- 1Forest Genetics and Physiology Research Group, School of Forest Engineering, Technical University of Madrid, Madrid, Spain
- 2Department of Plant Biology, Faculty of Biology, University of Barcelona, Barcelona, Spain
The photosynthesis source–sink relationship in young Pinus canariensis seedlings was modified by stem girdling to investigate sprouting and cambial activity, feedback inhibition of photosynthesis, and stem and root hydraulic capacity. Removal of bark tissue showed a trade-off between sprouting and diameter growth. Above the girdle, growth was accelerated but the number of sprouts was almost negligible, whereas below the girdle the response was reversed. Girdling resulted in a sharp decrease in whole plant transpiration and root hydraulic conductance. The reduction of leaf area after girdling was strengthened by the high levels of abscisic acid found in buds which pointed to stronger bud dormancy, preventing a new needle flush. Accumulation of sugars in leaves led to a coordinated reduction in net photosynthesis (AN) and stomatal conductance (gS) in the short term, but later (gS below 0.07 mol m-2 s-1) AN decreased faster. The decrease in maximal efficiency of photosystem II (FV/FM) and the operating quantum efficiency of photosystem II (ΦPSII) in girdled plants could suggest photoprotection of leaves, as shown by the vigorous recovery of AN and ΦPSII after reconnection of the phloem. Stem girdling did not affect xylem embolism but increased stem hydraulic conductance above the girdle. This study shows that stem girdling affects not only the carbon balance, but also the water status of the plant.
Introduction
Photosynthesis products are modulated by source–sink equilibria within the plant (Jang and Sheen, 1994) and hydraulic constraints (Brodribb and Field, 2000) including the activity of root meristems. Phloem serves as the long-distance transport pathway for photosynthate movement from source leaves to regions of active growth, storage structures, and other non-photosynthetic cells. Natural loss of phloem and bark can occur after severe perturbations such as wounding caused by volcanic activity (Rodríguez Martín et al., 2013) or when small rodents chew away the bark down to the cambium layer of seedlings during winter. Artificial girdling has traditionally been used to study apical control of branch growth (Münch, 1938) and the response dynamics of phloem transport (Parker, 1974), and to assess the effect of ion redistribution between phloem and xylem on xylem flow rate (Zwieniecki et al., 2004). In horticulture, girdling has been widely applied to increase flowering, fruit set and fruit size (Williams et al., 2000; Di Vaio et al., 2001; Williams and Ayars, 2005; Rivas et al., 2007; Urban and Alphonsout, 2007) and in forestry it has been used to alter wood properties (Noel, 1970). The effect of stem girdling at the base of the stem differs from the effect of branch girdling commonly used in agricultural practices, because besides promoting carbohydrate accumulation above the girdle it prevents phloem transport to the roots. Despite its ecological significance, studies about the physiological effects of complete stem girdling in forest trees are scarce compared to those carried out on branch girdling in horticulture.
The Canary Island pine (Pinus canariensis) is endemic to the Canary Islands with a broader distribution in the past, when volcanism represented a strong selection factor (Frankis, 1999). It is one of the few pines able to resprout after severe injury, a trait traditionally associated with fire resilience (Climent et al., 2004), and it is also capable of surviving after partial girdling of the stem, with only a small strip of phloem 3 cm wide, for more than 60 years (Figure 1).
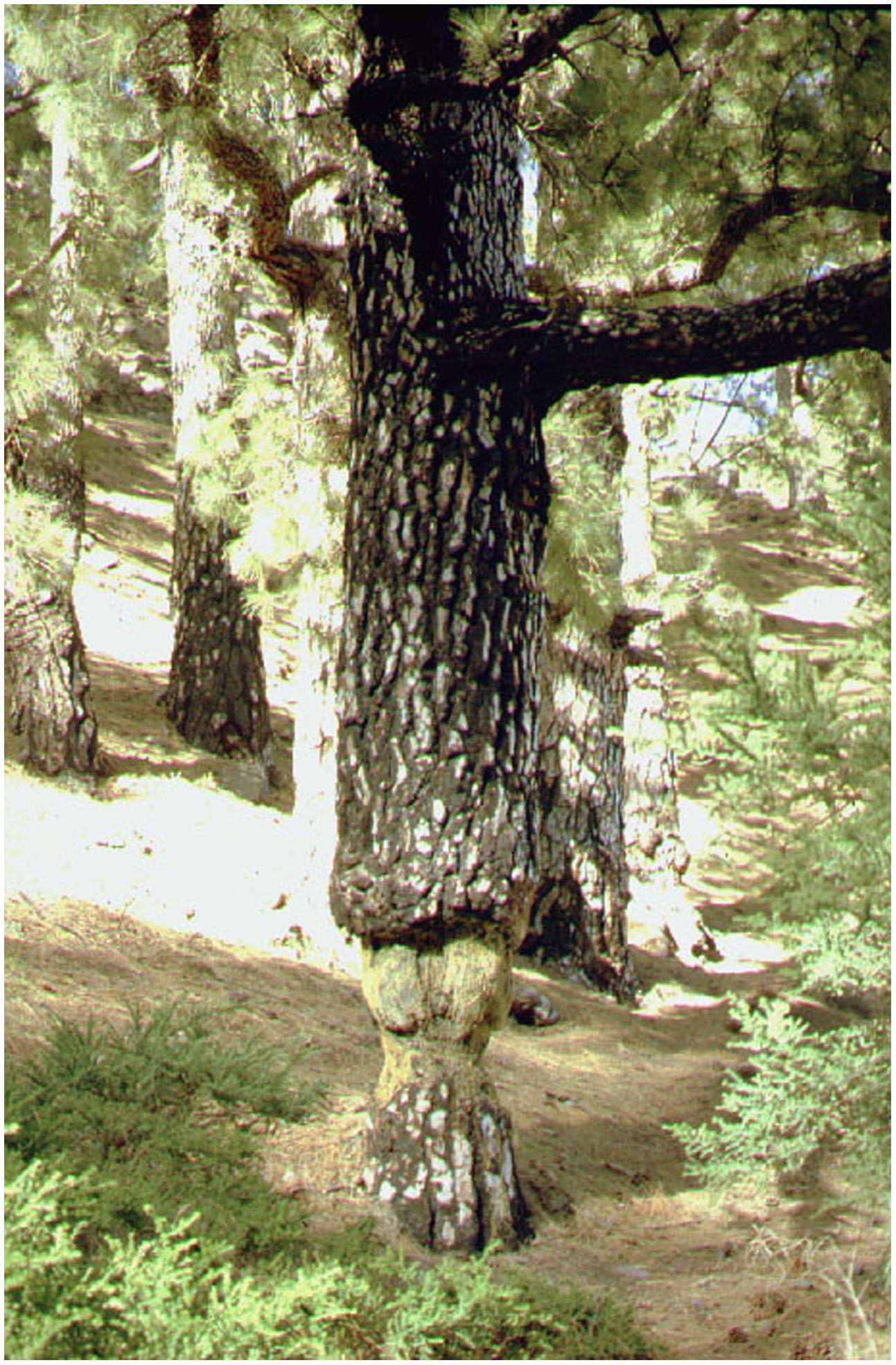
FIGURE 1. Pinus canariensis showing severe girdling (10 cm wide), with a small strip of phloem 3 cm wide. The tree was 65 years old when girdled and had a diameter of 56 cm. After 55 years, diameter increment was 1 cm below the girdle, compared to 30 cm above.
The first visual symptom after girdling is an increase in stem diameter above the girdle due to growth of bark and xylem stimulated by the accumulation of soluble sugars and starch, coupled with the interruption of stem growth below the girdle (Daudet et al., 2005; De Schepper et al., 2010). Roots are gradually depleted of their carbohydrate reserves and can suffer starvation (Weaver and McCune, 1959). Accumulation of carbohydrates above the girdle enhances the respiratory potential of the stem and branches and also increases leaf mass per area (LMA; Domec and Pruyn, 2008). This stock of sugars has been used to explain the decline in leaf photosynthesis due to end-product inhibition (Iglesias et al., 2002) and the reduction in quantum yield efficiency of photosystem II through feedback loops (Jang et al., 1997; Myers et al., 1999; Rivas et al., 2007; Urban and Alphonsout, 2007; Murakami et al., 2008). At the same time, removal of the sink demand of roots generally results in decreased stomatal conductance and transpiration, accumulation of abscisic acid (ABA) in leaves (Setter et al., 1980), and an increase in leaf water potential (Williams et al., 2000).
Although some studies assumed that girdling affects only carbon status and not water status (e.g., Daudet et al., 2005; Murakami et al., 2008), there is evidence that disruption of phloem transport reduces xylem conductivity and embolism repair, at least in some species (Salleo et al., 1996; Zwieniecki and Holbrook, 1998; Christman et al., 2012). The partial closure of stomata in girdled trees leads to a reduction in leaf hydraulic conductance (Domec and Pruyn, 2008; Sellin et al., 2013) resulting from regulation of membrane aquaporins (Kuwagata et al., 2012) or even occlusion of leaf veins (Sellin et al., 2013), and also to a decline in branch hydraulic capacity following changes in the sapwood to leaf area ratio (Domec and Pruyn, 2008). The long-term effect, even when the phloem has reconnected, entails changes in cambial activity in the following years. In conifers, tracheids are shorter and wider and more efficient in water transport but less resistant to cavitation in girdled trees (Domec and Pruyn, 2008). Girdling changes the properties of sapwood, particularly below the girdle, where stem moisture content and parenchyma viability are significantly reduced (Taylor and Cooper, 2002).
Blocking the downward translocation of photosynthates and metabolites through the phloem can also affect leaf size and number (Fumuro, 1998) and promote sprouting (De Schepper et al., 2010). To our knowledge, the effect on the potential trade-offs between sprouting and cambial activity as carbohydrate sinks has never been assessed. Sprouting as a means of persistence is thought to be strongly linked to the frequency, severity and predictability of disturbances and how these disturbances interact with regrowth rates (Bond and Midgley, 2003). Sprouter species from fire-prone ecosystems generally allocate more carbon to starch or other reserves in the roots than non-sprouters (Bell and Ojeda, 1999). Thus the effect of girdling on roots and radial growth could be lessened in these species. Sprouting is a ubiquitous feature of temperate angiosperm trees, at least in saplings (Del Tredici, 2001), but among conifers this ability is restricted in adults to a few species (Keeley and Zedler, 1998; Del Tredici, 2001).
In this study we monitor whole-plant physiological responses to girdling in 3-years-old seedlings of Canary Island pine to identify potential tradeoffs between hydraulic properties and photosynthesis and their influence in growth and aboveground biomass production. Two girdling treatments were tested to assess the impact of phloem reconnection in some plants. We hypothesized that (i) sprouting would be profuse after girdling, affecting radial growth of the stem (ii) root depletion of carbohydrates would decrease hydraulic conductivity, affecting leaf water status, triggering stomatal closure and eventually damaging the photosynthetic apparatus, and (iii) carbon starvation in roots would increase ABA synthesis promoting leaf senescence via hormone signaling.
