- 1Forage Seed Lab, China Agricultural University, Beijing, China
- 2Beijing Key Laboratory of Grassland Science, Beijing, China
- 3Department of Plant Sciences, University of California at Davis, Davis, California, CA, USA
To evaluate deterioration of oat seeds during storage, we analyzed oxygen radicals, antioxidant enzyme activity, proline content, and gene transcript levels in oat seeds with different moisture contents (MCs; 4, 16, and 28% w/w) during storage for 0, 6, and 12 months (CK, LT-6, and LT-12 treatments, respectively) at 4°C. The germination percentage decreased significantly with higher seed MCs and longer storage duration. The concentrations of superoxide radical and hydrogen peroxide increased with seed MC increasing. The activities of catalase (CAT), ascorbate peroxidase (APX), and superoxide dismutase (SOD) may have had a complementary or interacting role to scavenge reactive oxygen species. As the storage duration extended, the proline content decreased in seeds with 4 and 16% MC and increased in 28%. These findings suggest that proline played the main role in adaptation to oxidative stress in seeds with higher MC (28%), while antioxidant enzymes played the main role in seeds with lower MCs (4%, 16%). In the gene transcript analyses, SOD1 transcript levels were not consistent with total SOD activity. The transcript levels of APX1 and CAT1 showed similar trends to those of APX and CAT activity. The transcript levels of P5CS1, which encodes a proline biosynthetic enzyme, increased with seed MC increasing in CK. Compared with changing of proline content in seeds stored 12 months, PDH1 transcript levels showed the opposite trend and maintained the lower levels in seeds of 16 and 28% MCs. The transcript level of P5CS1 was significantly affected by MC, and PDH1 could improve stress resistance for seed aging and maintain seed vigor during long-term storage.
Introduction
Deterioration of seeds is a major problem in agricultural production. Seed deterioration depends on the temperature, seed moisture content (MC), and duration of storage (Priestley, 1986; Spanò et al., 2004). The vigor of seeds is reduced or lost during long-term storage, even as stored under low-temperature and low-moisture conditions. The reduction in seed vigor leads to commercial losses and decreased genetic diversity (Lu et al., 2005). Seed aging is associated with certain changes in cellular metabolism and biochemistry, including lipid peroxidation, enzyme inactivation, disruption of membrane integrity, and damage to DNA (McDonald, 1999; Hu et al., 2012). Although the exact mechanisms of seed aging are unknown, the accumulation of reactive oxygen species (ROS), including the superoxide radical () and hydrogen peroxide (H2O2), has been suggested to be the major cause of seed deterioration (Lehner et al., 2008; Yao et al., 2012). It has been hypothesized that seeds germinate completely only when the ROS content is maintained below a critical threshold (Bailly et al., 2008). Malondialdehyde (MDA) is a product of ROS, and is a biomarker of oxidative damage (Bailly et al., 1996). To minimize the damaging effects of ROS, antioxidant enzymes such as superoxide dismutase (SOD), catalase (CAT), and ascorbate peroxidase (APX) scavenge ROS (Moller et al., 2007). The amount of ROS is linked to the rate of their production and the capacity of the antioxidant system (Esfandiari et al., 2007). Overexpression of genes encoding SOD, APX, and CAT in tobacco, wheat, and Arabidopsis resulted in enhanced seed longevity (Melchiorre et al., 2009; Li and Yi, 2012). Proline has also been shown to scavenge ROS (Smirnoff and Cumbes, 1989). In many plant species, proline accumulation is one of the main metabolic responses to abiotic stress (Evers et al., 2010; Irina et al., 2012). Early studies on proline established a model whereby stress caused the transcriptional up-regulation of the gene encoding Δ-1-pyrroline-5-carboxylate synthetase1 (P5CS1), which catalyzes the first two steps of proline biosynthesis (Armengaud et al., 2004), and down-regulation of the gene encoding proline dehydrogenase (PDH1), which catalyzes the first step of proline catabolism (Kiyosue et al., 1996; Miller et al., 2005). Both genes were necessary and sufficient for stress-induced proline accumulation.
Oat (Avena sativa L.) is the fifth largest cereal crop in the world, with an annual yield of approximately 700 000 tons. Compared with other cereals, oat seed has higher concentrations of soluble fiber, vitamins, minerals, antioxidants, and high quality protein. Therefore, it is an important cereal in terms of its nutritional value (Klose and Arendt, 2012). In recent years, oat has been cultivated more widely, and has become an important forage grass in alpine regions where other grasses cannot grow. The lager deterioration occurs in oat seeds with 10 years storage (Magdalena et al., 1999). The oat seed deterioration results in greater losses in seed vigor, causing great economic losses (Price, 1975; Heneen et al., 2008). The oat seeds have higher lipid content than other cereals such as wheat, maize, rice, and barley. This high lipid content results in faster deterioration of oat seeds (Pekka et al., 2003). The seed MC is another key factor affecting seed vigor during storage. The germinability lost in untreated oat seed was found to depend on its temperature and water content at storage condition (Machacer et al., 1961; Kong et al., 2014). However, the physiological and transcriptional changes in oat seeds with different MCs during storage are unknown.
In this study, we examined the dynamics of ROS, antioxidant enzyme activity, proline content, and gene transcript levels of APX1, CAT1, SOD1, P5CS1, and PDH1 in deteriorated oat seeds with different MCs during storage. The aims were to investigate whether storage treatments at low temperature (4°C) affected ROS levels via its effects on the enzymatic system and proline content, and to evaluate the transcript level of genes encoding antioxidant enzymes during seed aging.
Materials and Methods
Plant Material
Oat seeds (Lot#P708O2498) were purchased from the Lockwood Seed and Grain Company (Woodland, CA, USA). The experiments were initiated in May, 2012. The seeds were initially at 8.8% MC and germinated 98% (ISTA, 2012).
Determination of Seed MC
Seed MC was measured according to the ISTA Procedure (2012). Briefly, approximately 4.5 g seeds were placed in a sample container, weighed, dried at 130–133°C for 1 h, and then reweighed. Four replicates were evaluated for each sample.
Adjustment of Seed MC
To adjust the MC of the oat seeds to 4, 16, and 28% (w/w) before storage, seeds (~25 g) with 8.8% MC were placed in an aluminum foil bag and then subjected to rehydration or dehydration to achieve the desired water content. The seed MCs were adjusted to 16 and 28% by adding appropriate amounts of distilled water into the foil bags and incubating the seeds at 5°C for 48 h. The seeds were adjusted to 4% water content by desiccation.
