchida et al., 2012, 2013). The EPF/EPFL family encodes cysteine-rich secretory peptides, which possess intramolecular disulfide bonds essential for the conformation of their bioactive mature forms (Kondo et al., 2010; Sugano et al., 2010; Ohki et al., 2011). A yet unknown ligand for ER family for the SAM regulation may also be a member of this family.
To date, more than 15 peptide hormone families have been described (Kondo et al., 2014). Though each family consists of multiple paralogs, roles for most of them are still obscure. Also, it is expected that yet unidentified peptide hormone genes can be found in genomes. It would be an important challenge to identify further secretory peptides, which participate in the shoot apex regulation.
Cell Wall Loosening Proteins as Key Factors in Organ Formation
Plant cells need to extend their cell walls during growth and development. Cell wall extensibility is enhanced by growth promoting phytohormones, including auxin and CK (Cleland, 1958; Thomas et al., 1981), and has started to be recognized as a key factor that determines developmental patterns and cell fates. The first breakthrough came from experiments using an apoplastic protein, EXP (EXPANSIN; Fleming et al., 1997; Pien et al., 2001), which relaxes cellulose microfibril network by binding to xyloglucan (Wang et al., 2013). The EXP expression increases in newly formed leaf primordia. Because its wall-loosening activity exhibits an acidic pH optimum, it is suggested that auxin accumulation in leaf primordia further increases the EXP activity (McQueen-Mason and Cosgrove, 1994; Cleland, 2001). It was also reported that local application of exogenous EXP proteins as well as artificial expression of EXP induces leaf formation on the SAM (Fleming et al., 1997). These indicate that local loosening of the cell wall triggers organ initiation. Auxin is also known to promote demethylesterification of pectin (Bryan and Newcomb, 1954; Braybrook and Peaucelle, 2013), and another secreted enzyme, PME (PECTIN METHYLESTERASE), was shown to loosen cell walls by demethylesterifying pectin (Peaucelle et al., 2008, 2011a). PME induces the organ initiation when applied to the SAM surface as well as EXP (Peaucelle et al., 2008, 2011a). Furthermore, a member of PGX (POLYGALACTURONASE) family regulates the organ initiation pattern by cleaving pectins (Xiao et al., 2014). These findings highlight the significance of the dynamic nature of cell wall extensibility during organ development.
The importance of the cell wall loosening has also been characterized in the stem cell regulation in moss. During stem cell induction triggered by leaf excision in Physcomitrella, some cell wall loosening enzymes are up-regulated (Sakakibara et al., 2014). Meanwhile, transient expression of EXP enhances the stem cell formation (Sakakibara et al., 2014). While the underlying molecular mechanisms may not be conserved between the SAM of vascular plants and stem cells of mosses, these observations emphasize the importance of cell wall extensibility in cell fate determination.
Cell Wall Loosening Changes Microtubule Orientation and Auxin Pattern in the SAM
Cell wall extensibility is a physical property. Therefore, it has been a key question how such a physical property is decoded as a developmental signal to change cellular behaviors. The physical force generated by cell wall loosening could provide an answer. In a growing tissue, cells are constrained by the cell wall. Therefore, cell wall loosening results in cell swelling and pushing the neighboring cells. Recent studies revealed that such pushing force regulates cellular behaviors by affecting the following two factors: cortical microtubule (CMT) orientation and PIN1 protein localization (Figure 1).
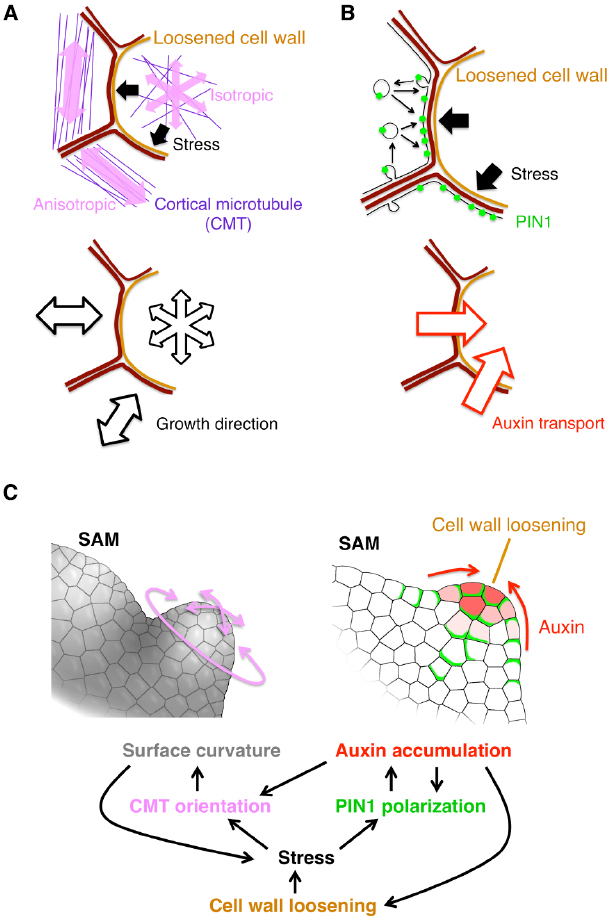
Figure 1. Current model for organ initiation and the role for cell wall loosening. (A,B) The stress from the cell with loosened cell wall leads to reorientation of cortical microtubule (CMT) (A) and PIN1 polarization toward the loosened wall (B) in neighboring cells. (C) Regulatory network among cell wall loosening, CMT and PIN1. Details are mentioned in the text.
The first player, CMT, is a type of cytoskeleton microtubules located under the plasma membrane. In general, the CMT orientation correlates with the stress direction in various plant tissues (Green and King, 1966; Williamson, 1990; Cleary and Hardham, 1993; Wymer et al., 1996; Fischer and Schopfer, 1998). By combining live imaging of CMT, computation of stress properties and laser manipulation techniques, it was shown that, in the SAM, the stress direction determines the CMT orientation (Hamant et al., 2008; Figure 1A). It was also revealed that KATANIN proteins catalyze depolymerization of crossing microtubules to form parallel CMT arrays (Uyttewaal et al., 2012). Since the CMT orientation directs the movement of cellulose synthase complex, cellulose microfibril orients in parallel to the CMT (Paredez et al., 2006). This cellulose microfibril array then restricts the cell expansion to its perpendicular direction (Figure 1A). Thus, cell wall loosening in one cell confers the growth anisotropy on its surrounding cells in response to the initial stress direction, leading to a local outgrowth of the tissue. Moreover, the uneven surface curvature formed by the local outgrowth, and, in turn, renders an anisotropic stress from the inner tissue to the outer tissue, forming a positive feedback circuit that contributes to continuous tissue outgrowth (Hamant et al., 2008; Figure 1C, left).