Materials and Methods
Plant Material and Phloem Girdling
A total of 60 3-years-old P. canariensis trees were grown in a greenhouse in 5 L pots filled with 1/4 perlite and 3/4 peat, v:v, and fertilized with a controlled release fertilizer (Nutricote, Chisso Asahi Fertilizer, Japan). At the beginning of May 2011, the plants were distributed in three blocks according to size. Average tree height in each block was 105, 80, and 70 cm and average basal diameter (i.e., at ground level) was 9.4, 9.2, and 7.0 cm, respectively. Two girdling treatments were applied in each block: fine girdling, (GF, 3 mm strip of bark, five plants per block) and wide girdling (GW, 15 mm strip of bark, 10 plants per block) and remaining plants were used as controls (C, five plants per block). In the girdled trees, a strip of bark (periderm, cortex, and phloem) was removed by inserting a razor blade at 1/3 height from the base (Figures 2A,B). All needles below the wound were removed.
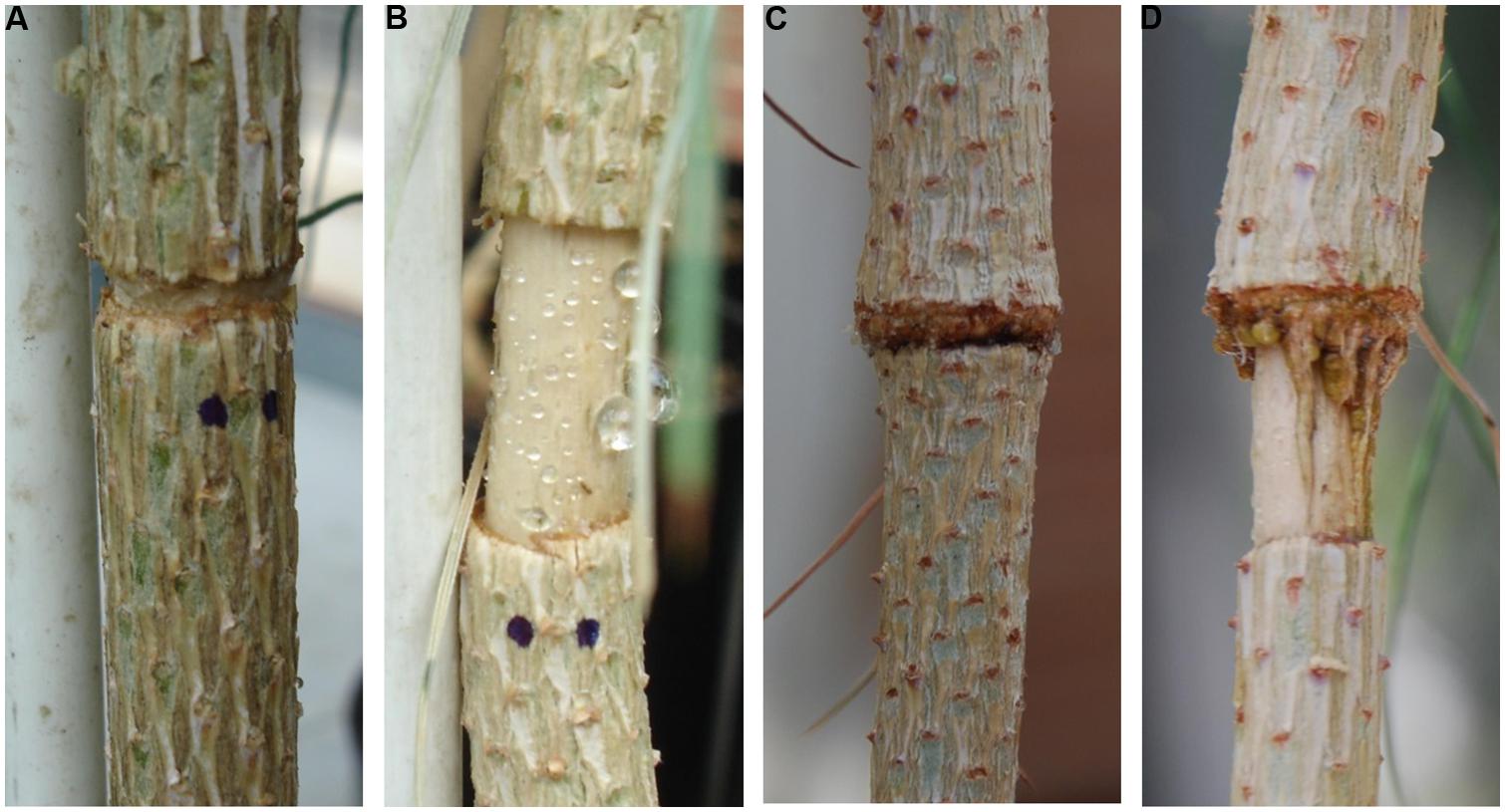
FIGURE 2. Girdling treatments: (A) fine girdling (GF) and (B) wide girdling (GW) 1 week after girdling. Formation of wound tissue on sites where bark tissue was removed 50 days after girdling (d.a.g.): (C) reconnection of the phloem in GF and (D) vertical strands from the top of the girdle in GW growing over to reconnect the two sides of the girdle.
Radial Growth and Aboveground Biomass
Stem diameter variations above and below the girdle (U and L, respectively) and in the base of the stem (B) were measured throughout the experiment with a digital caliper. The number of sprouts in U, L, and B was counted and sprouts were removed periodically after girdling to avoid new sources of carbohydrates below the girdle. Aboveground dry biomass was measured at the beginning of July (56 days after girdling, from now d.a.g.) in all plants from block 1 and at the end of the experiment (145 d.a.g.) in plants from blocks 2 and 3. After plant harvest, needles were detached and the stem was used for hydraulic conductance measurements. Stem and needle dry weights were measured after drying to constant weight at 80°C.
Plant Transpiration and Hydraulic Conductance
Whole plant transpiration was estimated gravimetrically by measuring the loss of mass from the pot every two-3 days before watering to field capacity in two phases. The first phase was from the beginning of the experiment until the first harvest (56 d.a.g.) and the second was from 112 d.a.g. to the final harvest (145 d.a.g.). Plants were kept well-watered throughout the experiment. Leaf area specific transpiration for each block and treatment was calculated as the maximum transpiration in the week previous to harvest per unit of projected leaf area.
Just after the first harvest, stem hydraulic conductance (kh) and native embolism, i.e., initial embolism naturally occurring or caused by the girdling treatment, were measured in two segments in L and in three segments in U with a XYL’EM (Xylem Embolism Meter, Bronkhorst, Montigny les Cormeilles, France). All segments were perfused with degassed and filtered (0.2 μm) 10 mM KCl and 1 mM CaCl2 solution in ultrapure water. Initial conductance (kin) was measured at low pressure (6 kPa) after the stems were cut under water. Embolism was removed at high pressure (0.18 MPa) for 20 min and maximum hydraulic conductance (kmax) was determined. For each segment, stem specific conductivity (KS) was calculated by multiplying kmax by sample length and dividing by xylem cross section and leaf-specific hydraulic conductivity (LSC), as the conductivity per unit of projected leaf area.
Whole root system conductance (kroot) was measured on severed root systems kept in their substrate, with the stump connected to a high pressure flow meter (HPFM, Dynamax Inc., Houston, TX, USA). Shoots were excised about 1 cm above the root collar and attached to the HPFM. Measurements were made in the transient mode. During each measurement the pressure was increased from 0.03 to 0.45 MPa at a rate of 5–8 KPa s-1 and the flow rate was recorded every 3 s. kroot was calculated from the linear region of the relationship between flow rate and pressure (Tyree et al., 1995). Two to eight measurements were made in each plant. We did not observe any pattern of decrease/increase in kroot with measurements. We therefore hypothesized that any change in kroot between measurements made on a single plant was caused by partial tracheid blockage with resin. Leaf-specific root hydraulic conductance (LScroot) was calculated as the root conductance per unit of projected leaf area.
Wood Density
Wood density was determined on 2.5 cm-long segments above and below the girdle. Green volume of the wood sample was determined according to Archimedes’ principle. Samples were stored at 105°C for 48 h and their dry weight was measured. Basic density was calculated as the ratio of dry weight to green volume.
Gas Exchange and Chlorophyll Fluorescence
Simultaneous measurements of gas exchange and chlorophyll fluorescence were made with an Li-6400 open gas exchange system (Li-Cor, Lincoln, NE, USA) and with an Li-6400-40 integrated fluorescence chamber (Li-Cor, Lincoln, NE, USA) on samples of nine fully expanded adult needles. Measurements were made in May, June, and September. Each block was measured on consecutive days (18, 19, 20 d.a.g., 35, 36, 37 d.a.g., and 140, 141 d.a.g.). All surviving plants were measured each time. As one block was harvested in July (56 d.a.g.), only two blocks were measured in September. Gas exchange parameters – net photosynthesis (AN), stomatal conductance (gS), transpiration rate (E), and leaf temperature (TL) – were measured with saturating light at ambient CO2 and ambient temperature. Steady state fluorescence (FS) and maximum fluorescence from a light adapted sample (FM’) were calculated after saturation flash (8000 μmol m-2 s-1, 0.8 s). The operating quantum efficiency of photosystem II (ΦPSII) was calculated as ΦPSII = 1 – FS/FM’ (Genty et al., 1989).
Chlorophyll fluorescence was measured separately from gas exchange, on the same plants and 2–3 days after the other measurements, from 9:00 to 11:30 h, on nine fully expanded adult needles after at least 40 min of dark adaptation. The ratio of variable to maximum chlorophyll fluorescence (FV/FM) was calculated as (FM – F0)/FM, where FM and F0 were, respectively, the maximum and basal fluorescence yields of dark-adapted needles. This parameter provides an estimate of the maximum photochemical efficiency of photosystem II.
Chlorophyll Content, Leaf Mass per Area and Leaf Relative Water Content
Three times during the experiment, at 35, 50, and 140 d.a.g., chlorophyll a and b and carotenoid concentrations in three needles per plant were determined spectrophotometrically after extraction with dimethyl sulfoxide at 60°C for 4 h, following Wellburn (1994).
At the end of the experiment, LMA was measured in 18 needles from each sampled tree. Needle projected area was obtained with a scanner and the images were analyzed with WinFOLIA (Regent Instruments). Needles were dried at 80°C for 2 days to determine leaf dry mass and LMA. In another subsample of needles, relative water content (RWC) was determined as: RWC = (FW – DW)/(TW – DW), where FW is fresh weight, TW is turgid weight after rehydrating needles for 24 h at 4°C in darkness, and DW is dry weight after oven-drying needles for 48 h at 80°C.