Storage and Germination Assay
Seeds with different MCs were stored at 4°C for 0 months (CK), 6 months (LT-6), and 12 months (LT-12). The seed germination percentage was determined by standard germination tests according to the ISTA protocol (ISTA, 2012). Four replicates of 100 seeds each were germinated in 150-mm Petri dishes on filter paper hydrated with 14 ml water. Germination tests were conducted in a growth chamber (Bio Chamber-Enconair, Winnipeg, MB, Canada) at 20°C under an 8-h light/16-h dark photoperiod. The number of normal seedlings was recorded after 10 days. The seed germination percentage was expressed as the percentage of normal seedlings determined as described in the ISTA protocol (ISTA, 2012).
Determination of Superoxide Anion () Production Rate
The production rate was measured as described elsewhere (Elstner and Heupel, 1976). Seed embryos (1 g) were ground in liquid nitrogen, homogenized in 7 ml phosphate buffer (50 mM, pH 7.8), and then centrifuged at 16 000 rpm for 10 min. The supernatant was centrifuged again. Then, 1 ml supernatant was mixed with 0.9 ml phosphate buffer (50 mM) and 0.1 ml hydroxylamine hydrochloride. The mixture was incubated at 25°C for 30 min, and then mixed with 1 ml sulfanilic acid (17 mM) and 1 ml α-naphthylamine (7 mM); the mixture was incubated at 25°C for 20 min and then the absorbance at 530 nm was recorded.
Determination of Hydrogen Peroxide (H2O2) Content
To measure the H2O2 content, embryos (200 mg) were ground in liquid nitrogen, homogenized in 2.0 ml cold acetone, and then centrifuged at 16 000 rpm for 10 min. The supernatant (1.0 ml) was mixed with 100 μl 10% (w/v) titanium tetrachloride and 200 μl ammonia water, and then mixed well. The mixture was centrifuged at 3000 rpm for 10 min, and the supernatant was discarded. The pellet was dissolved in concentrated sulfuric acid, and then absorbance at 415 nm was recorded. A standard curve was prepared by diluting a 100 μmol/L H2O2 stock solution to 10, 20, 40, 60, 80, 100 μmol/L.
Determination of Antioxidant Enzyme Activities
To extract enzymes, embryos (200 mg) were ground in liquid nitrogen, homogenized in 2 ml phosphate buffer (50 mM, pH 7.0), and then centrifuged at 15 000 rpm for 20 min at 4°C. The supernatant was used in the antioxidant enzyme assays.
The activity of SOD was determined according to the method of (Beaucham and Fridovic, 1971). The 3 ml reaction mixture contained 13 mM methionine, 1.3 μM riboflavin, 63 μM nitroblue tetrazolium (NBT) in 50 mM phosphate buffer (pH 7.8), and 25 μl enzyme extract. The enzyme extract was replaced with phosphate buffer in two controls. The reaction mixtures were incubated in a growth chamber (LRH-250-GII, Ningbo, China) at 25°C under illumination. Identical tubes that were not illuminated served as blanks. After illumination for 17 min, absorbance was measured at 560 nm.
The activity of APX was measured in the presence of 0.25 mM ascorbic acid and 10 mM H2O2 by monitoring the decrease in absorption at 290 nm. The reaction mixture consisted of 200 μl supernatant, 3.4 ml phosphate buffer, 200 μl ascorbic acid, and 200 μl H2O2. Enzyme activity was determined by measuring the change in absorbance at 290 nm every minute.
To measure CAT activity, 50 μl supernatant was mixed with 3.4 ml phosphate buffer (25 mM, pH 7.0, containing 0.1 mM EDTA), and 200 μl H2O2. Enzyme activity was determined by measuring the change in absorbance at 240 nm after 1 min.
Determination of Proline Content
To measure proline content, embryos (200 mg) were ground in liquid nitrogen, homogenized in 2 ml 3% sulfosalicylic acid, and then centrifuged at 4 000 rpm for 5 min. The supernatant (200 μl) was mixed with 200 μl glacial acetic acid and 200 μl acidic ninhydrin, and then mixed well. The mixture was incubated at 100°C for 60 min. The reaction was terminated by placing the mixture on ice, and then extracting the sample with 1.0 ml toluene. The absorbance of the mixture was measured at 520 nm. To prepare the standard curve, an L-proline stock solution (100 μg/ml) was diluted to 1, 3, 5, 7, 9, 11 μg/ml.
Gene Transcript Analyses
Total RNA was isolated from seed embryos using the phenol–chloroform method (Cooley et al., 1999). Briefly, seed embryos (100 mg) were ground in liquid nitrogen and homogenized in 2 ml RNA extraction buffer (15 ml TLE buffer, 15 ml phenol, 3 ml chloroform) with 30 μl β-mercaptoethanol. The mixture was shaken for 30 min at 300 rpm at 4°C, and then centrifuged at 10 000 rpm for 2 min. The supernatant was collected and mixed with an equal volume of phenol–chloroform–isoamylalcohol (25:24:1), then shaken for 10 min at 4°C and centrifuged for 2 min at 10 000 rpm at 4°C. The supernatant was collected and mixed with an equal volume of chloroform–isoamyl alcohol (24:1), then shaken for 10 min at 4°C and centrifuged for 5 min at 10 000 rpm at 4°C. The supernatant was collected and mixed with a one-third volume of 8 M LiCl, mixed thoroughly, and then incubated at –20°C overnight. The next day, the sample was thawed and then centrifuged at 10 000 rpm for 3 min. The pellet was washed with 1.0 ml 80% (v/v) ethanol. The RNA was dried completely, and then dissolved in 50 μl diethyl pyrocarbonate water. The RNA quality was assessed by 1% agarose gel electrophoresis.
Candidate and reference gene sequences corresponding to the top BLAST hits were identified at the Compositae Genome Project EST database based on sequence homology to known candidates in Arabidopsis, and from existing oat sequence data in GenBank. Primer sequences for qRT-PCR analyses were designed using Primer Express (Applied Biosystems, Foster City, CA, USA). The genes and primers used for qRT-PCR analyses are shown in Table 1.
Total RNA was reverse-transcribed and DNase-treated using a QuantiTect Reverse Transcription Kit (Qiagen, Hilden, Germany). The cDNAs of housekeeping genes and genes of interest were PCR-amplified with an Applied Biosystems 7300 Real-Time PCR System using SYBR Green detection. For each sample, the change in fluorescence was analyzed using DART PCR 1.0 software.
Statistical Analyses
Data were subjected to analysis of variance (ANOVA) using SPSS for Windows ver. 13.0. Duncan’s multiple range tests (P = 0.05) were used to compare treatment means of germination and physiological indicators.
Results
Changes in Germination Percentage in Oat Seeds during Storage
The germination percentage of oat seeds decreased with seed MCs increasing (Figure 1). In the storage treatments of CK and LT-6, the germination percentage decreased significantly (P< 0.05) at 28% MC. In LT-12, the germination percentage decreased significantly (P < 0.05) with MC increasing from 4 to 28%.