The second player is the auxin efflux carrier PIN1 as described before in the earlier section. Wall loosening in a cell can cause local stress in the neighboring cells, and it has been proposed that the PIN1 protein is preferentially localized to a part of plasma membrane where the contacting cell wall is the most stressed (Heisler et al., 2010; Figure 1B). A plausible model for this characteristic localization is as follows. PIN1 distribution is dynamically regulated through exocytosis and endocytosis, which increase and decrease the local PIN1 amount in the plasma membrane, respectively. Exocytosis is activated near the highly stressed portion of cell walls where the tension of plasma membrane is also high, thus leading to PIN1 enrichment there (Nakayama et al., 2012; Figure 1B). The resulting PIN1 localization facilitates transport of auxin into the wall-loosening cell (Figures 1B,C, right). Auxin, in turn, stimulates PME and EXP activities to further drive the cell wall loosening (Cleland, 2001; Braybrook and Peaucelle, 2013; Figure 1C, right). Thus, PIN1 mediates this positive feedback to enhance both cell wall loosening and local auxin accumulation (Braybrook and Peaucelle, 2013; Figures 1B,C, right).
Taken together, cell wall loosening triggers organ formation by the following two feedback mechanism (Sampathkumar et al., 2014). One is the feedback between the CMT anisotropy and the SAM geometry (Figures 1A,C left). The other is between PIN1-dependent auxin transport and auxin-induced wall loosening (Figures 1B,C right). Because the auxin accumulation increases the CMT isotropy (Sassi et al., 2014), these two feedback circuits are interconnected (Figure 1C). Therefore, any of auxin accumulation, cell wall loosening and CMT anisotropy change can initiate organ primordium formation. It would be interesting to investigate which of them is the earliest event in nature.
In addition, it was recently reported that a membrane-bound kinase, D6PK (D6 PROTEIN KINASE), phosphorylates PIN1 to control its localization and, vice versa, auxin promotes the polar distribution of D6PK at the plasma membrane, suggesting another feedback mechanism for the auxin-PIN1 mutual regulation (Zourelidou et al., 2009; Willige et al., 2013; Barbosa et al., 2014; Figure 1C). It would be interesting to investigate whether and/or how this D6PK-dependent mechanism and the above-mentioned stress-mediated mechanisms are integrated.
Remaining Questions on Relationships between Cell Wall Loosening and Organ Development
Whereas roles for cell wall loosening proteins in organ formation are evident and the underlying mechanisms have begun to unveil, questions and problems to be challenged still remain. Firstly, methods to directly measure physical stress in the SAM and initiating organs need to be further developed, as our current knowledge on the stress has been all indirectly estimated from measurements of other physical properties: the tissue geometry by scanning electron microscopy (Hamant et al., 2008), the elasticity by atomic force microscopy (Peaucelle et al., 2011a) and the cellular volume expansion caused by manipulation of osmotic pressure (Kierzkowski et al., 2012). Therefore, direct measurements of the stress are ultimately required for comprehensive understanding. Developing new applications of micro-manipulation tools (e.g., microfluidic device, optical tweezers and magnetic nanoparticles) might help to overcome this issue.
Secondly, little is known about mechanisms by which cells directly perceive the physical stress in the SAM. It has been reported that, in other parts such as roots and pollen tubes, loss of function of some receptor-like proteins (THESEUS1, FERONIA, ANXUR1/2, and Receptor-like protein 44) and putative mechanosensitive channels (MCA1/2) alters cellular responses to physical stress or changes in cell wall polysaccharide composition (Hématy et al., 2007; Nakagawa et al., 2007; Boisson-Dernier et al., 2013; Shih et al., 2014; Wolf et al., 2014). Though these proteins could be candidates for sensors to monitor physical stress or cell wall conditions (Cheung and Wu, 2011; Höfte, 2015), direct evidence is still lacking. It also remains to examine whether these proteins contribute to the shoot apex regulation.
Thirdly, though the pin1 mutation and the treatment of auxin transport inhibitors impair leaf morphogenesis and phyllotaxis (Kawamura et al., 2010; Guenot et al., 2012), they do not block the leaf initiation itself. Therefore, the PIN1-independent organ initiation machinery must exist. Some studies implicated that this machinery is driven largely by local auxin biosynthesis by YUCCA proteins (Cheng et al., 2007), auxin signal transduction by ARF5 (Schuetz et al., 2008), and dynamics of CMT array regulated by auxin through ABP1 and KATANIN (Sassi et al., 2014). These suggest that there should be a PIN1-independent but auxin-dependent process for the organ initiation. Activities of auxin influx carriers, AUX1/LAX, might be able to organize auxin distribution to some extent even without PIN1 proteins. Alternatively, as discussed above, any change in cell wall integrity, SAM surface geometry or physical stress might trigger the initiation of organ formation. Further analysis of the pin1 mutant is required to understand these processes.
Lastly, compared with the SAM regulation, there is only limited information as to whether and/or how cell wall loosening is regulated in growth and morphogenesis of other tissues. Some studies have shown that EXP induces the leaf lobe formation and increases the total leaf size (Pien et al., 2001; Sloan et al., 2009; Goh et al., 2012) and also that PME promotes stem elongation (Peaucelle et al., 2011b). However, the involvement of auxin and CMT is unclear in these processes. To further understand the developmental roles for the cell wall loosening, it is important to characterize similarities and differences of molecular mechanisms in different developmental events.
Concluding Remarks
In this review, we outlined current knowledge on the regulation of shoot apex development, especially highlighting signaling molecules, physicochemical properties of cell walls and their modifiers in the extracellular space. A great variety of their actions and interactions demonstrate the importance of the cell wall space as a field for intercellular communication more than just a wall that separates individual cells. This viewpoint is indispensable to understand how plants have evolved their own multicellular body whose cells are interconnected by cell walls in sharp contrast to the animal body. Further technical developments in imaging, measurement, manipulation and mathematical modeling will provide more insights into the dynamics and functions of the cell wall space.
Conflict of Interest Statement
The authors declare that the research was conducted in the absence of any commercial or financial relationships that could be construed as a potential conflict of interest.
Acknowledgments
This paper was supported by MEXT/JSPS KAKENHI Grant Number 14J08452 to YH, 26291057 and 26113507 to KT, and also 24570050, 25114511 and 26113707 to NU. NU was also supported by the Toyoaki Foundation. YH is a JSPS research fellow. KT is an investigator of the Howard Hughes Medical Institute and Gordon and Betty Moore Foundation.