Hormone Concentration
Concentrations of ABA, abscisic acid glucose ester (ABA-GE), and jasmonic acid (JA) in xylem, needles, buds, and phloem above and below the girdle were simultaneously analyzed by HPLC MS/MS, as described by Brossa et al. (2011). 100 mg fresh tissue was ground in liquid nitrogen with a mortar and pestle and extracted with 750 μl methanol–water–acetic acid (90:9:1 v/v/v). Deuterium-labeled internal standards (40 ng ABA-d6, 40 ng ABA-GE-d2, and 40 ng JA-d5) were added to each sample at the beginning of the extraction procedure. Extracts were vortexed for 5 min and incubated for 10 min at 4°C under ultrasonication (Vibra-Cell Ultrasonic Processor, Sonics & Materials Inc., Newtown, CT, USA) then centrifuged for 10 min at 10,000 rpm. The supernatants were collected and the pellets were re-extracted with 750 μl of the extraction solvent. Pellets were then pooled and filtered. 5 μl of each sample was injected into the LC system (Acquity UPLC, Waters) using a Waters X-Bridge C18 column (3.5 μm; 100 9 2.1 i.d.). The MS/MS quantification was performed on an API 3000 triple quadrupole mass spectrometer (AB Sciex, Danaher Corp., Washington, DC, USA) using multiple reaction monitoring (MRM) acquisition with the corresponding transitions for each analyte.
Statistical Analysis
The effects of treatment, block and position in the stem (U, L, and B) on diameter and number of sprouts were checked using repeated measures analyses of variance (ANOVA). Repeated measures ANOVA were also run to analyze transpiration, gas exchange, chlorophyll fluorescence, and chlorophyll content. Wood density was analyzed using a GLM approach to ANOVA with the factors treatment and block. One-way ANOVA with treatment as the only factor was performed on aboveground biomass, LMA, RWC, hydraulic conductance parameters, and hormone content. All factors were considered fixed for all the analyses. Differences between levels of significant predictors were tested by Duncan’s multiple-range tests.
Pearson’s product-moment correlation coefficients were calculated to relate gas exchange, chlorophyll fluorescence and growth traits. Bivariate relationships between the traits studied and independent variables were assessed using simple linear or non-linear least squares regression.
Results
Stem Diameter Variations and Sprouting
Girdling resulted in immediate reduction in radial growth of the segment below the girdle (L) and later of the base of the stem (B), whereas growth of the upper segment (U) increased significantly (Figure 3). Approximately 48 d.a.g., coinciding with the apparent reconnection between U and L in most plants with fine girdling (GF), the rate of radial growth of U in GF decreased, and after this date, the upper segment of plants with wide girdling (GW) was significantly wider than in GF (p < 0.001; Figure 3A).
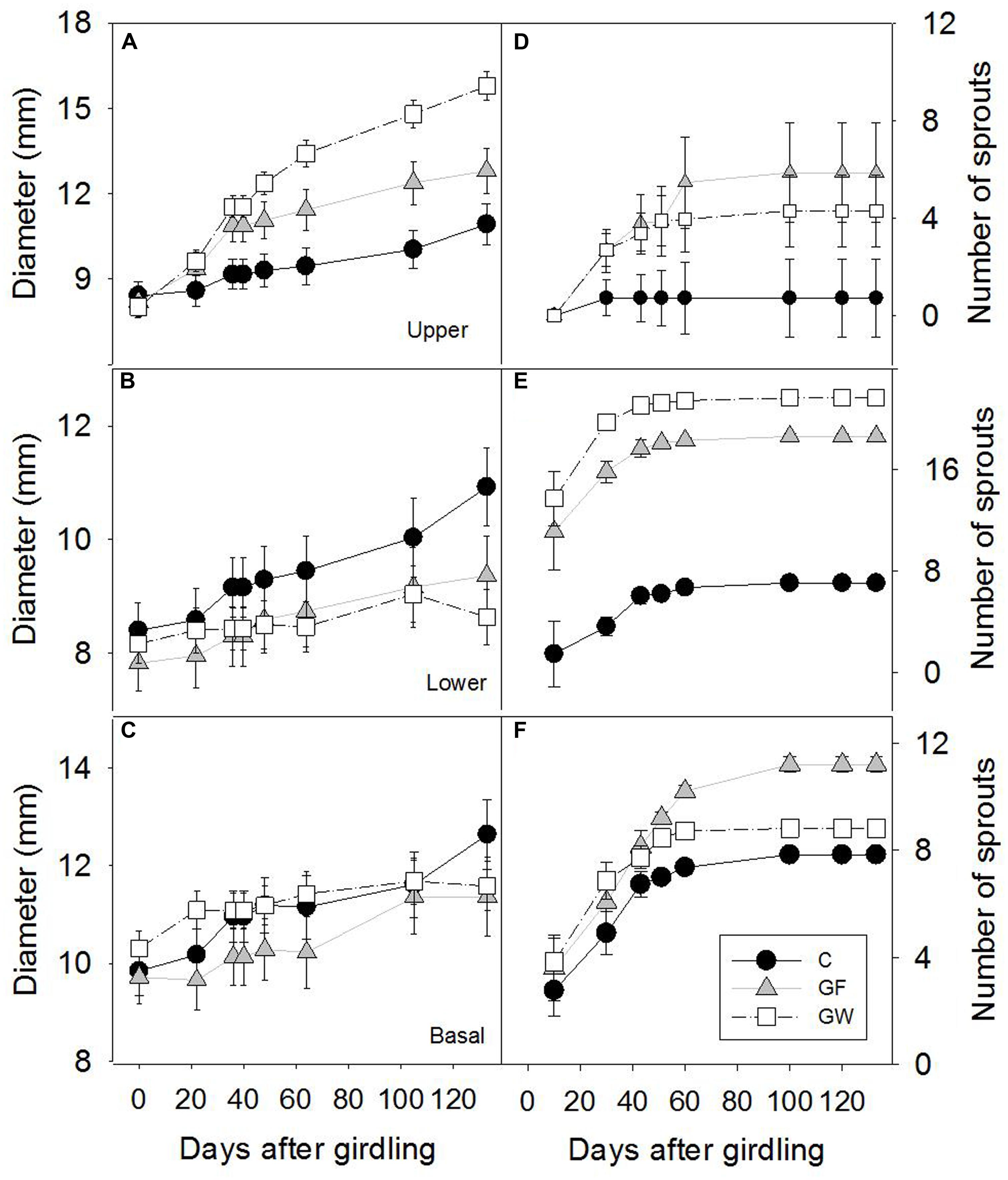
FIGURE 3. Time frame of stem diameter growth (A) above the girdle (U), (B) below the girdle (L) and (C) in the base of the stem (B) and accumulated number of sprouts (D) above the girdle, (E) below the girdle and (F) in the base of the stem in control (C), in fine girdled (GF) and wide girdled (GW) plants. Error bars represent the standard error.
Sprouting was profuse after girdling during the first month of the experiment, but from 50 d.a.g. it virtually ceased. The number of sprouts in B and L was much higher than in U (Figures 3D–F). In general, profuse sprouting was associated with lower diameter growth (Figure 4). Total leaf area and aboveground dry mass were higher in C than in GF and GW in the first harvest but differed significantly only between C and GW in the final harvest (Table 1). At the end of the experiment, LMA in GW was higher and RWC was lower than in C and GF (Table 1).
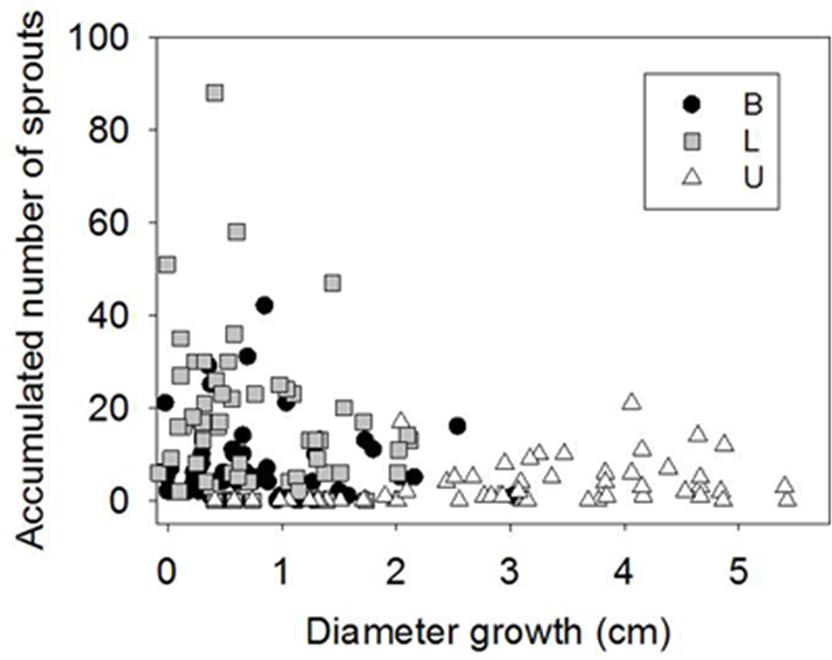
FIGURE 4. Diameter growth vs. accumulated number of sprouts in control and girdled plants in the base of the stem (B), below the girdle (L) and above the girdle (U) 48 d.a.g.
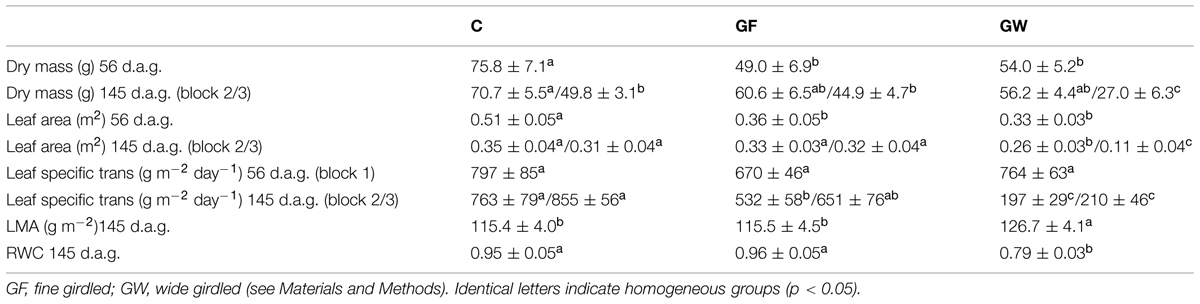
TABLE 1. Mean values (±SE) for aboveground dry mass, leaf area, and maximum leaf specific transpiration measured 56 days after girdling (d.a.g.) and at the end of the experiment (145 d.a.g.), leaf mass per area and relative water content of control (C) and girdled Pinus canariensis.