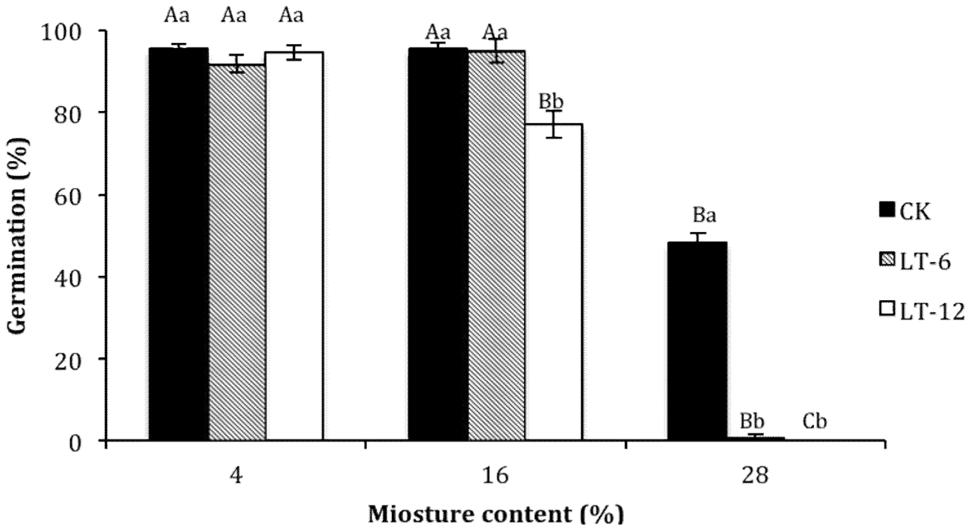
FIGURE 1. Effect of storage duration on germination percentage of oat seed with different moisture content (MC) at 4°C. The different letters indicate significant differences at 0.05 level among treatments as determined by the Duncan’s multiple range test. Means with different capital letters indicate the significant differences of seed at different MC and same storage duration, and with different lowercase letters at the same MC and different storage duration. Data represent the mean ± SD of four or more replicates.
The germination percentage of oat seeds with 4% MC did not change significantly (P > 0.05) during storage (Figure 1). At 16% MC, the germination percentage of seeds in LT-12 decreased significantly (P < 0.05). The germination percentage of seeds in LT-6 and LT-12 was zero at 28% MC (Figure 1).
Changes in Superoxide Anion () Production Rate in Oat Seeds during Storage
In the storage treatments of CK, the production rate increased significantly (P < 0.05) at 28% MC. The production rate in the LT-6 treatment increased significantly (P < 0.05) with MC increasing, but not in the LT-12 treatment (Figure 2A).
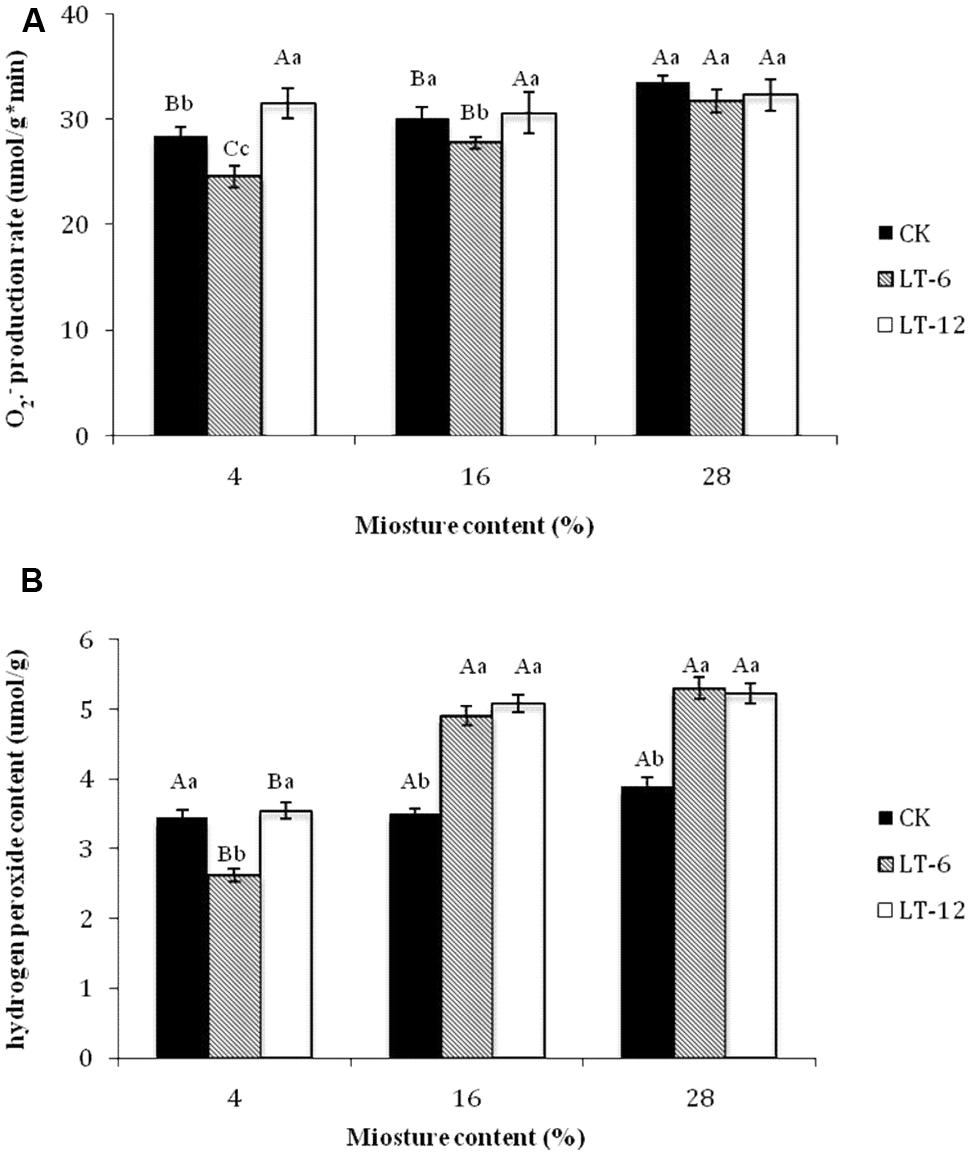
FIGURE 2. Effect of storage duration on production rate and H2O2 content of oat seed with different MC at 4°C. (A) production rate; (B) H2O2 content. The different letters indicate significant differences at 0.05 level among treatments as determined by the Duncan’s multiple range test. Means with different capital letters indicate the significant differences of seed at different MC and same storage duration, and with different lowercase letters at the same MC and different storage duration. Data represent the mean ± SD of four or more replicates.