References
Abrash, E. B., and Bergmann, D. C. (2010). Regional specification of stomatal production by the putative ligand CHALLAH. Development 137, 447–455. doi: 10.1242/dev.040931
Abrash, E. B., Davies, K. A., and Bergmann, D. C. (2011). Generation of signaling specificity in Arabidopsis by spatially restricted buffering of ligand-receptor interactions. Plant Cell 23, 2864–2879. doi: 10.1105/tpc.111.086637
Amano, Y., Tsubouchi, H., Shinohara, H., Ogawa, M., and Matsubayashi, Y. (2007). Tyrosine-sulfated glycopeptide involved in cellular proliferation and expansion in Arabidopsis. Proc. Natl. Acad. Sci. U.S.A. 104, 18333–18338. doi: 10.1073/pnas.0706403104
Bainbridge, K., Guyomarc’h, S., Bayer, E., Swarup, R., Bennett, M., Mandel, T., et al. (2008). Auxin influx carriers stabilize phyllotactic patterning. Genes Dev. 22, 810–823. doi: 10.1101/gad.462608
Barbosa, I. C., Zourelidou, M., Willige, B. C., Weller, B., and Schwechheimer, C. (2014). D6 PROTEIN KINASE activates auxin transport-dependent growth and PIN-FORMED phosphorylation at the plasma membrane. Dev. Cell 29, 674–685. doi: 10.1016/j.devcel.2014.05.006
Barkoulas, M., Hay, A., Kougioumoutzi, E., and Tsiantis, M. (2008). A developmental framework for dissected leaf formation in the Arabidopsis relative Cardamine hirsuta. Nat. Genet. 40, 1136–1141. doi: 10.1038/ng.189
Bartrina, I., Otto, E., Strnad, M., Werner, T., and Schmülling, T. (2011). Cytokinin regulates the activity of reproductive meristems, flower organ size, ovule formation, and thus seed yield in Arabidopsis thaliana. Plant Cell 23, 69–80. doi: 10.1105/tpc.110.079079
Bayer, E. M., Smith, R. S., Mandel, T., Nakayama, N., Sauer, M., Prusinkiewicz, P., et al. (2009). Integration of transport-based models for phyllotaxis and midvein formation. Genes Dev. 23, 373–384. doi: 10.1101/gad.497009
Benková, E., Michniewicz, M., Sauer, M., Teichmann, T., Seifertová, D., Jürgens, G., et al. (2003). Local, efflux-dependent auxin gradients as a common module for plant organ formation. Cell 115, 591–602. doi: 10.1016/S0092-8674(03)00924-3
Berger, D., and Altmann, T. (2000). A subtilisin-like serine protease involved in the regulation of stomatal density and distribution in Arabidopsis thaliana. Genes Dev. 14, 1119–1131. doi: 10.1101/gad.14.9.1119
Besnard, F., Refahi, Y., Morin, V., Marteaux, B., Brunoud, G., Chambrier, P., et al. (2014). Cytokinin signalling inhibitory fields provide robustness to phyllotaxis. Nature 505, 417–421. doi: 10.1038/nature12791
Bishopp, A., Help, H., El-Showk, S., Weijers, D., Scheres, B., Friml, J., et al. (2011). A mutually inhibitory interaction between auxin and cytokinin specifies vascular pattern in roots. Curr. Biol. 21, 917–926. doi: 10.1016/j.cub.2011.04.017
Bleckmann, A., Weidtkamp-Peters, S., Seidel, C. A., and Simon, R. (2010). Stem cell signaling in Arabidopsis requires CRN to localize CLV2 to the plasma membrane. Plant Physiol. 152, 166–176. doi: 10.1104/pp.109.149930
Boisson-Dernier, A., Lituiev, D. S., Nestorova, A., Franck, C. M., Thirugnanarajah, S., and Grossniklaus, U. (2013). ANXUR receptor-like kinases coordinate cell wall integrity with growth at the pollen tube tip via NADPH oxidases. PLoS Biol. 11:e1001719. doi: 10.1371/journal.pbio.1001719
Boutté, Y., Ikeda, Y., and Grebe, M. (2007). Mechanisms of auxin-dependent cell and tissue polarity. Curr. Opin. Plant Biol. 10, 616–623. doi: 10.1016/j.pbi.2007.07.008
Bowman, J. L., and Eshed, Y. (2000). Formation and maintenance of the shoot apical meristem. Trends Plant Sci. 5, 110–115. doi: 10.1016/S1360-1385(00)01569-7
Brand, U., Fletcher, J. C., Hobe, M., Meyerowitz, E. M., and Simon, R. (2000). Dependence of stem cell fate in Arabidopsis on a feedback loop regulated by CLV3 activity. Science 289, 617–619. doi: 10.1126/science.289.5479.617
Braybrook, S. A., and Peaucelle, A. (2013). Mechano-chemical aspects of organ formation in Arabidopsis thaliana: the relationship between auxin and pectin. PLoS ONE 8:e57813. doi: 10.1371/journal.pone.0057813
Bryan, W. H., and Newcomb, K. H. (1954). Stimulation of pectin methylesterase activity of cultured tobacco pith by indoleacetic acid. Physiol. Plant. 7, 290–297. doi: 10.1111/j.1399-3054.1954.tb07578.x
Bürkle, L., Cedzich, A., Döpke, C., Stransky, H., Okumoto, S., Gillissen, B., et al. (2003). Transport of cytokinins mediated by purine transporters of the PUP family expressed in phloem, hydathodes, and pollen of Arabidopsis. Plant J. 34, 13–26. doi: 10.1046/j.1365-313X.2003.01700.x
Cedzich, A., Stransky, H., Schulz, B., and Frommer, W. B. (2008). Characterization of cytokinin and adenine transport in Arabidopsis cell cultures. Plant Physiol. 148, 1857–1867. doi: 10.1104/pp.108.128454
Chandler, J. W. (2009). Local auxin production: a small contribution to a big field. Bioessays 31, 60–70. doi: 10.1002/bies.080146
Chen, M. K., Wilson, R. L., Palme, K., Ditengou, F. A., and Shpak, E. D. (2013). ERECTA family genes regulate auxin transport in the shoot apical meristem and forming leaf primordia. Plant Physiol. 162, 1978–1991. doi: 10.1104/pp.113.218198
Cheng, Y., Dai, X., and Zhao, Y. (2007). Auxin synthesized by the YUCCA flavin monooxygenases is essential for embryogenesis and leaf formation in Arabidopsis. Plant Cell 19, 2430–2439. doi: 10.1105/tpc.107.053009
Cheung, A. Y., and Wu, H. M. (2011). THESEUS 1, FERONIA and relatives: a family of cell wall-sensing receptor kinases? Curr. Opin. Plant Biol. 14, 632–641. doi: 10.1016/j.pbi.2011.09.001
Chickarmane, V. S., Gordon, S. P., Tarr, P. T., Heisler, M. G., and Meyerowitz, E. M. (2012). Cytokinin signaling as a positional cue for patterning the apical-basal axis of the growing Arabidopsis shoot meristem. Proc. Natl. Acad. Sci. U.S.A. 109, 4002–4007. doi: 10.1073/pnas.1200636109
Cleary, A. L., and Hardham, A. R. (1993). Pressure induced reorientation of cortical microtubules in epidermal cells of lolium rigidum leaves. Plant Cell Physiol. 34, 1003–1008.
Cleland, R. (1958). A separation of auxin-induced cell wall loosening into its plastie and elastic components. Physiol. Plant. 11, 599–609. doi: 10.1111/j.1399-3054.1958.tb08255.x
Cleland, R. E. (2001). Unlocking the mysteries of leaf primordia formation. Proc. Natl. Acad. Sci. U.S.A. 98, 10981–10982. doi: 10.1073/pnas.211443498
Cock, J. M., and McCormick, S. (2001). A large family of genes that share homology with CLAVATA3. Plant Physiol. 126, 939–942. doi: 10.1104/pp.126.3.939
Davies, P. J. (1995). “The plant hormones: their nature, occurrence and functions,” in Plant Hormones Physiology, Biochemistry and Molecular Biology, ed. P. J. Davies (Dordrecht: Kluwer Academic Publishers), 1–12.