Transpiration was lower in girdled than in control plants throughout the experiment but differences were considerable in the second phase, after the warmest months, when water loss in GW was four times lower than in C. Although in this second phase most GF had apparently reconnected the vascular tissue, transpiration did not fully recover and was 25% lower than in C (Figure 5), despite the lack of differences in leaf area between C and GF (Table 1). When considering leaf area specific transpiration, differences between treatments were not significant in the first phase of the experiment, whereas in the second phase the whole plant transpiration pattern was confirmed (Table 1).
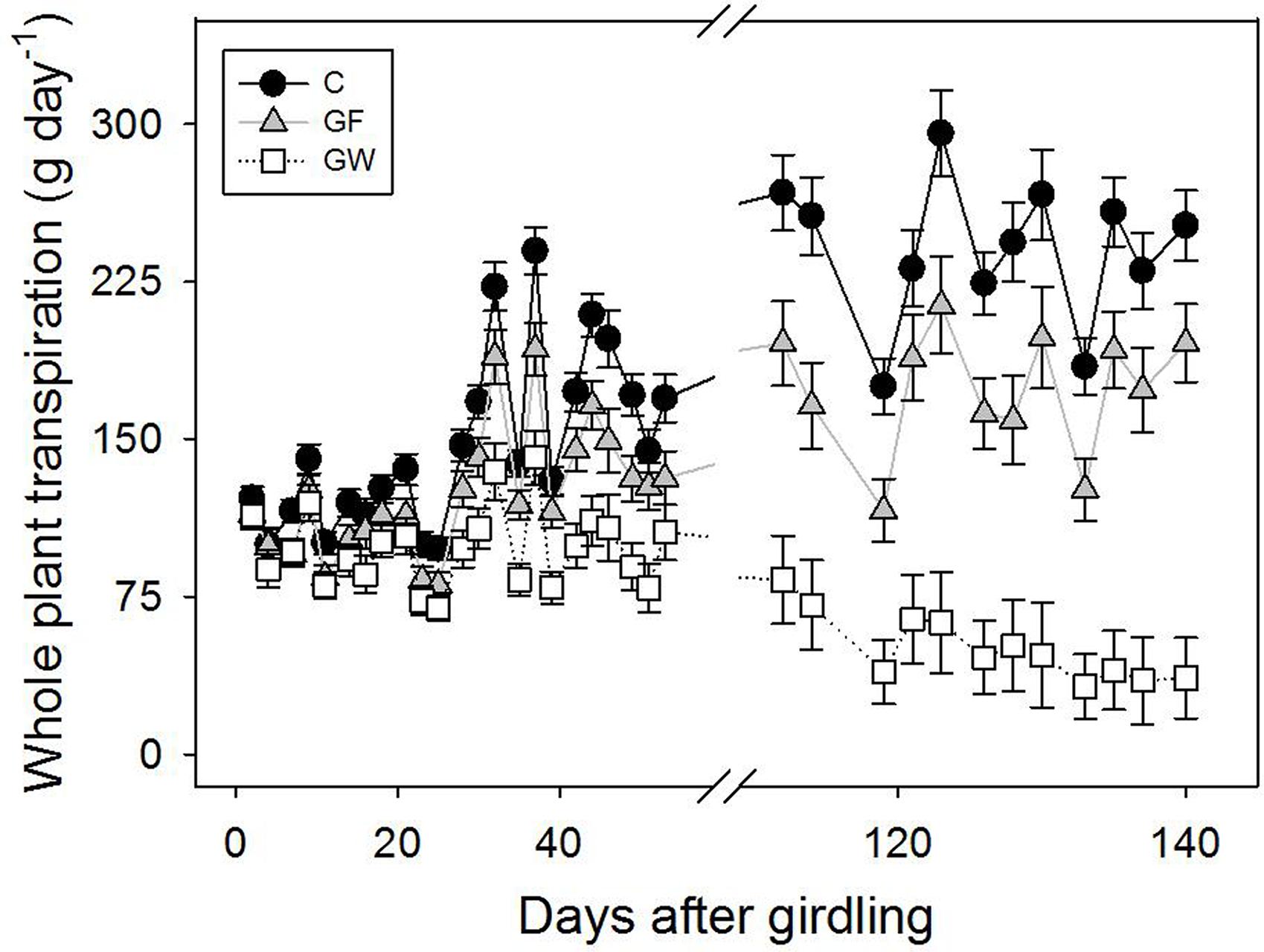
FIGURE 5. Changes in whole plant transpiration in control (C), fine girdled (GF), and wide girdled (GW) plants. Reconnection of the phloem in GF was ca. 48 d.a.g. Error bars represent the standard error.
Gas Exchange and Chlorophyll Fluorescence Parameters
Gas exchange and chlorophyll fluorescence values 18 d.a.g. were similar among treatments. On following dates, net photosynthesis (AN), transpiration (E) and stomatal conductance (gS) decreased strongly in GW and 140 d.a.g. gas exchange values were more than 10 times lower than in C and GF (Figures 6A–C). ΦPSII decreased steadily in GW, reaching a value of 0.05 at the end of the experiment, five times lower than in C and GF (Figure 6E). At 35 d.a.g. AN differed between girdled and control plants, whereas we found significant differences in gS and E between blocks in girdled plants: larger plants did not differ from C whereas smaller plants showed lower values (Figures 6B,C). At the end of the experiment, values in GF recovered to C values. FV/FM remained almost constant at 0.85 in C throughout the experiment. At 35 d.a.g. we again found a block effect in girdled plants: FV/FM of larger plants was 0.85 but in smaller plants it decreased to 0.82. At 140 d.a.g. FV/FM almost recovered to C values in GF, but GW values dropped to 0.63 (Figure 6F). At 35 d.a.g. chlorophyll content and carotenoids in both GW and GF were lower than in C. However, measurements made 50 and 140 d.a.g. showed that photosynthetic pigment content of GF had recovered and differed significantly from GW (Table 2). The chlorophyll a:chlorophyll b ratio increased over time in C and GF, whereas in GW we observed a substantial decrease from 50 to 140 d.a.g. (Table 2). In contrast, carotenoids were more abundant at the beginning of summer (35 d.a.g.).

TABLE 2. Mean values (±SE) for chlorophyll content (Chla+b), chlorophyll a:chlorophyll b ratio (Chla+b) and carotenoids (Carot) 35, 50, and 140 days after girdling (d.a.g.) in control (C), fine girdled (GF), and wide girdled (GW) Pinus canariensis.
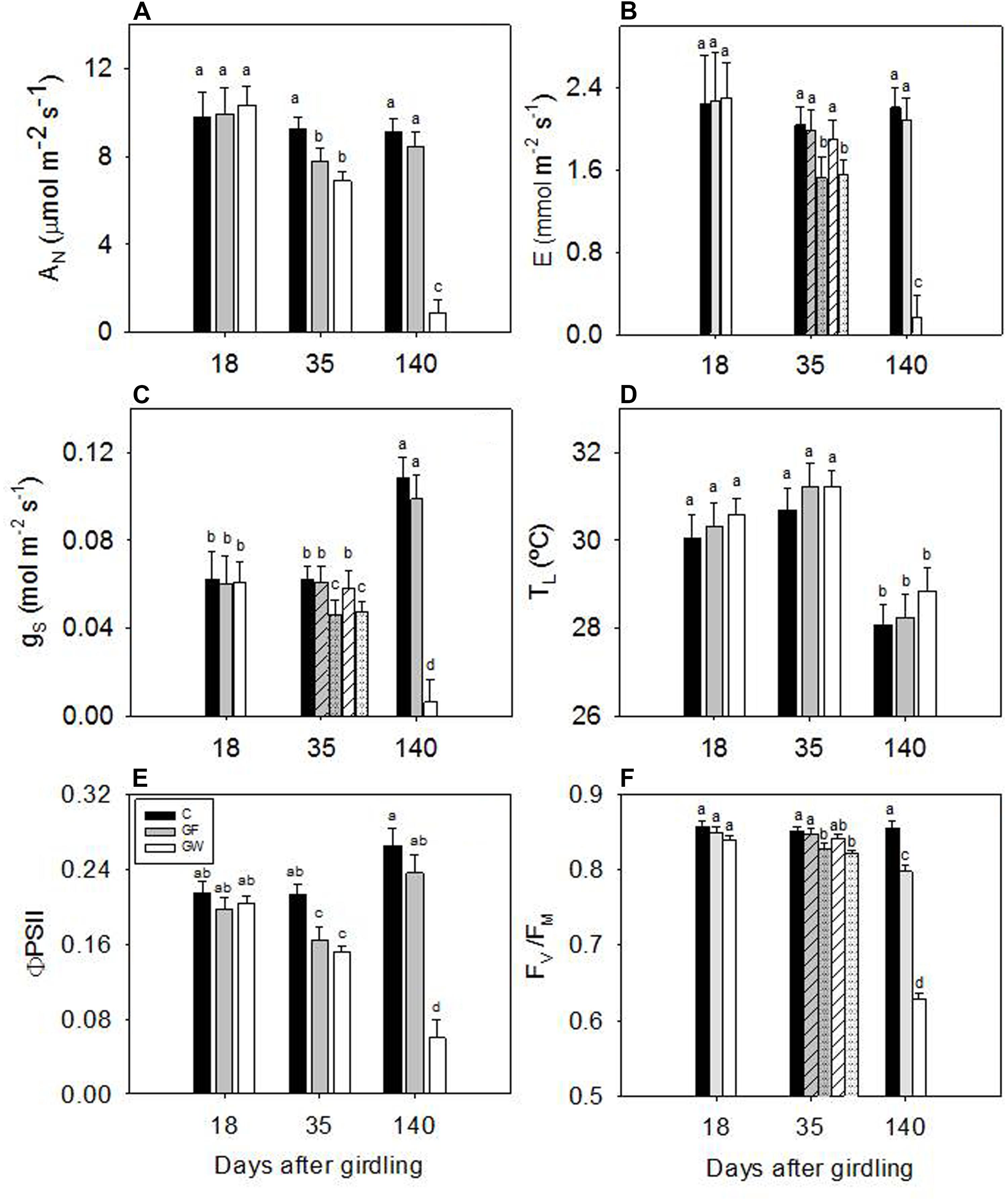
FIGURE 6. Changes in (A) net photosynthesis (AN), (B) transpiration rate (E), (C) stomatal conductance to water vapor (gS), (D) leaf temperature (TL), (E) operating quantum efficiency of photosystem II electron transport (ΦPSII) and (F) maximum photochemical efficiency of photosystem II in dark adapted leaves (FV/FM) in response to phloem girdling. Error bars represent the standard error and significant differences (p < 0.05) are indicated by different letters. Differences between blocks 35 d.a.g. in E, gS and FV/FM in girdled plants are shown with striped bars for larger plants (block 1) and dotted bars for plants in blocks 2 and 3.