In the seeds with 4 and 16% MC, the production rate decreased and then increased during storage; the lowest rate was after the stored 6 months (Figure 2A). For seeds with 28% MC, there were no significant (P > 0.05) differences for production rate among CK, LT-6, and LT-12 treatments.
Changes in Hydrogen Peroxide (H2O2) Contents in Oat Seeds during Storage
In the storage treatments of CK, there was not significantly (P > 0.05) different for H2O2 content of oat seeds among different MCs (Figure 2B). In the LT-6 and LT-12 treatments, the H2O2 contents of seeds with 16 and 28% MC were significantly higher (P < 0.05) than those with 4% MC. For seeds with 4% MC, the lowest of H2O2 content presented after stored 6 months. For 16 and 28% MCs, the H2O2 content in LT-6 and LT-12 were significantly higher (P < 0.05) than those in CK (Figure 2B).
Changes in Activities of Antioxidant Enzymes in Oat Seeds during Storage
In the storage treatments of CK, there was no significant difference (P > 0.05) for SOD activity of seeds among different MC (Figure 3A). In LT-6 and LT-12, the SOD activity significantly (P < 0.05) decreased with MC increasing from 4 to 28%. For seeds with 4% MC, SOD activities in CK and LT-12 were significantly (P < 0.05) lower than that in LT-6. For seeds of 16% MC, there were no significant (P < 0.05) differences for SOD activity among storage treatments. For seeds with 28% MC, SOD activity in LT-6 or LT-12 was significantly (P < 0.05) lower than that in CK.
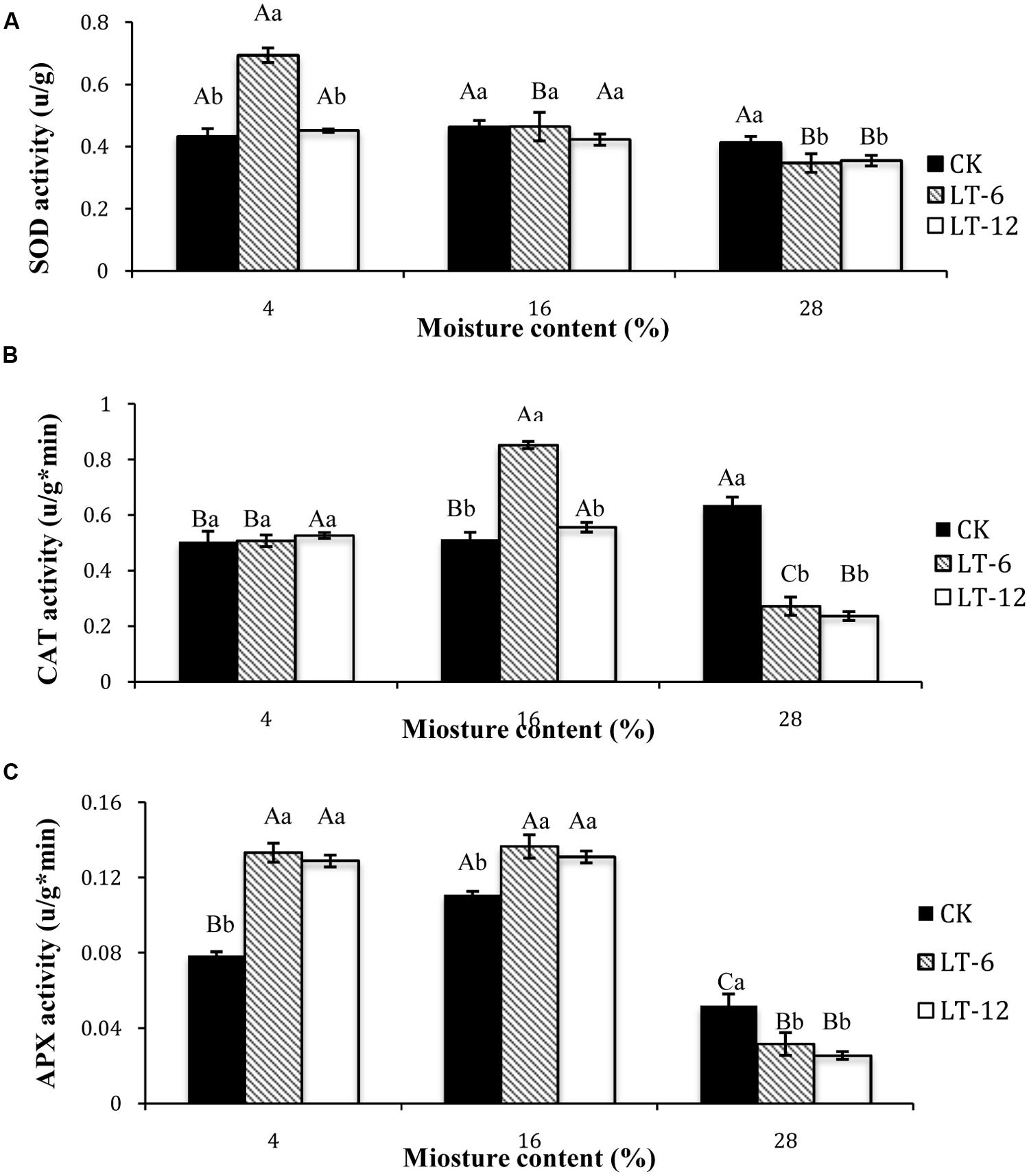
FIGURE 3. Effect of storage duration on SOD, CAT, and APX activity of oat seed with different MC at 4°C. (A) SOD; (B) CAT; (C) APX. The different letters indicate significant differences at 0.05 level among treatments as determined by the Duncan’s multiple range test. Means with different capital letters indicate the significant differences of seed at different MC and same storage duration, and with different lowercase letters at the same MC and different storage duration. Data represent the mean ± SD of four or more replicates.
In the storage treatments of CK, the CAT activity of seeds with 28% MC was significantly (P < 0.05) higher than that with 4 or 16%. In LT-6, the highest CAT activity occurred in seeds with 16% MC, and the lowest was in 28% MC. In LT-12, CAT activity in seeds of 28% MC decreased significantly (P < 0.05). For 4% moisture seeds, there were no significant (P > 0.05) differences for CAT activity among CK, LT-6, and LT-12. For 16% moisture seeds, the maximum CAT activity appeared after stored 6 months. For 28% moisture seeds, CAT activity in CK was significantly (P < 0.05) higher than other treatments (Figure 3B).
The changes of APX activity in oat seeds during storage showed that the highest APX activity in CK occurred in 16% moisture seeds, and the lowest was in those with 28% MC (Figure 3C). In LT-6 and LT-12, APX activities decreased significantly (P < 0.05) in 28% moisture seeds. For seeds with 4 and 16% MC, APX activities in LT-6 and LT-12 were significantly higher (P < 0.05) than that in CK. In seeds with 28% MC, changing of APX activity was opposite (Figure 3C).