De Rybel, B., Adibi, M., Breda, A. S., Wendrich, J. R., Smit, M. E., Novák, O., et al. (2014). Plant development. Integration of growth and patterning during vascular tissue formation in Arabidopsis. Science 345, 1255215. doi: 10.1126/science.1255215
DeYoung, B. J., Bickle, K. L., Schrage, K. J., Muskett, P., Patel, K., and Clark, S. E. (2006). The CLAVATA1-related BAM1, BAM2, and BAM3 receptor kinase-like proteins are required for meristem function in Arabidopsis. Plant J. 45, 1–16. doi: 10.1111/j.1365-313X.2005.02592.x
DeYoung, B. J., and Clark, S. E. (2008). BAM receptors regulate stem cell specification and organ development through complex interactions with CLAVATA signaling. Genetics 180, 895–904. doi: 10.1534/genetics.108.091108
Efroni, I., Han, S. K., Kim, H. J., Wu, M. F., Steiner, E., Birnbaum, K. D., et al. (2013). Regulation of leaf maturation by chromatin-mediated modulation of cytokinin responses. Dev. Cell 24, 438–445. doi: 10.1016/j.devcel.2013.01.019
Engineer, C. B., Ghassemian, M., Anderson, J. C., Peck, S. C., Hu, H., and Schroeder, J. I. (2014). Carbonic anhydrases, EPF2 and a novel protease mediate CO2 control of stomatal development. Nature 513, 246–250. doi: 10.1038/nature13452
Fischer, K., and Schopfer, P. (1998). Physical strain-mediated microtubule reorientation in the epidermis of gravitropically or phototropically stimulated maize coleoptiles. Plant J. 15, 119–123. doi: 10.1046/j.1365-313X.1998.00173.x
Fleming, A., McQueen-Mason, S., Mandel, T., and Kuhlemeier, C. (1997). Induction of leaf primordia by the cell wall protein expansin. Science 276, 1415–1418. doi: 10.1126/science.276.5317.1415
Fletcher, J. C., Brand, U., Running, M. P., Simon, R., and Meyerowitz, E. M. (1999). Signaling of cell fate decisions by CLAVATA3 in Arabidopsis shoot meristems. Science 283, 1911–1914. doi: 10.1126/science.283.5409.1911
Furutani, M., Vernoux, T., Traas, J., Kato, T., Tasaka, M., and Aida, M. (2004). PIN-FORMED1 and PINOID regulate boundary formation and cotyledon development in Arabidopsis embryogenesis. Development 131, 5021–5030. doi: 10.1242/dev.01388
Gajdošová, S., Spíchal, L., Kamínek, M., Hoyerová, K., Novák, O., Dobrev, P. I., et al. (2011). Distribution, biological activities, metabolism, and the conceivable function of cis-zeatin-type cytokinins in plants. J. Exp. Bot. 62, 2827–2840. doi: 10.1093/jxb/erq457
Giulini, A., Wang, J., and Jackson, D. (2004). Control of phyllotaxy by the cytokinin-inducible response regulator homologue ABPHYL1. Nature 430, 1031–1034. doi: 10.1038/nature02778
Goh, H. H., Sloan, J., Dorca-Fornell, C., and Fleming, A. (2012). Inducible repression of multiple expansin genes leads to growth suppression during leaf development. Plant Physiol. 159, 1759–1770. doi: 10.1104/pp.112.200881
Gordon, S. P., Chickarmane, V. S., Ohno, C., and Meyerowitz, E. M. (2009). Multiple feedback loops through cytokinin signaling control stem cell number within the Arabidopsis shoot meristem. Proc. Natl. Acad. Sci. U.S.A. 106, 16529–16534. doi: 10.1073/pnas.0908122106
Green, P. B., and King, A. (1966). A mechanism for the origin of specifically oriented textures in development with special reference to Nitella wall texture. Aust. J. Biol. Sci. 19, 421–438.
Greenboim-Wainberg, Y., Maymon, I., Borochov, R., Alvarez, J., Olszewski, N., Ori, N., et al. (2005). Cross talk between gibberellin and cytokinin: the Arabidopsis GA response inhibitor SPINDLY plays a positive role in cytokinin signaling. Plant Cell 17, 92–102. doi: 10.1105/tpc.104.028472
Grones, P., and Friml, J. (2015). Auxin transporters and binding proteins at a glance. J. Cell. Sci. 128, 1–7. doi: 10.1242/jcs.159418
Guenot, B., Bayer, E., Kierzkowski, D., Smith, R. S., Mandel, T., Žádníková, P., et al. (2012). PIN1-independent leaf initiation in Arabidopsis. Plant Physiol. 159, 1501–1510. doi: 10.1104/pp.112.200402
Guilfoyle, T. J., and Hagen, G. (2007). Auxin response factors. Curr. Opin. Plant Biol. 10, 453–460. doi: 10.1016/j.pbi.2007.08.014
Guo, Y., Han, L., Hymes, M., Denver, R., and Clark, S. E. (2010). CLAVATA2 forms a distinct CLE-binding receptor complex regulating Arabidopsis stem cell specification. Plant J. 63, 889–900. doi: 10.1111/j.1365-313X.2010.04295.x
Hamant, O., Heisler, M. G., Jönsson, H., Krupinski, P., Uyttewaal, M., Bokov, P., et al. (2008). Developmental patterning by mechanical signals in Arabidopsis. Science 322, 1650–1655. doi: 10.1126/science.1165594
Hara, K., Kajita, R., Torii, K. U., Bergmann, D. C., and Kakimoto, T. (2007). The secretory peptide gene EPF1 enforces the stomatal one-cell-spacing rule. Genes Dev. 21, 1720–1725. doi: 10.1101/gad.1550707
Hara, K., Yokoo, T., Kajita, R., Onishi, T., Yahata, S., Peterson, K. M., et al. (2009). Epidermal cell density is autoregulated via a secretory peptide, EPIDERMAL PATTERNING FACTOR 2 in Arabidopsis leaves. Plant Cell Physiol. 50, 1019–1031. doi: 10.1093/pcp/pcp068
Hay, A., Barkoulas, M., and Tsiantis, M. (2006). ASYMMETRIC LEAVES1 and auxin activities converge to repress BREVIPEDICELLUS expression and promote leaf development in Arabidopsis. Development 133, 3955–3961. doi: 10.1242/dev.02545
Heisler, M. G., Hamant, O., Krupinski, P., Uyttewaal, M., Ohno, C., Jönsson, H., et al. (2010). Alignment between PIN1 polarity and microtubule orientation in the shoot apical meristem reveals a tight coupling between morphogenesis and auxin transport. PLoS Biol. 8:e1000516. doi: 10.1371/journal.pbio.1000516
Heisler, M. G., Ohno, C., Das, P., Sieber, P., Reddy, G. V., Long, J. A., et al. (2005). Patterns of auxin transport and gene expression during primordium development revealed by live imaging of the Arabidopsis inflorescence meristem. Curr. Biol. 15, 1899–1911. doi: 10.1016/j.cub.2005.09.052
Hématy, K., Sado, P. E., Van Tuinen, A., Rochange, S., Desnos, T., Balzergue, S., et al. (2007). A receptor-like kinase mediates the response of Arabidopsis cells to the inhibition of cellulose synthesis. Curr. Biol. 17, 922–931. doi: 10.1016/j.cub.2007.05.018
Hirose, N., Makita, N., Yamaya, T., and Sakakibara, H. (2005). Functional characterization and expression analysis of a gene, OsENT2, encoding an equilibrative nucleoside transporter in rice suggest a function in cytokinin transport. Plant Physiol. 138, 196–206. doi: 10.1104/pp.105.060137