AN was strongly related to gS and ΦPSII for all three treatments. The relationship was linear with ΦPSII (Figure 7B), whereas gS and AN responded coordinately at first but from gS below 0.07 mol m-2 s-1, AN decreased faster (Figure 7A). Variation of leaf temperature was similar in all treatments: 28.4 ± 0.5 C in September and 30.9 ± 0.7 C in the middle of June (Figure 6D).
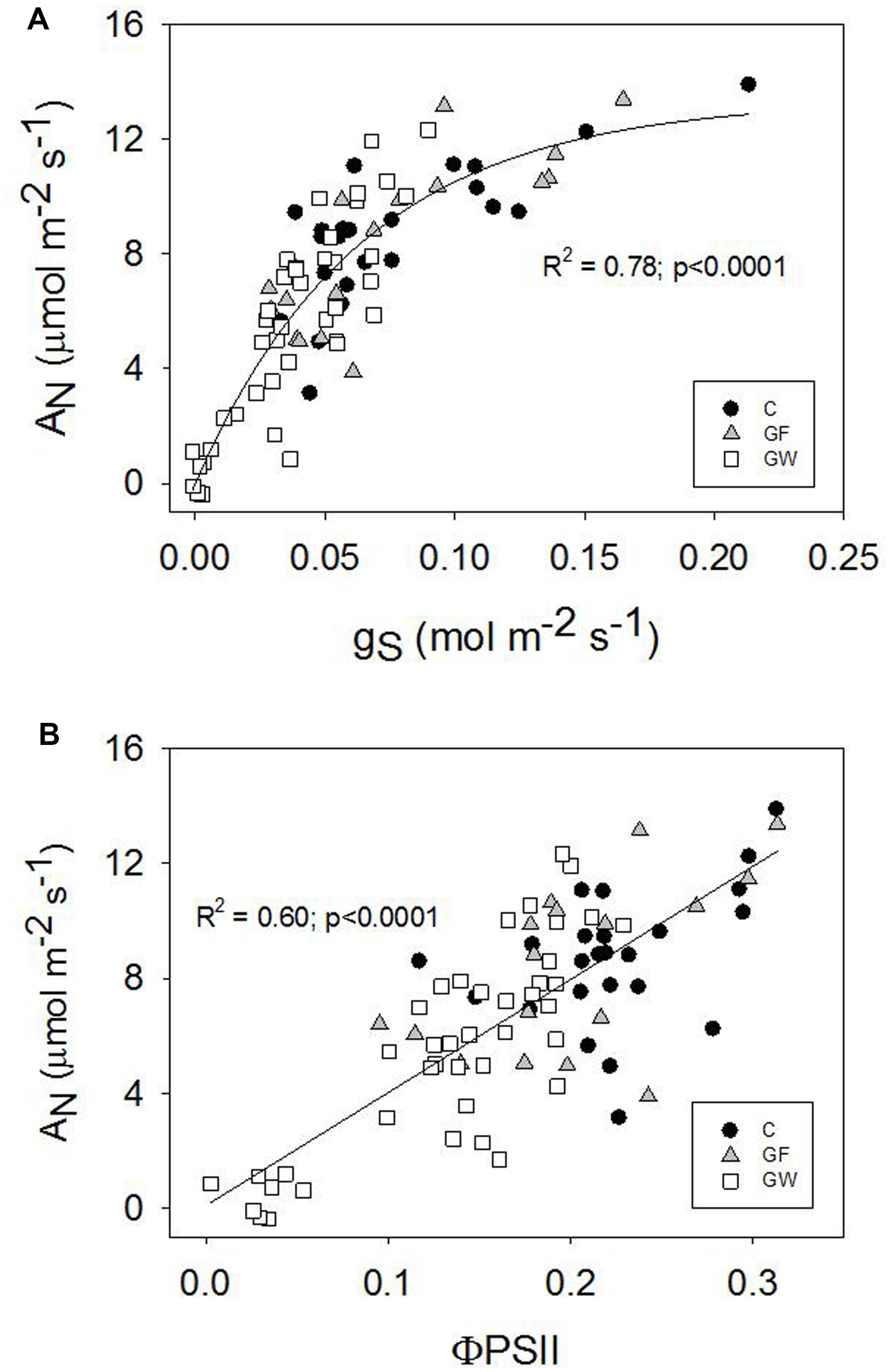
FIGURE 7. (A) Stomatal conductance (gS) vs. net photosynthetic rate (AN) and (B) operating quantum efficiency of photosystem II electron transport (ΦPSII) vs. net photosynthetic rate (AN), combining control and girdled plants.
Hydraulic Conductivity and Wood Density
Girdling slightly increased wood density in both U and L and triggered a significant decrease in Kh in L and an increase in U, particularly in GW. KS did not differ between segments or treatments but LSC was significantly higher in U in girdled plants (Table 3). Girdling did not cause xylem embolism in any of the samples. Maximum hydraulic conductance of the roots was affected by girdling and kroot in C was almost twice kroot in GF and GW (Table 3). Despite the reduction of leaf area of girdled plants during the first phase of the experiment (Table 1), LScroot decreased in GF and GW by 28% (Table 3). kroot was positively correlated with maximum transpiration during the first phase of the experiment, measured 37 d.a.g. (r = 0.83) and ΦPSII (r = 0.72), but was negatively correlated with kh (r = -0.55) and WD (r = -0.75) in U.
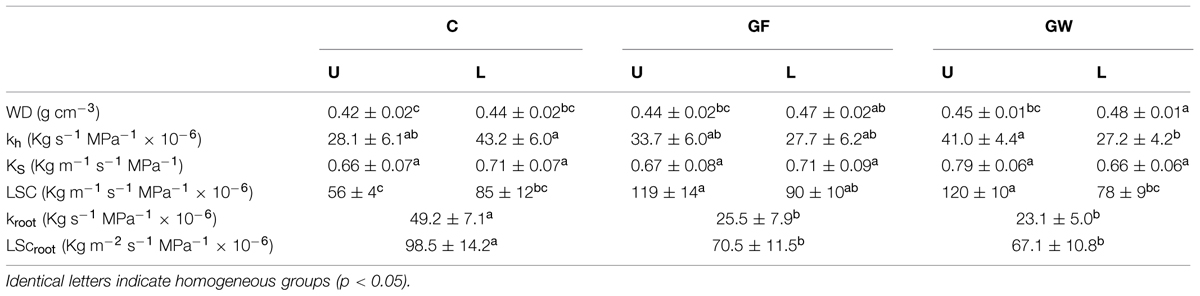
TABLE 3. Mean values (±SE) for wood density (WD), hydraulic conductance (kh), specific hydraulic conductivity (KS), leaf specific conductivity (LSC), root conductance (kroot) and leaf specific root hydraulic conductance (LScroot) above (U) and below (L) the girdle 56 days after girdling (d.a.g.) in control (C), fine girdled (GF), and wide girdled (GW) Pinus canariensis.
Concentration of Abscisic Acid and Jasmonates
Abscisic acid content in C and GF was almost negligible, whereas in the xylem, upper phloem, and buds in GW we detected up to 33 nmol g-1 (Table 4). ABA-GE was abundant in the needles of plants in all treatments and also in buds in GW, where we found the highest content: 23.1 nmol g-1. No differences were found for JAs (Table 4).
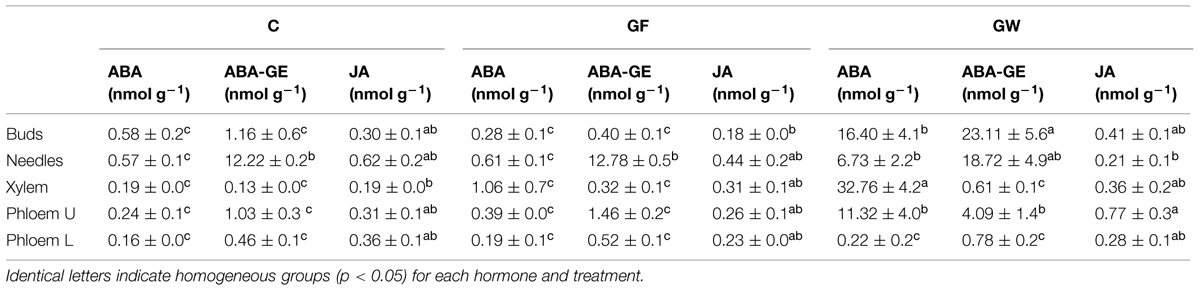
TABLE 4. Mean concentration (±SE) of abscisic acid (ABA), abscisic acid glucose ester (ABA-GE), and jasmonic acid (JA) in xylem, needles, buds and phloem above (U) and below (L) the girdle 145 days after girdling (d.a.g.) in control (C), fine girdled (GF), and wide girdled (GW) Pinus canariensis.
Discussion
Sprouting and Cambial Growth
Girdling changed the carbon source–sink equilibrium in the trees. Roots no longer functioned as the sink of new assimilates, which tended to accumulate just above the girdle (Singh et al., 2003), triggering the increase in stem diameter above the girdle (zone U) due to irreversible structural growth and reversible swelling of living bark cells (De Schepper et al., 2010). In contrast, stem diameter growth ceased almost immediately just below the girdle (L), whereas in the base of the stem (B) it slowed down and girdled plants differed from controls only at the end of the experiment (Figure 3). Although cambial activity was directly affected by girdling (Chano et al., 2015), wood density barely changed. Only wood density of L in GW differed slightly from C (Table 3) but we could not discard that this change in density reflected changes in water content and thus green volume between C and GW plants. Wound tissue developed rapidly only from U (Figure 2D), and ca. 48 d.a.g. most GF plants had reconnected both sides of the girdle (Figure 2C) and the rate of diameter growth in U and L tended to balance out (Figures 3A,B).
Profuse sprouting was activated in L, indicating mobilization and consumption of stored carbohydrates in the roots as a response to serious above-ground damage to the plant (Del Tredici, 2001; Landhausser and Lieffers, 2002). Similar new basal shoots have been observed in other girdling studies with angiosperms (Wan et al., 2006; De Schepper et al., 2010). It appears that the available sugars were allocated to different purposes in U and L. Whereas the healing process and diameter growth were prioritized above the girdle (Chano et al., 2015), production of new photosynthetic material was the priority below the girdle as a consequence of blocked auxin transport (Wan et al., 2006). It may also have been due to higher concentrations of root-borne cytokinins, which promote the development of stem buds and shoots (Schmuelling, 2002). Profuse sprouting and reduced stem growth below the girdle supported a trade-off between cambial activity and sprouting (Figure 4) previously found in Fagus sylvatica, associated with local competition for carbohydrates between buds and close cambium (Collin et al., 2012). The interruption of sprout production approximately 30 d.a.g. in L and 60 d.a.g. in B may have indicated depletion of carbohydrates stored in the roots, as sprouting vigor is affected by the amount of the reserves in the roots (Zhu et al., 2012). When girdling was reversed, i.e., the phloem was reconnected, stem diameter growth below the girdle restarted but did not reach the same total increment of control trees (Figure 3).