Changes in Transcript Levels of Genes Encoding Antioxidant Enzymes in Oat Seeds During Storage
According to the results of transcript levels in oat seeds with different MC (Figure 4A), the SOD1 transcript level in CK was lowest in 4% moisture seeds. In LT-6, the highest SOD1 transcript level presented in seeds of 16% MC. In LT-12, the SOD1 transcript level were down-regulated significantly (P < 0.05) with MC increased from 4% to 28%. For seeds with 4% MC, SOD1 in LT-6 was down-regulated and up-regulated in LT-12. In seeds with 16 and 28% MC, the levels of SOD1 were down-regulated as the storage duration prolonged.
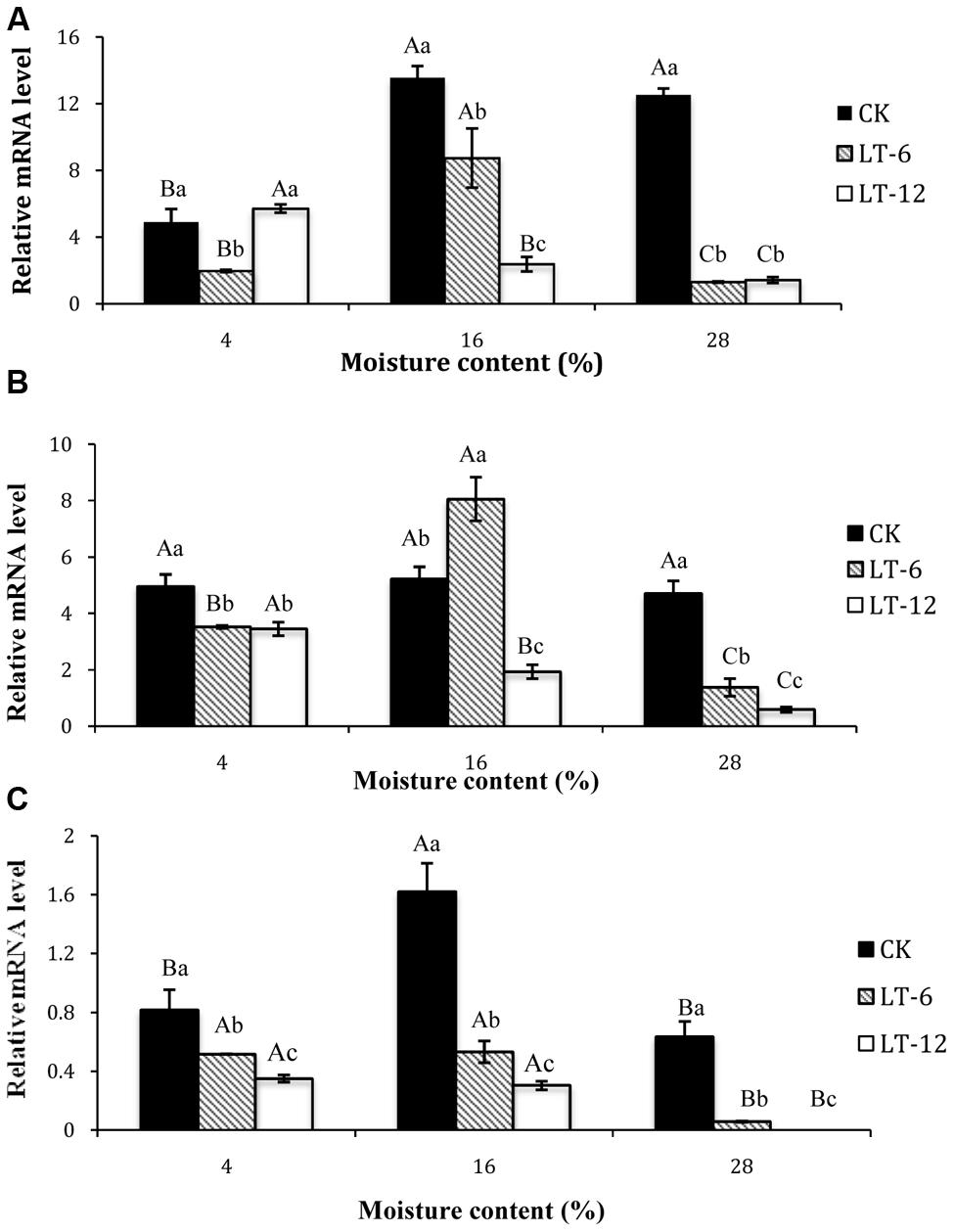
FIGURE 4. Effect of storage duration on SOD1, CAT1 and APX1 gene expression of oat seeds with different MC at 4°C. (A) SOD1; (B) CAT1; (C) APX1. The different letters indicate significant differences at 0.05 level among treatments as determined by the Duncan’s multiple range test. Means with different capital letters indicate the significant differences of seed at different MC and same storage duration, and with different lowercase letters at the same MC and different storage duration. Data represent the mean ± SD of three or more replicates.
The CAT1 transcript levels of seeds were similar among different MC in CK (Figure 4B). In LT-6 and LT-12, the changing of CAT1 transcript level was similar with SOD1. The CAT1 transcript levels tended to decrease with increasing storage duration in seeds with 4 and 28% MCs (Figure 4B). The transcript level of CAT1 in seeds with 16% MC was up-regulated in LT-6, but down-regulated in LT-12.
The changes of APX1 indicated that the highest APX1 level in CK occurred in seeds of 16% MC, and the lowest was of 28% MC. The transcript levels of APX1 in seeds with 4, 16, and 28% MC all decreased significantly (P < 0.05) during storage (Figure 4C).
Changes in Proline Contents in Oat Seeds during Storage
The proline contents in LT-6 and LT-12 increased significantly (P < 0.05) with seed MC increasing from 4 to 28% (Figure 5). In CK, the proline contents of seeds with 4% MC was significantly (P < 0.05) lower than that with 16 and 28% MC. For seeds with 4 and 16% MC, there were no significant (P > 0.05) differences in proline content between LT-6 and LT-12, but both showed significantly (P < 0.05) lower proline contents than those in CK (Figure 5). For seeds with 28% MC, the proline content increased significantly (P < 0.05) with storage duration prolonged.