Höfte, H. (2015). The yin and yang of cell wall integrity control: brassinosteroid and FERONIA signaling. Plant Cell Physiol. 56, 224–231. doi: 10.1093/pcp/pcu182
Hohm, T., Demarsy, E., Quan, C., Allenbach Petrolati, L., Preuten, T., Vernoux, T., et al. (2014). Plasma membrane H+ -ATPase regulation is required for auxin gradient formation preceding phototropic growth. Mol. Syst. Biol. 10, 751. doi: 10.15252/msb.20145247
Hunt, L., Bailey, K. J., and Gray, J. E. (2010). The signalling peptide EPFL9 is a positive regulator of stomatal development. New Phytol. 186, 609–614. doi: 10.1111/j.1469-8137.2010.03200.x
Hunt, L., and Gray, J. E. (2009). The signaling peptide EPF2 controls asymmetric cell divisions during stomatal development. Curr. Biol. 19, 864–869. doi: 10.1016/j.cub.2009.03.069
Jean, R. V. (1994). Phyllotaxis: A Systemic Study in Plant Morphogenesis. New York: Cambridge University Press. doi: 10.1017/CBO9780511666933
Jönsson, H., Heisler, M. G., Shapiro, B. E., Meyerowitz, E. M., and Mjolsness, E. (2006). An auxin-driven polarized transport model for phyllotaxis. Proc. Natl. Acad. Sci. U.S.A. 103, 1633–1638. doi: 10.1073/pnas.0509839103
Kawamura, E., Horiguchi, G., and Tsukaya, H. (2010). Mechanisms of leaf tooth formation in Arabidopsis. Plant J. 62, 429–441. doi: 10.1111/j.1365-313X.2010.04156.x
Kieber, J. J., and Schaller, G. E. (2014). Cytokinins. Arabidopsis Book 12:e0168. doi: 10.1199/tab.0168
Kierzkowski, D., Nakayama, N., Routier-Kierzkowska, A. L., Weber, A., Bayer, E., Schorderet, M., et al. (2012). Elastic domains regulate growth and organogenesis in the plant shoot apical meristem. Science 335, 1096–1099. doi: 10.1126/science.1213100
Kinoshita, A., Betsuyaku, S., Osakabe, Y., Mizuno, S., Nagawa, S., Stahl, Y., et al. (2010). RPK2 is an essential receptor-like kinase that transmits the CLV3 signal in Arabidopsis. Development 137, 3911–3920. doi: 10.1242/dev.048199
Ko, D., Kang, J., Kiba, T., Park, J., Kojima, M., Do, J., et al. (2014). Arabidopsis ABCG14 is essential for the root-to-shoot translocation of cytokinin. Proc. Natl. Acad. Sci. U.S.A. 111, 7150–7155. doi: 10.1073/pnas.1321519111
Köllmer, I., Novák, O., Strnad, M., Schmülling, T., and Werner, T. (2014). Overexpression of the cytosolic cytokinin oxidase/dehydrogenase (CKX7) from Arabidopsis causes specific changes in root growth and xylem differentiation. Plant J. 78, 359–371. doi: 10.1111/tpj.12477
Komori, R., Amano, Y., Ogawa-Ohnishi, M., and Matsubayashi, Y. (2009). Identification of tyrosylprotein sulfotransferase in Arabidopsis. Proc. Natl. Acad. Sci. U.S.A. 106, 15067–15072. doi: 10.1073/pnas.0902801106
Kondo, T., Kajita, R., Miyazaki, A., Hokoyama, M., Nakamura-Miura, T., Mizuno, S., et al. (2010). Stomatal density is controlled by a mesophyll-derived signaling molecule. Plant Cell Physiol. 51, 1–8. doi: 10.1093/pcp/pcp180
Kondo, Y., Hirakawa, Y., and Fukuda H. (2014). “Chapter four—peptide ligands in plants,” in The Enzymes Vol. 35—Signaling Pathways in Plants, eds Y. Machida, C. Lin, and F. Tamanoi (Waltham: Academic Press), 85–112.
Kudo, T., Kiba, T., and Sakakibara, H. (2010). Metabolism and long-distance translocation of cytokinins. J. Integr. Plant Biol. 52, 53–60. doi: 10.1111/j.1744-7909.2010.00898.x
Kudoyarova, G. R., Korobova, A. V., Akhiyarova, G. R., Arkhipova, T. N., Zaytsev, D. Y., Prinsen, E., et al. (2014). Accumulation of cytokinins in roots and their export to the shoots of durum wheat plants treated with the protonophore carbonyl cyanide m-chlorophenylhydrazone (CCCP). J. Exp. Bot. 65, 2287–2294. doi: 10.1093/jxb/eru113
Laloue, M., Pethe-Terrine, C., and Guern, J. (1981). “Uptake and metabolism of CKs in tobacco cells: studies in relation to the expression of their biological activities,” in Metabolism and Molecular Activities of CKs, eds J. Guern and C. Peaud-Lenoel (Berlin: Springer), 80–96.
Lee, B. H., Johnston, R., Yang, Y., Gallavotti, A., Kojima, M., Travençolo, B. A., et al. (2009). Studies of aberrant phyllotaxy1 mutants of maize indicate complex interactions between auxin and cytokinin signaling in the shoot apical meristem. Plant Physiol. 150, 205–216. doi: 10.1104/pp.109.137034
Leibfried, A., To, J. P., Busch, W., Stehling, S., Kehle, A., Demar, M., et al. (2005). WUSCHEL controls meristem function by direct regulation of cytokinin-inducible response regulators. Nature 438, 1172–1175. doi: 10.1038/nature04270
Li, G., Liu, K., Baldwin, S. A., and Wang, D. (2003). Equilibrative nucleoside transporters of Arabidopsis thaliana. cDNA cloning, expression pattern, and analysis of transport activities. J. Biol. Chem. 278, 35732–35742. doi: 10.1074/jbc.M304768200
Löbler, M., and Klämbt, D. (1985). Auxin-binding protein from coleoptile membranes of corn (Zea mays L.). I. Purification by immunological methods and characterization. J. Biol. Chem. 260, 9848–9853.
Luschnig, C., and Vert, G. (2014). The dynamics of plant plasma membrane proteins: PINs and beyond. Development 141, 2924–2938. doi: 10.1242/dev.103424
Marhavý, P., Duclercq, J., Weller, B., Feraru, E., Bielach, A., Offringa, R., et al. (2014). Cytokinin controls polarity of PIN1-dependent auxin transport during lateral root organogenesis. Curr. Biol. 24, 1031–1037. doi: 10.1016/j.cub.2014.04.002
Matsubayashi, Y. (2014). Posttranslationally modified small-peptide signals in plants. Annu. Rev. Plant Biol. 65, 385–413. doi: 10.1146/annurev-arplant-050312-120122
Matsubayashi, Y., Ogawa, M., Kihara, H., Niwa, M., and Sakagami, Y. (2006). Disruption and overexpression of Arabidopsis phytosulfokine receptor gene affects cellular longevity and potential for growth. Plant Physiol. 142, 45–53. doi: 10.1104/pp.106.081109
Matsubayashi, Y., Ogawa, M., Morita, A., and Sakagami, Y. (2002). An LRR receptor kinase involved in perception of a peptide plant hormone, phytosulfokine. Science 296, 1470–1472. doi: 10.1126/science.1069607
Matsubayashi, Y., and Sakagami, Y. (1996). Phytosulfokine, sulfated peptides that induce the proliferation of single mesophyll cells of Asparagus officinalis L. Proc. Natl. Acad. Sci. U.S.A. 93, 7623–7627.