Water Use and Aboveground Biomass
The sharp decrease in whole plant transpiration 1 week after girdling onward may have been caused by foliage shedding or stomatal closure. Less aboveground dry mass and lower leaf area in girdled plants in the first harvest (56 d.a.g.) suggested that needle loss or less elongation of new needles could have partially triggered the reduction of plant transpiration as confirmed by negligible changes in leaf area specific transpiration. Decrease in gS appeared to contribute more to reducing transpiration several weeks after girdling, as no drop in gs was detected 18 d.a.g. In the second measurement (35 d.a.g.), gS had still not descended in larger plants, but in smaller girdled plants it decreased by up to 40% compared with controls. Later in the experiment (after summer), gS and whole plant transpiration were almost negligible in GW, whereas in C and GF they increased, although leaf area specific transpiration did not fully recover in GF. Accumulation of carbohydrates in the needles of girdled trees is known to inhibit photosynthesis (Iglesias et al., 2002) and induce consequent stomatal closure. The significant increase in LMA in GW needles could be interpreted as indirect evidence of an increase in carbohydrate concentration (Day and DeJong, 1990; Domec and Pruyn, 2008). The reduction of gS could also be attributed to the accumulation of ABA (Setter et al., 1980; Williams et al., 2000). We measured significant amounts of both ABA and its metabolite ABA-GE in needles, buds, xylem, and phloem above the girdle at the end of the experiment. We also expected GW plants to show higher levels of JA. However and despite the quick burst of JA in several species after mechanical injury (Wasternack, 2007) we did not find differences between treatments in any organ. Since we quantified hormones only at the end of the experiment, we could not discard the role of JA in stimulation of senescence and inhibiting root growth (Wasternack, 2007) in earlier stages, as previous works have reported that the signaling events mediated by this hormone occurs in a time window between a few minutes to several hours after wounding (León et al., 2001).
Abscisic acid, a well-known stress hormone, is produced in cells of stressed roots and may be delivered to the root apoplast and then transferred to the shoot via the transpiration stream (Sauter et al., 2002). It could have contributed to increased sink strength above the girdle (Yang et al., 2003) and to adjusting the physiological responses to wounding and decrease sink demand by regulating stomatal aperture (Setter et al., 1980). ABA synthesis is induced through the cleavage of carotenoid precursors (Nambara and Marion-Poll, 2005), thus lower values of water content and carotenoids in needles of GW plants 140 d.a.g. could also be the consequence of the increase in ABA in needles and play a role in leaf senescence (Schaper and Chacko, 1993; He and Jin, 1999). The reduction of leaf area after girdling was strengthened by the high levels of ABA found in buds which pointed to stronger bud dormancy in GW, preventing a new needle flush (Rinne et al., 1994). Soluble sugars above the girdle might have favored ABA-GE synthesis and accumulation in cell vacuoles (Sauter et al., 2002) regulating the ABA pool through glucosidase activity (Arve et al., 2013). Although the role of this metabolite is still unclear and it was initially reported as being physiologically inactive and functioning in storage and as a transport form of ABA (Jiang and Hartung, 2008), in P. canariensis free ABA was transported through xylem, and ABA-GE was found accumulated in significant amounts in needles of C, GF and GW plants, suggesting that this conjugate is trapped in the vacuoles and withdrawn from further metabolism (Sauter et al., 2002). In GW, the higher amount of ABA-GE in the phloem above the girdle than in the xylem or the phloem below the girdle suggested that leaves can release accumulated ABA-GE as a transport form to other tissues, such as buds, where β-glucosidase activity will release free active ABA (Yang and Zeevaart, 2006).
Girdling may affect sap transport in the xylem as a consequence of the concomitant reduction in leaf hydraulic conductance and stomatal closure (Domec and Pruyn, 2008; Sellin et al., 2013) and perhaps due to changes in the osmotic concentration of the xylem sap (Zwieniecki et al., 2004). In our experiment, we did not detect native xylem embolism in U or L or changes in KS. The reduction of leaf area in girdled trees with the increment of stem diameter in U resulted in higher efficiency of stem xylem in conducting water, measured as LSC, as occurs in dry-prone environments (López et al., 2013), but contrary to the reduction of stem hydraulic conductance capacity postulated in peach, a ring porous species, after girdling (Tombesi et al., 2014). In this sense, cambium removal early during the vegetative season affects less to tracheid bearing species than to ring porous species, dependent of the outermost part of the xylem to conduct water (Améglio et al., 2002). In contrast, the sharp decrease in root hydraulic conductance due to girdling reflected either changes in root development and morphology as a result of carbohydrate depletion or the inhibition of primary and lateral root elongation after the accumulation of cytokinins (Wan et al., 2006) caused by the decline in transpiration. Roots contain twice the concentration of starch early in the summer than later in the summer and therefore root respiration is less dependent on carbohydrate import in the early growing season (Högberg et al., 2001; Johnsen et al., 2007). Exhaustion of root reserves over time, intensified by the resprouting effort, and the suberization observed during stress, reduce root permeability (Steudle, 2000). In the same way, a reduction in nutrients decreases root conductance, as aquaporin activity is affected (Carvajal et al., 1996; Vandeleur et al., 2014). We found that, to a large degree, root conductance governs the maximum rate of transpiration that the plant is able to sustain. Root starvation after photosynthate withdrawal could therefore have decisively altered plant–water relations. The long-term response to the decrease in root hydraulic conductance would entail a lower moisture content of the stem and the progressive loss of parenchyma viability (Taylor and Cooper, 2002). Leaves of girdled plants were supplied with less water, measured as LScroot, and although transpiration was almost negligible at the end of the experiment in GW, leaf RWC was significantly lower in GW than in C and GF.
Gas Exchange and Damage to the Photosynthetic Apparatus
Downregulation of photosynthesis after girdling was consistent with other studies showing a negative feedback effect of carbohydrate accumulation on AN (DeJong, 1986; Day and DeJong, 1990; Myers et al., 1999; Iglesias et al., 2002; Urban and Alphonsout, 2007; Vemmos et al., 2012). The more rapid decline in AN than in gS from a gS lower than ca. 0.10 mol m-2 s-1 in P. canariensis (Figure 7A), and similar results observed in hybrid aspen (Sellin et al., 2013) and mango (Urban and Alphonsout, 2007), were attributed to the depressing effect of girdling on photosynthesis, primarily because of changes in the electron transport rate rather than changes in gS (Urban and Alphonsout, 2007). Two likely explanations previously proposed for this negative feedback on photosynthesis were an excess of starch grains leading to physical damage to thylakoids and the subsequent decrease of chlorophyll levels (Schaffer et al., 1986), and inhibition of photosynthetic genes regulated by carbohydrate content (Paul and Foyer, 2001). Girdling changed the concentration and ratio of photosynthetic pigments. Chlorophylls progressively decreased in girdled plants and we observed chlorosis in mature leaves early in the experiment. The effect was rapidly reversed when the phloem reconnected and only the chla:b ratio took longer to recover to the levels of control plants.
Actual efficiency of PSII, ΦPSII was affected by sink limitation earlier than by maximum photochemical efficiency (FV/FM). The reduction of ΦPSII pointed to a decrease of absorbed energy and also to loss of efficiency in energy processing. The chla:b ratio has been correlated with the ratio of PSII cores to light harvesting-protein complex (LHCII), in which the majority of chlb is located (Kitajima and Hogan, 2003). In girdled plants the chla:b ratio decreased suggesting a faster drop in light processing than light harvesting. Reduced energy utilization by CO2 assimilation, in combination with high energy capture, is potentially harmful and can result in over-reduction of the electron transport chain, photoinhibition and oxidative stress (Badger, 1985). The decreased observed in AN was correlated with a decrease in ΦPSII and an increase in harmless non-radiative energy dissipation that provided photoprotection from oxidative damage following sink limitation (Myers et al., 1999; Adams et al., 2005). As a result, FV/FM was higher than the optimum 0.83 (Maxwell and Johnson, 2000) in 61% of girdled plants 35 d.a.g. Partial recovery after reconnection of the phloem, with most plants showing FV/FM higher than 0.8 in our experiment, and earlier results after flowering (new sinks for assimilates) in mango trees, support the idea that the photosynthetic system was maintained in a highly photoprotected state in girdled branches or stems (Urban and Alphonsout, 2007).
Conclusion
Girdling affected both the downward carbon flow and the upward water flow. Blocking the root sink led to an increase in the increment in diameter growth above the girdle and the decrease below it, accompanied by profuse sprouting below the girdle that stopped after depletion of carbohydrates stored in the roots. Removing the sprouts may have jeopardized the plant strategy to recover from the damage, although the adaptation of this species to wounding allowed plants to survive more than 140 days without carbohydrate supply to the roots. The accumulation of carbohydrates in the aerial parts caused the reduction of whole plant transpiration due to leaf shedding and stomata closure due to feedback inhibition of photosynthesis and higher ABA and ABA-GE metabolism. Girdling strongly decreased root hydraulic conductance, whereas the water-conducting capacity of the stem increased above the girdle. Both ΦPSII and FV/FM decreased in girdled plants in comparison with controls. FV/FM values did not recover after wound healing, suggesting that phloem reconnection may still not be fully functional in some plants at the end of the experiment.
Author Contributions
LG provided the idea after observations in natural populations. RL, PP and LG designed the experiment. RL and PP performed the wounds and carried out measurements of growth, gas exchange, fluorescence and plant hydraulics. RB analyzed the hormones. All authors contributed to interpret the results. RL drafted the manuscript. All authors read and approved the final manuscript.
Conflict of Interest Statement
The authors declare that the research was conducted in the absence of any commercial or financial relationships that could be construed as a potential conflict of interest.
Acknowledgments
We are grateful to the Canary Islands Government and the Cabildos of Tenerife and Gran Canaria for longstanding support in the study of Canary Island pine. We thank Leonor Alegre and Marta Pintó-Marijuan for their assistance in quantifying hormones at the University of Barcelona. We also want to thank the two reviewers who improved with their comments and suggestions the manuscript. This study was funded by the Spanish Ministry of Science as part of the Project AGL2009-10606 (VULCAN) and by the National Parks Authority through the project SPIP2014-01093 (REPICAN).