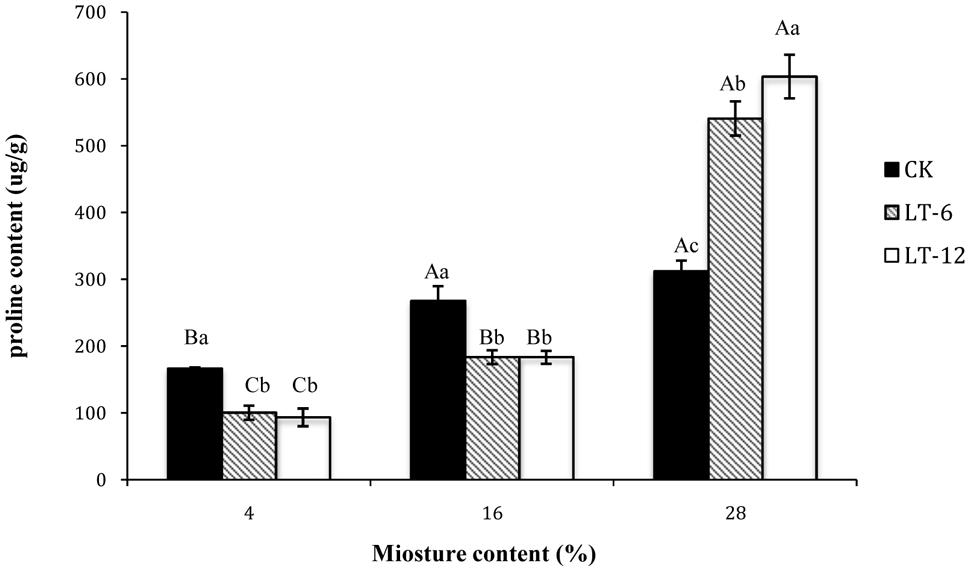
FIGURE 5. Effect of storage duration on proline content of oat seed with different MC at 4°C. The different letters indicate significant differences at 0.05 level among treatments as determined by the Duncan’s multiple range test. Means with different capital letters indicate the significant differences of seed at different MC and same storage duration, and with different lowercase letters at the same MC and different storage duration. Data represent the mean ± SD of four or more replicates.
Changes in Transcript Levels of Genes Related to Proline Biosynthesis and Catabolism during Storage
In the storage treatments of CK, the transcript level of P5CS1 increased significantly (P < 0.05) with seed MC increasing from 4 to 28% (Figure 6A). In LT-6 and LT-12, the highest transcript level of P5CS1 occurred in seeds of 16% MC. There was no significant (P > 0.05) differences for P5CS1 transcript levels in seeds with 4% MC during storage. For seeds with 16% MC, the transcript levels of P5CS1 in LT-6 and LT-12 were higher than that in CK. For seeds with 28% MC, the transcript levels of P5CS1 reached the minimum level after stored 12 months.
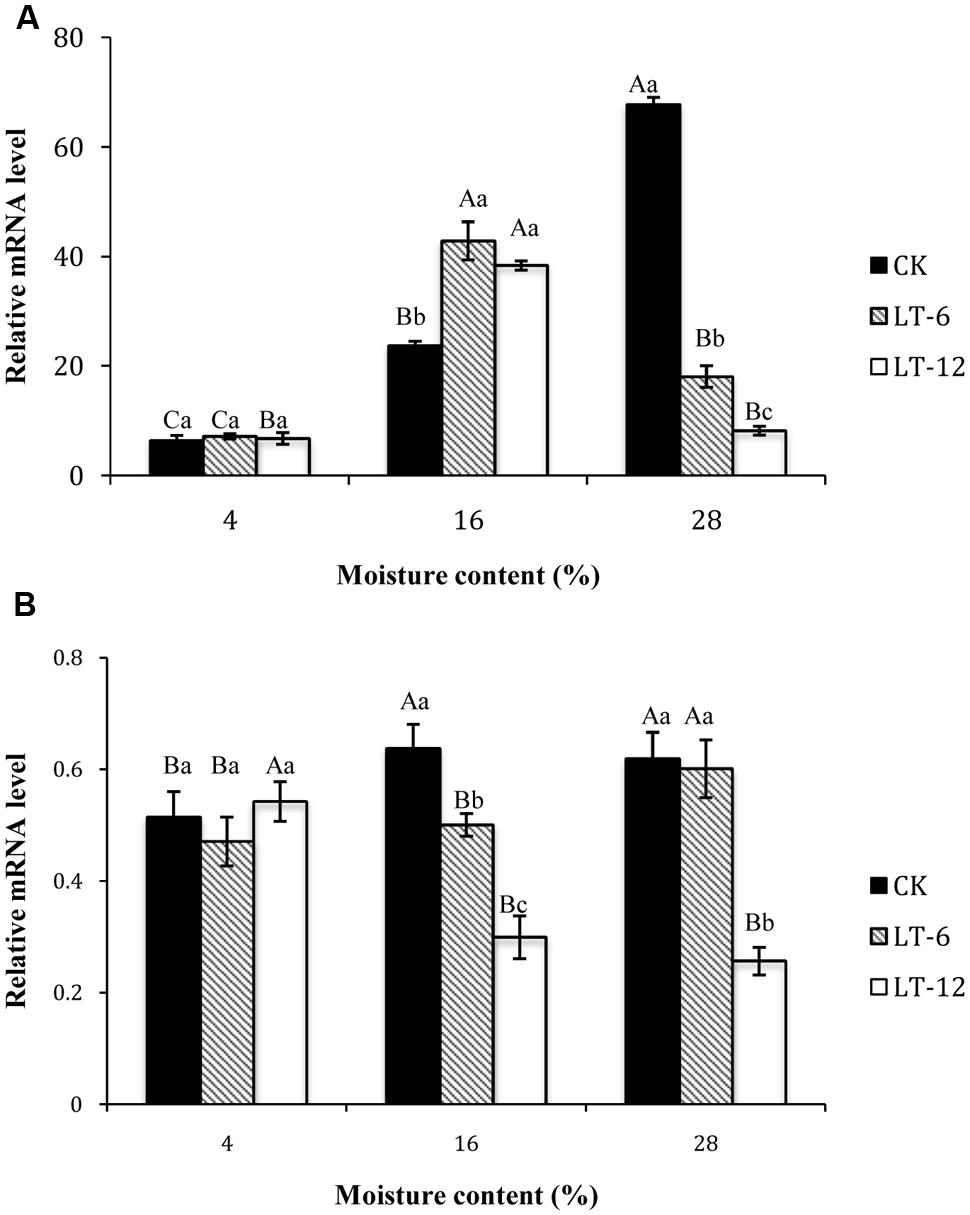
FIGURE 6. Effect of storage duration on P5CS1 and PDH1 gene expression of oat seeds with different MC at 4°C. (A) P5CS1; (B) PDH1. The different letters indicate significant differences at 0.05 level among treatments as determined by the Duncan’s multiple range test. Means with different capital letters indicate the significant differences of seed at different MC and same storage duration, and with different lowercase letters at the same MC and different storage duration. Data represent the mean ± SD of three or more replicates.