Mayer, K. F., Schoof, H., Haecker, A., Lenhard, M., Jürgens, G., and Laux, T. (1998). Role of WUSCHEL in regulating stem cell fate in the Arabidopsis shoot meristem. Cell 95, 805–815. doi: 10.1016/S0092-8674(00)81703-1
McQueen-Mason, S., and Cosgrove, D. J. (1994). Disruption of hydrogen bonding between plant cell wall polymers by proteins that induce wall extension. Proc. Natl. Acad. Sci. U.S.A. 91, 6574–6578.
Miwa, H., Betsuyaku, S., Iwamoto, K., Kinoshita, A., Fukuda, H., and Sawa, S. (2008). The receptor-like kinase SOL2 mediates CLE signaling in Arabidopsis. Plant Cell Physiol. 49, 1752–1757. doi: 10.1093/pcp/pcn148
Motyka, V., Vaňková, R., Čapková, V., Petrášek, J., Kamínek, M., and Schmülling, T. (2003). Cytokinin-induced upregulation of cytokinin oxidase activity in tobacco includes changes in enzyme glycosylation and secretion. Physiol. Plant. 117, 11–21. doi: 10.1034/j.1399-3054.2003.1170102.x
Müller, R., Bleckmann, A., and Simon, R. (2008). The receptor kinase CORYNE of Arabidopsis transmits the stem cell-limiting signal CLAVATA3 independently of CLAVATA1. Plant Cell 20, 934–946. doi: 10.1105/tpc.107.057547
Murray, J. A., Jones, A., Godin, C., and Traas, J. (2012). Systems analysis of shoot apical meristem growth and development: integrating hormonal and mechanical signaling. Plant Cell 24, 3907–3919. doi: 10.1105/tpc.112.102194
Nagawa, S., Xu, T., Lin, D., Dhonukshe, P., Zhang, X., Friml, J., et al. (2012). ROP GTPase-dependent actin microfilaments promote PIN1 polarization by localized inhibition of clathrin-dependent endocytosis. PLoS Biol. 10:e1001299. doi: 10.1371/journal.pbio.1001299
Nakagawa, Y., Katagiri, T., Shinozaki, K., Qi, Z., Tatsumi, H., Furuichi, T., et al. (2007). Arabidopsis plasma membrane protein crucial for Ca2+ influx and touch sensing in roots. Proc. Natl. Acad. Sci. U.S.A. 104, 3639–3644. doi: 10.1073/pnas.0607703104
Nakamura, M., Kiefer, C. S., and Grebe, M. (2012). Planar polarity, tissue polarity and planar morphogenesis in plants. Curr. Opin. Plant Biol. 15, 593–600. doi: 10.1016/j.pbi.2012.07.006
Nakayama, N., Smith, R. S., Mandel, T., Robinson, S., Kimura, S., Boudaoud, A., et al. (2012). Mechanical regulation of auxin-mediated growth. Curr. Biol. 22, 1468–1476. doi: 10.1016/j.cub.2012.06.050
Narváez-Vásquez, J., and Ryan, C. A. (2004). The cellular localization of prosystemin: a functional role for phloem parenchyma in systemic wound signaling. Planta 218, 360–369. doi: 10.1007/s00425-003-1115-3
O’Connor, D. L., Runions, A., Sluis, A., Bragg, J., Vogel, J. P., Prusinkiewicz, P., et al. (2014). A division in PIN-mediated auxin patterning during organ initiation in grasses. PLoS Comput. Biol. 10:e1003447. doi: 10.1371/journal.pcbi.1003447
Ogawa-Ohnishi, M., Matsushita, W., and Matsubayashi, Y. (2013). Identification of three hydroxyproline O-arabinosyltransferases in Arabidopsis thaliana. Nat. Chem. Biol. 9, 726–730. doi: 10.1038/nchembio.1351
Ogawa, M., Shinohara, H., Sakagami, Y., and Matsubayashi, Y. (2008). Arabidopsis CLV3 peptide directly binds CLV1 ectodomain. Science 319, 294. doi: 10.1126/science.1150083
Ohki, S., Takeuchi, M., and Mori, M. (2011). The NMR structure of stomagen reveals the basis of stomatal density regulation by plant peptide hormones. Nat. Commun. 2, 512. doi: 10.1038/ncomms1520
Ohyama, K., Shinohara, H., Ogawa-Ohnishi, M., and Matsubayashi, Y. (2009). A glycopeptide regulating stem cell fate in Arabidopsis thaliana. Nat. Chem. Biol. 5, 578–580. doi: 10.1038/nchembio.182
Okada, K., Ueda, J., Komaki, M. K., Bell, C. J., and Shimura, Y. (1991). Requirement of the auxin polar transport system in early stages of Arabidopsis floral bud formation. Plant Cell 3, 677–684. doi: 10.1105/tpc.3.7.677
Paredez, A. R., Somerville, C. R., and Ehrhardt, D. W. (2006). Visualization of cellulose synthase demonstrates functional association with microtubules. Science 312, 1491–1495. doi: 10.1126/science.1126551
Pearce, G., Strydom, D., Johnson, S., and Ryan, C. A. (1991). A polypeptide from tomato leaves induces wound-inducible proteinase inhibitor proteins. Science 253, 895–897. doi: 10.1126/science.253.5022.895
Peaucelle, A., Braybrook, S. A., Le Guillou, L., Bron, E., Kuhlemeier, C., and Höfte, H. (2011a). Pectin-induced changes in cell wall mechanics underlie organ initiation in Arabidopsis. Curr. Biol. 21, 1720–1726. doi: 10.1016/j.cub.2011.08.057
Peaucelle, A., Louvet, R., Johansen, J. N., Salsac, F., Morin, H., Fournet, F., et al. (2011b). The transcription factor BELLRINGER modulates phyllotaxis by regulating the expression of a pectin methylesterase in Arabidopsis. Development 138, 4733–4741. doi: 10.1242/dev.072496
Peaucelle, A., Louvet, R., Johansen, J. N., Höfte, H., Laufs, P., Pelloux, J., et al. (2008). Arabidopsis phyllotaxis is controlled by the methyl-esterification status of cell-wall pectins. Curr. Biol. 18, 1943–1948. doi: 10.1016/j.cub.2008.10.065
Pien, S., Wyrzykowska, J., McQueen-Mason, S., Smart, C., and Fleming, A. (2001). Local expression of expansin induces the entire process of leaf development and modifies leaf shape. Proc. Natl. Acad. Sci. U.S.A. 98, 11812–11817. doi: 10.1073/pnas.191380498
Qi, Z., and Xiong, L. (2013). Characterization of a purine permease family gene OsPUP7 involved in growth and development control in rice. J. Integr. Plant Biol. 55, 1119–1135. doi: 10.1111/jipb.12101
Reinhardt, D., Mandel, T., and Kuhlemeier, C. (2000). Auxin regulates the initiation and radial position of plant lateral organs. Plant Cell 12, 507–518. doi: 10.1105/tpc.12.4.507
Reinhardt, D., Pesce, E. R., Stieger, P., Mandel, T., Baltensperger, K., Bennett, M., et al. (2003). Regulation of phyllotaxis by polar auxin transport. Nature 426, 255–260. doi: 10.1038/nature02081