References
Adams, W. W. III, Zarter, C. R., Mueh, K. E., Amiar, V. S. E., and Demmig-Adams, B. (2005). “Energy dissipation and photoinhibition: a continuum of photoprotection,” in Photoprotection, Photoinhibition, Gene Regulation and Environment, eds B. Demming-Adams, W. W. Adams III, and A. K. Mattoo (Berlin: Springer-Verlag), 49–64.
Améglio, T., Bodet, C., Lacointe, A., and Cochard, H. (2002). Winter embolism, mechanisms of xylem hydraulic conductivity recovery and springtime growth patterns in walnut and peach species. Tree Physiol. 22, 1211–1220. doi: 10.1093/treephys/22.17.1211
PubMed Abstract | Full Text | CrossRef Full Text | Google Scholar
Arve, L. E., Terfa, M. T., Gislerød, H. R., Olsen, J. E., and Torre, S. (2013). High relative air humidity and continuous light reduce stomata functionality by affecting the ABA regulation in rose leaves. Plant Cell Environ. 36, 382–392. doi: 10.1111/j.1365-3040.2012.02580.x
PubMed Abstract | Full Text | CrossRef Full Text | Google Scholar
Badger, M. R. (1985). Photosynthetic oxygen exchange. Ann. Rev. Plant Physiol. 36, 27–53. doi: 10.1146/annurev.pp.36.060185.000331
Bell, T. L., and Ojeda, F. (1999). Underground starch storage in Erica species of the Cape Floristic Region – differences between seeders and resprouters. New Phytol. 144, 143–152. doi: 10.1046/j.1469-8137.1999.00489.x
Bond, W. J., and Midgley, J. J. (2003). The evolutionary ecology of sprouting in woody plants. Int. J. Plant Sci. 164, S103–S114. doi: 10.1086/374191
PubMed Abstract | Full Text | CrossRef Full Text | Google Scholar
Brodribb, T. J., and Field, T. S. (2000). Stem hydraulic supply is linked to leaf photosynthetic capacity: evidence from New Caledonian and Tasmanian rainforests. Plant Cell Environ. 23, 1381–1388. doi: 10.1046/j.1365-3040.2000.00647.x
Brossa, R., López-Carbonell, M., Jubany-Marí, T., and Alegre, L. (2011). Interplay between Abscisic acid and jasmonic acid and its role in water-oxidative stress in wild-type, ABA-deficient, JA-deficient, and ascorbate-deficient Arabidopsis plants. J. Plant Growth Regul. 30, 322–333. doi: 10.1007/s00344-011-9194-z
Carvajal, M., Cooke, D. T., and Clarkson, D. T. (1996). Responses of wheat plants to nutrition deprivation may involve the regulation of water-channel function. Planta 199, 372–381. doi: 10.1007/BF00195729
Chano, V., López, R., Pita, P., Collada, C., and Soto, A. (2015). Proliferation of axial parenchymatic xylem cells is a key step in wound closure of girdled stems in Pinus canariensis. BMC Plant Biol. 15:64. doi: 10.1186/s12870-015-0447-z
PubMed Abstract | Full Text | CrossRef Full Text | Google Scholar
Christman, M. A., Sperry, J. S., and Smith, D. D. (2012). Rare pits, large vessels and extreme vulnerability to cavitation in a ringporous tree species. New Phytol. 193, 713–720. doi: 10.1111/j.1469-8137.2011.03984.x
PubMed Abstract | Full Text | CrossRef Full Text | Google Scholar
Climent, J., Tapias, R., Pardos, J. A., and Gil, L. (2004). Fire adaptations in the Canary Islands pine (Pinus canariensis). Plant Ecol. 171, 185–196. doi: 10.1023/B:VEGE.0000029374.64778.68
Collin, F., Sanjines, A., Fortin, M., Bontemps, J. D., and Nicolini, E. (2012). Fagus sylvatica trunk epicormics in relation to primary and secondary growth. Ann. Bot. 110, 995–1005. doi: 10.1093/aob/mcs178
PubMed Abstract | Full Text | CrossRef Full Text | Google Scholar
Daudet, F. A., Ameglio, T., Cochard, H., Archilla, O., and Lacointe, A. (2005). Experimental analysis of the role of water and carbon in tree stem diameter variations. J. Exp. Bot. 56, 135–144.
Day, K. R., and DeJong, T. M. (1990). Girdling of early season ‘Mayfire’ nectarine trees. J. Hortic. Sci. 65, 529–534.
DeJong, T. M. (1986). Fruit effects of photosynthesis in Prunus persica. Physiol. Plant. 66, 149–153. doi: 10.1111/j.1399-3054.1986.tb01248.x
Del Tredici, P. (2001). Sprouting in temperate trees: a morphological and ecological review. Bot. Rev. 67, 121–140. doi: 10.1007/BF02858075
De Schepper, V., Steppe, K., Van Labeke, M. C., and Lemeur, R. (2010). Detailed analysis of double girdling effects on stem diameter variations and sap flow in young oak trees. Environ. Exp. Bot. 68, 149–156. doi: 10.1016/j.envexpbot.2009.11.012
Di Vaio, C., Petito, A., and Buccheri, M. (2001). Effect of girdling on gas exchanges and leaf mineral content in the ‘Independence’ nectarine. J. Plant Nutr. 24, 1047–1060. doi: 10.1081/PLN-100103802
Domec, J. C., and Pruyn, M. L. (2008). Bole girdling affects metabolic properties and root, trunk and branch hydraulics of young ponderosa pine trees. Tree Physiol. 28, 1493–1504. doi: 10.1093/treephys/28.10.1493
PubMed Abstract | Full Text | CrossRef Full Text | Google Scholar
Frankis, M. (1999). Pinus brutia (Pinaceae). Curtis Bot. Mag. 16, 173–184. doi: 10.1111/1467-8748.00216
Fumuro, M. (1998). Effects of trunk girdling during early shoot elongation period on tree growth, mineral absorption, water stress, and root respiration in Japanese Persimmon (Diospyros kaki L.) cv. Nishimurawase. J. Jpn. Soc. Hortic. Sci. 67, 219–227. doi: 10.2503/jjshs.67.219
Genty, B., Briantais, J. M., and Baker, N. R. (1989). The relationship between quantum yield of photosynthetic electron transport and quenching of chlorophyll fluorescence. Biochim. Biophys. Acta 900, 87–92. doi: 10.1016/S0304-4165(89)80016-9
He, P., and Jin, J. N. (1999). Relationships among hormone changes, transmembrane flux of Ca2+ and lipid peroxidation during leaf senescing in spring maize. Acta Bot. Sin. 41, 1221–1225.
Högberg, P., Nordgren, A., Buchmann, N., Taylor, A. F., Ekblad, A., Högberg, M. N., et al. (2001). Large-scale forest girdling shows that current photosynthesis drives soil respiration. Nature 411, 789–792. doi: 10.1038/35081058
PubMed Abstract | Full Text | CrossRef Full Text | Google Scholar
Iglesias, D. J., Lliso, I., Tadeo, F. R., and Talon, M. (2002). Regulation of photosynthesis through source: sink imbalance in citrus is mediated by carbohydrate content in leaves. Physiol. Plant. 116, 563–572. doi: 10.1034/j.1399-3054.2002.1160416.x
PubMed Abstract | Full Text | CrossRef Full Text | Google Scholar
Jang, J. C., Leon, P., Zhou, L., and Sheen, J. (1997). Hexokinase as a sugar sensor in higher plants. Plant Cell 9, 5–19. doi: 10.1105/tpc.9.1.5
PubMed Abstract | Full Text | CrossRef Full Text | Google Scholar
Jang, J. C., and Sheen, J. (1994). Sugar sensing in higher plants. Plant Cell 6, 1665–1679. doi: 10.1105/tpc.6.11.1665
PubMed Abstract | Full Text | CrossRef Full Text | Google Scholar
Jiang, F., and Hartung, W. (2008). Long-distance signalling of abscisic acid (ABA): the factors regulating the intensity of the ABA signal. J. Exp. Bot. 59, 37–43. doi: 10.1093/jxb/erm127
PubMed Abstract | Full Text | CrossRef Full Text | Google Scholar
Johnsen, K., Maier, C., Sanchez, F., Anderson, P., Butnor, J., Waring, R., et al. (2007). Physiological girdling of pine trees via phloem chilling: proof of concept. Plant Cell Environ. 30, 128–134. doi: 10.1111/j.1365-3040.2006.01610.x
PubMed Abstract | Full Text | CrossRef Full Text | Google Scholar
Keeley, J. E., and Zedler, P. H. (1998). “Evolution of life histories in Pinus,” in Ecology and Biogeography of Pinus, ed. D. M. Richardson (Cambridge: Cambridge University Press), 219–249.
Kitajima, K., and Hogan, K. P. (2003). Increases of chlorophyll a/b ratios during acclimation of tropical woody seedlings to nitrogen limitation and high light. Plant Cell Environ. 26, 857–865. doi: 10.1046/j.1365-3040.2003.01017.x
PubMed Abstract | Full Text | CrossRef Full Text | Google Scholar
Kuwagata, T., Ishikawa-Sakurai, J., Hayashi, H., Nagasuga, K., Fukushi, K., Ahamed, A., et al. (2012). Influence of low air humidity and low root temperature on water uptake, growth and aquaporin expression in rice plants. Plant Cell Physiol. 53, 1418–1431. doi: 10.1093/pcp/pcs087
PubMed Abstract | Full Text | CrossRef Full Text | Google Scholar
Landhausser, S. M., and Lieffers, V. J. (2002). Leaf area renewal, root retention and carbohydrate reserves in a clonal tree species following above-ground disturbance. J. Ecol. 90, 658–665. doi: 10.1046/j.1365-2745.2002.00699.x
León, J., Rojo, E., and Sánchez-Serrano, J. J. (2001). Wound signalling in plants. J. Exp. Bot. 52, 1–9. doi: 10.1093/jexbot/52.354.1
López, R., López de Heredia, U., Collada, C., Cano, F. J., Emerson, B. C., Cochard, H., et al. (2013). Vulnerability to cavitation, hydraulic efficiency, growth and survival in an insular pine (Pinus canariensis). Ann. Bot. 111, 1167–1179. doi: 10.1093/aob/mct084
PubMed Abstract | Full Text | CrossRef Full Text | Google Scholar
Maxwell, K., and Johnson, G. N. (2000). Chlorophyll fluorescence–a practical guide. J. Exp. Bot. 51, 659–668. doi: 10.1093/jexbot/51.345.659
Münch, E. (1938). Investigations on the harmony of tree shape (trans. M. H. Zimmermann, 1963). Jahbr. Wiss. Bot. 86, 581–673.