The changing of transcript levels of PDH1 during seed storage indicated that PDH1 levels in CK and LT-6 both increased with MC increasing from 4 to 28% (Figure 6B), but there was opposite changing for the transcript levels of PDH1 in LT-12. For seeds with 4% MC, there were no significant (P > 0.05) differences for transcript levels of PDH1 among three storage treatments. For seeds with 28% MC, the transcript levels of PDH1 decreased with storage duration prolonged, and reached the minimum after stored 12 months.
Discussion
Seed MC and storage duration greatly affect seed longevity during storage (Abba and Lovato, 1999; Mira et al., 2015). In this study, the germination percentage of oat seeds with 28% MC decreased significantly during storage (Figure 1). As the storing from 0 to 12 months, there was no significant difference for germination of oat seeds with 4% MC, but seeds with 28% MC did not germinate well, even in the CK. These findings suggested that low MC might be more important than the storage duration in terms maintaining the vigor of stored seeds. Similar results have been reported for seeds of many species under hermetic storage (Ellis et al., 1988; Zhang et al., 2010). The optimum MC for oat seeds may be 4%, but 16% MC was acceptable for seeds stored for less than 6 months.
The loss of seed germinability has been attributed to the accumulation of ROS (Wojtyla et al., 2006). Many reports have indicated that oxidative stress imposed by ROS is an important cause of seed deterioration during aging (McDonald, 1999; Rajjou and Debeaujon, 2008). In our study, the reduction of seed vigor was related to higher levels of ROS, such as and H2O2, as seed MC increased from 4 to 28% (Figure 2). A loss of seed viability was shown to be positively correlated with increasing MC in seeds of sunflower, beech (Fagus sylvatica), holm oak (Quercus ilex L.) and cotton (Gossypium hirsutum L.; Bailly et al., 1996; Pukacka and Ratajczak, 2005; Kibinza et al., 2006). Higher MCs in aging seeds resulted in greater oxidative damage and ROS generation. In this study, as the storage duration extended from 0 to 12 months, the production rate remained stable in seeds with 28% MC. The lowest production rate in seeds with 4 and 16% MC was attained after 6 months of storage (Figure 2A). The H2O2 content showed a similar trend; that is, the minimum level was in seeds with 4% MC after 6 months of storage (Figure 2B). These differences in H2O2 contents might be related with the level of MC. However, antioxidant enzymes could be activated after 6 months storage or the contents of non-enzymatic antioxidants had increased in seeds with 4 and 16% MC. This result suggested that seeds with lower MCs were able to repair the deteriorated damages at 4°C and storage of 6 months.
Antioxidant enzymes and non-enzymatic antioxidants, including SOD, CAT, APX, and proline, contribute to reduce the concentration of ROS (Kishor et al., 1995; Mittal et al., 2012; Oliveira et al., 2012). SOD is one of the most important enzymes in cellular defense against ROS, as it could catalyze the into H2O2 (Kumar et al., 2010; Kumara et al., 2010). CAT and APX have been demonstrated to scavenge the H2O2 produced by interacting under oxidative stress (Erdal et al., 2011; Yin et al., 2014). CAT reduces H2O2 to water and dioxygen, and APX reduces H2O2 to water and generates MDA (Noctor and Foyer, 1998). Activities of antioxidant enzymes have been observed to decrease in aged soybean, cotton, and sunflower (Bailly et al., 1996; Murthy et al., 2002; Goel et al., 2003). Increasing of MC promotes enzymatic oxidation and leads to rapid cellular damage, which decreases seed vigor (Gill and Tuteja, 2010). In this study, the changing of SOD activity was related with the level of MC and storage duration. There were no significant changes in CK with MC increasing, however, SOD activity decreased with MC increasing as stored 6 and 12 months (Figure 3A). There was different changing tendency between CAT and APX activity with MC increasing and storage duration prolonged. CAT and APX could respond rapidly to scavenge H2O2 under stress. CAT activity was more responsive to the variation in higher MC compared to APX. The highest SOD activity in seeds with 4% MC occurred after 6 months of storage, but the production rate and H2O2 content reached the lowest level. Furthermore, the different changing for CAT and APX activity under moisture of 4% indicated that APX was more responsive than CAT to oxidative damage as the storage duration extended. These two enzymes may have complementary or interacting roles. The activities of SOD, CAT, and APX in seeds with 28% MC showed similar trends. The reductions in SOD, CAT, and APX activities may be because of ROS accumulated to toxic levels (Kong et al., 2014) or intolerant of the higher MC and/or long-term storage.
The accumulation of low-molecular weight metabolites acting as osmoprotectants, such as proline, is part of the adaptive response to stress in plants (Hoekstra et al., 2001). Kishor and Dange (1990) reported that proline protected cellular functions by scavenging ROS. In our study, proline content tended to increase with seed MC increasing. It’s illustrated that proline accumulation in seeds could confer some adaptive advantages under stress. However, an increasing in proline content could also be considered as a stress-induced marker for oxidative damage during aging (Lei and Chang, 2012). As seeds storing 12 months, the proline contents decreased in seeds with 4 and 16% MC, but increased in seeds with 28% MC (Figure 5). Meanwhile, antioxidant enzyme activity also tended to increase in seeds with 4 and 16% MC, but decrease in those with 28% content MC during storage (Figure 3). This result suggested that proline played a more important role in adapting to oxidative stress in seeds with higher MC (28%), while antioxidant enzymes played a more important role in seeds with lower MCs (4 and 16%).
To elucidate the role of the ROS scavenging system during seed aging, we analyzed the transcript levels of genes encoding antioxidant enzymes by real-time PCR. The changes in transcript levels of SOD1 which encodes a Cu/Zn SOD were not consistent with the SOD activity (Figure 4A). It indicated that SOD1 was not the main enzyme contributing to total SOD activity. Vaseva et al. (2012) proposed that Cu/Zn SOD was suppressed by Fe SOD in aging oat seeds. The transcript levels of APX1 and CAT1 in seeds with different MC were consistent with APX and CAT activities during storage, suggesting that APX1 and CAT1 were the major H2O2-scavenging enzymes for maintaining the balance of redox reaction in aged oat seeds. From these results, the expressions of APX1 and CAT1 in oat seeds were suppressed during aging and leading to H2O2 accumulation. Similar results have been reported for rice seeds (Yin et al., 2014).
P5CS1 catalyzes the first step of proline synthesis (Abraham et al., 2003). Overexpression of P5CS1 improved stress tolerance in rice and wheatgrass (Choudhary et al., 2005). In this study, P5CS1 transcript levels were significantly up-regulated with MC increasing from 4 to 28% in CK (Figure 6A). However, the expression of P5CS1 presented the up-regulation at 16% MC and down-regulation at 28% during 6 and 12 months storage, while the proline content increased continually. This indicated that P5CS1 transcript levels were affected significantly by the seed MC. Some studies have shown that suppression of PDH1 increased the proline content and enhanced stress resistance (Nanjo et al., 1999; Armengaud et al., 2004). In our study, PDH1 transcript levels showed the opposite trend in comparison with proline contents in oat seeds stored 12 months, and reached lower levels at MCs of 16 and 28%. This implied that PDH1 could improve stress resistance for seed aging and maintain seed vigor during long-term storage.