Robert, S., Kleine-Vehn, J., Barbez, E., Sauer, M., Paciorek, T., Baster, P., et al. (2010). ABP1 mediates auxin inhibition of clathrin-dependent endocytosis in Arabidopsis. Cell 143, 111–121. doi: 10.1016/j.cell.2010.09.027
Sakakibara, K., Reisewitz, P., Aoyama, T., Friedrich, T., Ando, S., Sato, Y., et al. (2014). WOX13-like genes are required for reprogramming of leaf and protoplast cells into stem cells in the moss Physcomitrella patens. Development 141, 1660–1670. doi: 10.1242/dev.097444
Salehin, M., Bagchi, R., and Estelle, M. (2015). SCFTIR1/AFB-based auxin perception: mechanism and role in plant growth and development. Plant Cell 27, 9–19. doi: 10.1105/tpc.114.133744
Sampathkumar, A., Yan, A., Krupinski, P., and Meyerowitz, E. M. (2014). Physical forces regulate plant development and morphogenesis. Curr. Biol. 24, R475–R483. doi: 10.1016/j.cub.2014.03.014
Sassi, M., Ali, O., Boudon, F., Cloarec, G., Abad, U., Cellier, C., et al. (2014). An auxin-mediated shift toward growth isotropy promotes organ formation at the shoot meristem in Arabidopsis. Curr. Biol. 24, 2335–2342. doi: 10.1016/j.cub.2014.08.036
Scarpella, E., Marcos, D., Friml, J., and Berleth, T. (2006). Control of leaf vascular patterning by polar auxin transport. Genes Dev. 20, 1015–1027. doi: 10.1101/gad.1402406
Schaller, G. E., Bishopp, A., and Kieber, J. J. (2015). The yin-yang of hormones: cytokinin and auxin interactions in plant development. Plant Cell 27, 44–63. doi: 10.1105/tpc.114.133595
Schoof, H., Lenhard, M., Haecker, A., Mayer, K. F., Jürgens, G., and Laux, T. (2000). The stem cell population of Arabidopsis shoot meristems in maintained by a regulatory loop between the CLAVATA and WUSCHEL genes. Cell 100, 635–644. doi: 10.1016/S0092-8674(00)80700-X
Schuetz, M., Berleth, T., and Mattsson, J. (2008). Multiple MONOPTEROS-dependent pathways are involved in leaf initiation. Plant Physiol. 148, 870–880. doi: 10.1104/pp.108.119396
Shani, E., Ben-Gera, H., Shleizer-Burko, S., Burko, Y., Weiss, D., and Ori, N. (2010). Cytokinin regulates compound leaf development in tomato. Plant Cell 22, 3206–3217. doi: 10.1105/tpc.110.078253
Shih, H. W., Miller, N. D., Dai, C., Spalding, E. P., and Monshausen, G. B. (2014). The receptor-like kinase FERONIA is required for mechanical signal transduction in Arabidopsis seedlings. Curr. Biol. 24, 1887–18892. doi: 10.1016/j.cub.2014.06.064
Shinohara, H., Moriyama, Y., Ohyama, K., and Matsubayashi, Y. (2012). Biochemical mapping of a ligand-binding domain within Arabidopsis BAM1 reveals diversified ligand recognition mechanisms of plant LRR-RKs. Plant J. 70, 845–854. doi: 10.1111/j.1365-313X.2012.04934.x
Shpak, E. D., Berthiaume, C. T., Hill, E. J., and Torii, K. U. (2004). Synergistic interaction of three ERECTA-family receptor-like kinases controls Arabidopsis organ growth and flower development by promoting cell proliferation. Development 131, 1491–1501. doi: 10.1242/dev.01028
Sloan, J., Backhaus, A., Malinowski, R., McQueen-Mason, S., and Fleming, A. J. (2009). Phased control of expansin activity during leaf development identifies a sensitivity window for expansin-mediated induction of leaf growth. Plant Physiol. 151, 1844–1854. doi: 10.1104/pp.109.144683
Smith, R. S., Guyomarc’h, S., Mandel, T., Reinhardt, D., Kuhlemeier, C., and Prusinkiewicz, P. (2006). A plausible model of phyllotaxis. Proc. Natl. Acad. Sci. U.S.A. 103, 1301–1306. doi: 10.1073/pnas.0510457103
Srivastava, R., Liu, J. X., and Howell, S. H. (2008). Proteolytic processing of a precursor protein for a growth-promoting peptide by a subtilisin serine protease in Arabidopsis. Plant J. 56, 219–227. doi: 10.1111/j.1365-313X.2008.03598.x
Steeves, T. A., and Sussex, I. M. (1989). Patterns in Plant Development, 2nd Edn. New York: Cambridge University Press. doi: 10.1017/CBO9780511626227
Stoma, S., Lucas, M., Chopard, J., Schaedel, M., Traas, J., and Godin, C. (2008). Flux-based transport enhancement as a plausible unifying mechanism for auxin transport in meristem development. PLoS Comput. Biol. 4:e1000207. doi: 10.1371/journal.pcbi.1000207
Su, Y. H., Liu, Y. B., and Zhang, X. S. (2011). Auxin-cytokinin interaction regulates meristem development. Mol. Plant 4, 616–625. doi: 10.1093/mp/ssr007
Sugano, S. S., Shimada, T., Imai, Y., Okawa, K., Tamai, A., Mori, M., et al. (2010). Stomagen positively regulates stomatal density in Arabidopsis. Nature 463, 241–244. doi: 10.1038/nature08682
Sun, J. Q., Hirose, N., Wang, X. C., Wen, P., Xue, L., Sakakibara, H., et al. (2005). The Arabidopsis SOI33/AtENT8 gene encodes a putative equilibrative nucleoside transporter that is involved in cytokinin transport in Planta. J. Integr. Plant Biol. 47, 588–603. doi: 10.1111/j.1744-7909.2005.00104.x
Tamaki, T., Betsuyaku, S., Fujiwara, M., Fukao, Y., Fukuda, H., and Sawa, S. (2013). SUPPRESSOR OF LLP1 1-mediated C-terminal processing is critical for CLE19 peptide activity. Plant J. 76, 970–981. doi: 10.1111/tpj.12349
Thomas, J., Ross, C. W., Chastain, C. J., Koomanoff, N., and Hendrix, J. E. (1981). Cytokinin-induced wall extensibility in excised cotyledons of radish and cucumber. Plant Physiol. 68, 107–110. doi: 10.1104/pp.68.1.107
Torii, K. U., Mitsukawa, N., Oosumi, T., Matsuura, Y., Yokoyama, R., Whittier, R. F., et al. (1996). The Arabidopsis ERECTA gene encodes a putative receptor protein kinase with extracellular leucine-rich repeats. Plant Cell 8, 735–746. doi: 10.1105/tpc.8.4.735
Tsugeki, R., Ditengou, F. A., Sumi, Y., Teale, W., Palme, K., and Okada, K. (2009). NO VEIN mediates auxin-dependent specification and patterning in the Arabidopsis embryo, shoot, and root. Plant Cell 21, 3133–3151. doi: 10.1105/tpc.109.068841
Uchida, N., Igari, K., Bogenschutz, N. L., Torii, K. U., and Tasaka, M. (2011). Arabidopsis ERECTA-family receptor kinases mediate morphological alterations stimulated by activation of NB-LRR-type UNI proteins. Plant Cell Physiol. 52, 804–814. doi: 10.1093/pcp/pcr032