Murakami, P. F., Schaberg, P. G., and Shane, J. B. (2008). Stem girdling manipulates leaf sugar concentrations and anthocyanin expression in sugar maple trees during autumn. Tree Physiol. 28, 1467–1473. doi: 10.1093/treephys/28.10.1467
PubMed Abstract | Full Text | CrossRef Full Text | Google Scholar
Myers, D., Thomas, R., and DeLucia, E. (1999). Photosynthetic responses of loblolly pine (Pinus taeda L.) needles to experimental sink demand reduction. Tree Physiol. 19, 235–242. doi: 10.1093/treephys/19.4-5.235
Nambara, E., and Marion-Poll, A. (2005). Abscisic acid biosynthesis and catabolism. Annu. Rev. Plant Biol. 56, 165–185. doi: 10.1146/annurev.arplant.56.032604.144046
PubMed Abstract | Full Text | CrossRef Full Text | Google Scholar
Parker, J. (1974). Effects of Defoliation, Girdling, and Severing of Sugar Maple Trees on Root Starch and Sugar Levels. Upper Darby, PA: U.S. Department of Agriculture, Forest Service, Research Paper NE-306, 4.
Paul, M. J., and Foyer, C. (2001). Sink regulation of photosynthesis. J. Exp. Bot. 360, 1383–1400. doi: 10.1093/jexbot/52.360.1383
Rinne, P., Tuominen, H., and Junttila, O. (1994). Seasonal changes in bud dormancy in relation to bud morphology, water and starch content, and abscisic acid concentration in adult trees of Betula pubescens. Tree Physiol. 14, 549–561. doi: 10.1093/treephys/14.6.549
PubMed Abstract | Full Text | CrossRef Full Text | Google Scholar
Rivas, F., Gravina, A., and Agustí, M. (2007). Girdling effects on fruit set and quantum yield efficiency of PSII in two Citrus cultivars. Tree Physiol. 27, 527–535. doi: 10.1093/treephys/27.4.527
PubMed Abstract | Full Text | CrossRef Full Text | Google Scholar
Rodríguez Martín, J. A., Nanos, N., Miranda, J. C., Carbonell, G., and Gil, L. (2013). Volcanic mercury in Pinus canariensis. Naturwissenschaften 100, 739–747. doi: 10.1007/s00114-013-1070-1
PubMed Abstract | Full Text | CrossRef Full Text | Google Scholar
Salleo, S., Lo Gullo, M. A., De Paoli, D., and Zippo, M. (1996). Xylem recovery from cavitation-induced embolism in young plants of Laurus nobilis: a possible mechanism. New Phytol. 132, 47–56. doi: 10.1111/j.1469-8137.1996.tb04507.x
Sauter, A., Dietz, K. J., and Hartung, W. (2002). A possible stress physiological role of abscisic acid conjugates in root-t-shoot signaling. Plant Cell Environ. 25, 223–228. doi: 10.1046/j.1365-3040.2002.00747.x
Schaffer, A. A., Liu, K. C., Goldschmidt, E. E., Boyer, C. D., and Goren, R. (1986). Citrus leaf chlorosis induced by sink removal- starch, nitrogen and chloroplast ultrastructure. J. Plant Physiol. 124, 111–121. doi: 10.1016/S0176-1617(86)80183-3
Schaper, H., and Chacko, E. K. (1993). Effect of irradiance, leaf age, chlorophyll content and branch-girdling on gas exchange of cashew (Anacardium occidentale L.). J. Hortic. Sci. 68, 541–550.
Schmuelling, T. (2002). New insights into the functions of cytokinins in plant development. J. Plant Growth Regul. 21, 40–49. doi: 10.1007/s003440010046
PubMed Abstract | Full Text | CrossRef Full Text | Google Scholar
Sellin, A., Niglas, A., Õunapuu, E., and Karusion, A. (2013). Impact of phloem girdling on leaf gas exchange and hydraulic conductance in hybrid aspen. Biol. Plant. 57, 531–539. doi: 10.1007/s10535-013-0316-2
Setter, T. L., Brun, W. A., and Brenner, M. L. (1980). Effect of obstructed translocation of leaf abscisic acid on associated stomatal closure and photosynthesis decline. Plant Physiol. 65, 1111–1115. doi: 10.1104/pp.65.6.1111
PubMed Abstract | Full Text | CrossRef Full Text | Google Scholar
Singh, B., Nordgren, A., Lofvenius, M. O., Hogberg, M. N., Mellander, P. E., and Hogberg, P. (2003). Tree root and soil heterotrophic respiration as revealed by girdling of boreal Scots pine forest: extending observations beyond the first year. Plant Cell Environ. 26, 1287–1296. doi: 10.1046/j.1365-3040.2003.01053.x
Steudle, E. (2000). Water uptake by plant roots: an integration of views. Plant Soil 226, 45–56. doi: 10.1023/A:1026439226716
Taylor, A., and Cooper, P. (2002). The effect of stem girdling on wood quality. Wood Fiber Sci. 34, 212–220.
Tombesi, S., Day, K. R., Johnson, R. S., Phene, R., and DeJong, T. M. (2014). Vigour reduction in girdled peach trees is related to lower midday stem water potentials. Funct. Plant Biol. 41, 1336–1341. doi: 10.1071/FP14089
Tyree, M. T., Patiño, S., Bennink, J., and Alexander, J. (1995). Dynamic measurements of roots hydraulic conductance using a high-pressure flowmeter in the laboratory and field. J. Exp. Bot. 46, 83–94. doi: 10.1093/jxb/46.1.83
Urban, L., and Alphonsout, L. (2007). Girdling decreases photosynthetic electron fluxes and induces sustained photoprotection in mango leaves. Tree Physiol. 27, 345–352. doi: 10.1093/treephys/27.3.345
PubMed Abstract | Full Text | CrossRef Full Text | Google Scholar
Vandeleur, R. K., Sullivan, W., Athman, A., Jordans, C., Gilliham, M., Kaiser, B. N., et al. (2014). Rapid shoot-to-root signaling regulates root hydraulic conductance via aquaporins. Plant Cell Environ. 37, 520–538. doi: 10.1111/pce.12175
PubMed Abstract | Full Text | CrossRef Full Text | Google Scholar
Vemmos, S. N., Pagagiannopoulou, A., and Coward, S. (2012). Effects of shoot girdling on photosynthetic capacity, leaf carbohydrate, and bud abscission in pistachio (Pistacia vera L.). Photosynthetica 50, 35–48. doi: 10.1007/s11099-012-0003-0
Wan, X. C., Landhäusser, S. M., Lieffers, V. J., and Zwiazek, J. J. (2006). Signals controlling root suckering and adventitious shoot formation in aspen (Populus tremuloides). Tree Physiol. 26, 681–687. doi: 10.1093/treephys/26.5.681
PubMed Abstract | Full Text | CrossRef Full Text | Google Scholar
Wasternack, C. (2007). Jasmonates: an update on biosynthesis, signal transduction and action in plant stress response, growth and development. Ann. Bot. 100, 681–697. doi: 10.1093/aob/mcm079
PubMed Abstract | Full Text | CrossRef Full Text | Google Scholar
Weaver, R. J., and McCune, S. B. (1959). Girdling: its relation to carbohydrate nutrition and development of Thompson Seedless, Red Malaga and Ribier grapes. Hilgardia 28, 421–456. doi: 10.3733/hilg.v28n16p421
Wellburn, A. R. (1994). The spectral determination of Chlorophylls a and b, as well as total carotenoids, using various solvents with spectrophotometers of different resolution. J. Plant Physiol. 144, 307–313. doi: 10.1016/S0176-1617(11)81192-2
Williams, L. E., and Ayars, J. E. (2005). Water use of Thompson Seedless grapevines as affected by the application of gibberellic acid (GA3) and trunk girdling – practices to increase berry size. Agric. Forest Meteorol. 129, 85–94. doi: 10.1016/j.agrformet.2004.11.007
Williams, L. E., Retzlaff, W. A., Yang, W., Biscay, P. J., and Ebisuda, N. (2000). The effect of girdling on leaf gas exchange, water status and non-structural carbohydrates of field-grown Vitis vinifera L. (cv. Flame Seedless). Am. J. Enol. Viticult. 51, 49–54.
Yang, J., Zhang, J., Wang, Z., and Zhu, Q. (2003). Hormones in the grains in relation to sink strength and postanthesis development of spikelets in rice. Plant Growth Regul. 41, 85–195. doi: 10.1023/B:GROW.0000007503.95391.38
Yang, S. H., and Zeevaart, J. A. D. (2006). Expression of ABA 8’-hydroxylases in relation to leaf water relations and seed development in bean. Plant J. 47, 675–686. doi: 10.1111/j.1365-313X.2006.02815.x
PubMed Abstract | Full Text | CrossRef Full Text | Google Scholar
Zhu, W. Z., Xiang, J. S., Wang, S. G., and Li, M. H. (2012). Resprouting ability and mobile carbohydrate reserves in an oak shrubland decline with increasing elevation on the eastern edge of the Qinghai-Tibet Plateau. Forest Ecol. Manage. 278, 118–126. doi: 10.1016/j.foreco.2012.04.032
Zwieniecki, M. A., and Holbrook, N. M. (1998). Diurnal variations in xylem hydraulic conductivity in white ash (Fraxinus americana L.), red maple (Acer rubrum L.) and red spruce (Picea rubens Sarg.). Plant Cell Environ. 21, 1173–1180. doi: 10.1046/j.1365-3040.1998.00342.x
Keywords: sprouting, source–sink regulation, gas exchange, chlorophyll fluorescence, hydraulic conductance, ABA
Citation: López R, Brossa R, Gil L and Pita P (2015) Stem girdling evidences a trade-off between cambial activity and sprouting and dramatically reduces plant transpiration due to feedback inhibition of photosynthesis and hormone signaling. Front. Plant Sci. 6:285. doi: 10.3389/fpls.2015.00285
Received: 24 February 2015; Accepted: 09 April 2015;
Published online: 29 April 2015
Edited by:
Boris Rewald, University of Natural Resources and Life Sciences, Vienna, AustriaReviewed by:
Sergio Tombesi, University of Perugia, ItalyGreg Moore, The University of Melbourne, Australia
Copyright © 2015 López, Brossa, Gil and Pita. This is an open-access article distributed under the terms of the Creative Commons Attribution License (CC BY). The use, distribution or reproduction in other forums is permitted, provided the original author(s) or licensor are credited and that the original publication in this journal is cited, in accordance with accepted academic practice. No use, distribution or reproduction is permitted which does not comply with these terms.
*Correspondence: Rosana López, Forest Genetics and Physiology Research Group, School of Forest Engineering, Technical University of Madrid, Ciudad Universitaria s/n, 28040 Madrid, Spain,cm9zYW5hLmxvcGV6QHVwbS5lcw==