Uchida, N., Lee, J. S., Horst, R. J., Lai, H. H., Kajita, R., Kakimoto, T., et al. (2012). Regulation of inflorescence architecture by intertissue layer ligand-receptor communication between endodermis and phloem. Proc. Natl. Acad. Sci. U.S.A. 109, 6337–6342. doi: 10.1073/pnas.1117537109
Uchida, N., Shimada, M., and Tasaka, M. (2013). ERECTA-family receptor kinases regulate stem cell homeostasis via buffering its cytokinin responsiveness in the shoot apical meristem. Plant Cell Physiol. 54, 343–351. doi: 10.1093/pcp/pcs109
Uyttewaal, M., Burian, A., Alim, K., Landrein, B., Borowska-Wykret, D., Dedieu, A., et al. (2012). Mechanical stress acts via katanin to amplify differences in growth rate between adjacent cells in Arabidopsis. Cell 149, 439–451. doi: 10.1016/j.cell.2012.02.048
Vieten, A., Sauer, M., Brewer, P. B., and Friml, J. (2007). Molecular and cellular aspects of auxin-transport-mediated development. Trends Plant Sci. 12, 160–168. doi: 10.1016/j.tplants.2007.03.006
von Schwartzenberg, K., Núñez, M. F., Blaschke, H., Dobrev, P. I., Novák, O., Motyka, V., et al. (2007). Cytokinins in the bryophyte Physcomitrella patens: analyses of activity, distribution, and cytokinin oxidase/dehydrogenase overexpression reveal the role of extracellular cytokinins. Plant Physiol. 145, 786–800. doi: 10.1104/pp.107.103176
Wang, T., Park, Y. B., Caporini, M. A., Rosay, M., Zhong, L., Cosgrove, D. J., et al. (2013). Sensitivity-enhanced solid-state NMR detection of expansin’s target in plant cell walls. Proc. Natl. Acad. Sci. U.S.A. 110, 16444–16449. doi: 10.1073/pnas.1316290110
Werner, T., Motyka, V., Laucou, V., Smets, R., Van Onckelen, H., and Schmülling, T. (2003). Cytokinin-deficient transgenic Arabidopsis plants show multiple developmental alterations indicating opposite functions of cytokinins in the regulation of shoot and root meristem activity. Plant Cell 15, 2532–2550. doi: 10.1105/tpc.014928
Williamson, R. E. (1990). Alignment of cortical microtubules by anisotropic wall stresses. Aust. J. Biol. Sci. 17, 601–613. doi: 10.1071/PP9900601
Willige, B. C., Ahlers, S., Zourelidou, M., Barbosa, I. C., Demarsy, E., Trevisan, M., et al. (2013). D6PK AGCVIII kinases are required for auxin transport and phototropic hypocotyl bending in Arabidopsis. Plant Cell 25, 1674–1688. doi: 10.1105/tpc.113.111484
Wolf, S., van der Does, D., Ladwig, F., Sticht, C., Kolbeck, A., Schürholz, A. K., et al. (2014). A receptor-like protein mediates the response to pectin modification by activating brassinosteroid signaling. Proc. Natl. Acad. Sci. U.S.A. 111, 15261–15266. doi: 10.1073/pnas.1322979111
Wymer, C. L., Wymer, S. A., Cosgrove, D. J., and Cyr, R. J. (1996). Plant cell growth responds to external forces and the response requires intact microtubules. Plant Physiol. 110, 425–430. doi: 10.1104/pp.110.2.425
Xiao, C., Somerville, C., and Anderson, C. T. (2014). POLYGALACTURONASE INVOLVED IN EXPANSION1 functions in cell elongation and flower development in Arabidopsis. Plant Cell 26, 1018–1035. doi: 10.1105/tpc.114.123968
Xu, T., Wen, M., Nagawa, S., Fu, Y., Chen, J. G., Wu, M. J., et al. (2010). Cell surface- and rho GTPase-based auxin signaling controls cellular interdigitation in Arabidopsis. Cell 143, 99–110. doi: 10.1016/j.cell.2010.09.003
Yanai, O., Shani, E., Dolezal, K., Tarkowski, P., Sablowski, R., Sandberg, G., et al. (2005). Arabidopsis KNOXI proteins activate cytokinin biosynthesis. Curr. Biol. 15, 1566–1571. doi: 10.1016/j.cub.2005.07.060
Yang, Y., Hammes, U. Z., Taylor, C. G., Schachtman, D. P., and Nielsen, E. (2006). High-affinity auxin transport by the AUX1 influx carrier protein. Curr. Biol. 16, 1123–1127. doi: 10.1016/j.cub.2005.07.060
Yoshida, S., Mandel, T., and Kuhlemeier, C. (2011). Stem cell activation by light guides plant organogenesis. Genes Dev. 25, 1439–1450. doi: 10.1101/gad.631211
Zhao, Y. (2008). The role of local biosynthesis of auxin and cytokinin in plant development. Curr. Opin. Plant Biol. 11, 16–22. doi: 10.1016/j.pbi.2007.10.008
Zhao, Z., Andersen, S. U., Ljung, K., Dolezal, K., Miotk, A., Schultheiss, S. J., et al. (2010). Hormonal control of the shoot stem-cell niche. Nature 465, 1089–1092. doi: 10.1038/nature09126
Zhu, Y., Wang, Y., Li, R., Song, X., Wang, Q., Huang, S., et al. (2010). Analysis of interactions among the CLAVATA3 receptors reveals a direct interaction between CLAVATA2 and CORYNE in Arabidopsis. Plant J. 61, 223–233. doi: 10.1111/j.1365-313X.2009.04049.x
Keywords: auxin, cell wall, cytokinin, intercellular communication, organ primordia, peptide hormone, physical stress, shoot apical meristem
Citation: Tameshige T, Hirakawa Y, Torii KU and Uchida N (2015) Cell walls as a stage for intercellular communication regulating shoot meristem development. Front. Plant Sci. 6:324. doi: 10.3389/fpls.2015.00324
Received: 16 February 2015; Accepted: 24 April 2015;
Published: 11 May 2015.
Edited by:
Masaru Fujimoto, The University of Tokyo, JapanReviewed by:
Mitsuhiro Aida, Nara Institute of Science and Technology, JapanThorsten Hamann, Norwegian University of Science and Technology, Norway
Copyright © 2015 Tameshige, Hirakawa, Torii and Uchida. This is an open-access article distributed under the terms of the Creative Commons Attribution License (CC BY). The use, distribution or reproduction in other forums is permitted, provided the original author(s) or licensor are credited and that the original publication in this journal is cited, in accordance with accepted academic practice. No use, distribution or reproduction is permitted which does not comply with these terms.
*Correspondence: Keiko U. Torii and Naoyuki Uchida, Institute of Transformative Bio-Molecules (WPI-ITbM), Nagoya University, Furo-cho, Chikusa-ku, Nagoya 464-8602, Japan,a3RvcmlpQHUud2FzaGluZ3Rvbi5lZHU=;dWNoaW5hb0BpdGJtLm5hZ295YS11LmFjLmpw