In summary, proline played the main role in adaptation to oxidative stress in seeds with higher MC (28%), while antioxidant enzymes (SOD, CAT, APX) played the main roles in seeds with lower MCs (4%, 16%) during storage at a low temperature (4°C). The transcript analyses showed that SOD1 was not a main factor in total SOD activity, while APX1 and CAT1 were the main H2O2-scavenging enzymes in aging oat seeds. The transcript level of P5CS1 was significantly affected by MC, and PDH1 could improve stress resistance for seed aging and maintain seed vigor during long-term storage.
Conflict of Interest Statement
The authors declare that the research was conducted in the absence of any commercial or financial relationships that could be construed as a potential conflict of interest.
Acknowledgments
This research was financially supported by earmarked fund for the China Agriculture Research System (CARS-35), National Key Technologies R&D Program of the 12th Five-Year Plan (2011BAD17B01-02), and earmarked fund for Beijing Common Construction Project.
References
Abba, E. J., and Lovato, A. (1999). Effect of seed storage temperature and relative humidity on maize (Zea mays L.) seed viability and vigour. Seed Sci. Technol. 27, 101–114.
Abraham, E., Rigo, G., Szekely, G., Nagy, R., Koncz, C., and Szabados, L. (2003). Light-dependent induction of proline biosynthesis by abscisic acid and salt stress is inhibited by brassinosteroid in Arabidopsis. Plant Mol. Biol. 51, 363–372. doi: 10.1023/A:1022043000516
PubMed Abstract | Full Text | CrossRef Full Text | Google Scholar
Armengaud, P., Thiery, L., Buhot, N., Grenier-De March, G., and Savoure, A. (2004). Transcriptional regulation of proline biosynthesis in Medicago truncatula reveals developmental and environmental specific features. Physiol. Plant. 120, 442–450. doi: 10.1111/J.0031-9317.2004.00251.X
PubMed Abstract | Full Text | CrossRef Full Text | Google Scholar
Bailly, C., Benamar, A., Corbineau, F., and Come, D. (1996). Changes in malondialdehyde content and in superoxide dismutase, catalase and glutathione reductase activities in sunflower seeds as related to deterioration during accelerated aging. Physiol. Plant. 97, 104–110. doi: 10.1111/j.1399-3054.1996.tb00485.x
Bailly, C., El-Maarouf-Bouteau, H., and Corbineau, F. (2008). From intracellular signaling networks to cell death: the dual role of reactive oxygen species in seed physiology. C. R. Biol. 331, 806–814. doi: 10.1016/J.Crvi.2008.07.022
PubMed Abstract | Full Text | CrossRef Full Text | Google Scholar
Beaucham, C., and Fridovic, I. (1971). Superoxide dismutase - improved assays and an assay applicable to acrylamide gels. Anal. Biochem. 44, 276–278. doi: 10.1016/0003-2697(71)90370–90378
PubMed Abstract | Full Text | CrossRef Full Text | Google Scholar
Choudhary, N. L., Sairam, R. K., and Tyagi, A. (2005). Expression of D1-pyrroline-5-carboxylate synthetase gene during drought in rice (Oryza sativa L.). Indian J. Biochem. Biophys. 42, 366–370.
Cooley, M. B., Yang, H., Dahal, P., Mella, R. A., Downie, A. B., Haigh, A. M.,et al. (1999). Vacuolar H+-ATPase is expressed in response to gibberellin during tomato seed germination. Plant Physiol. 121, 1339–1347. doi: 10.1104/Pp.121.4.1339
Ellis, R. H., Hong, T. D., and Roberts, E. H. (1988). A low-moisture-content limit to logarithmic relations between seed moisture-content and longevity. Ann. Bot. 61, 405–408.
Elstner, E. F., and Heupel, A. (1976). Inhibition of nitrite formation from hydroxylammoniumchloride: a simple assay for superoxide dismutase. Anal. Biochem. 70, 616–620. doi: 10.1016/0003-2697(76)90488-7
PubMed Abstract | Full Text | CrossRef Full Text | Google Scholar
Erdal, S., Aydin, M., Genisel, M., Taspinar, M. S., Dumlupinar, R., Kaya, O.,et al. (2011). Effects of salicylic acid on wheat salt sensitivity. Afr. J. Biotechnol. 10, 5713–5718.
Esfandiari, E. O., Shakiba, M. R., Mahboob, S. A., Alyari, H., and Toorchi, M. (2007). Water stress, antioxidant enzyme activity and lipid peroxidation in wheat seedling. J. Food Agric. Environ. 5, 149–153.
Evers, D., Lefèvre, I., Legay, S., Lamoureux, D., Hausman, J.-F., Rosales, R. O. G.,et al. (2010). Identification of drought-responsive compounds in potato through a combined transcriptomic and targeted metabolite approach. J. Exp. Bot. 61, 2327–2343. doi: 10.1093/jxb/erq060
PubMed Abstract | Full Text | CrossRef Full Text | Google Scholar
Gill, S. S., and Tuteja, N. (2010). Reactive oxygen species and antioxidant machinery in abiotic stress tolerance in crop plants. Plant Physiol. Biochem. 48, 909–930. doi: 10.1016/J.Plaphy.2010.08.016
PubMed Abstract | Full Text | CrossRef Full Text | Google Scholar
Goel, A., Goel, A. K., and Sheoran, I. S. (2003). Changes in oxidative stress enzymes during artificial ageing in cotton seeds. J. Plant Physiol. 160, 1093–1100. doi: 10.1078/0176-1617-00881
PubMed Abstract | Full Text | CrossRef Full Text | Google Scholar
Heneen, W. K., Karlsson, G., Brismar, K., Gummeson, P.-O., Marttila, S., Leonova, S.,et al. (2008). Fusion of oil bodies in endosperm of oat grains. Planta 228, 589–599. doi: 10.1007/s00425-008-0761-x
PubMed Abstract | Full Text | CrossRef Full Text | Google Scholar
Hoekstra, F. A., Golovina, E. A., and Buitink, J. (2001). Mechanisms of plant desiccation tolerance. Trends Plant Sci. 6, 431–438. doi: 10.1016/S1360-1385(01)02052–2050
Hu, D., Ma, G., Wang, Q., Yao, J. H., Wang, Y., Pritchard, H.,et al. (2012). Spatial and temporal nature of reactive oxygen species production and programmed cell death in elm (Ulmus pumila L.) seeds during controlled deterioration. Plant Cell Environ. 35, 2045–2059. doi: 10.1111/J.1365-3040.2012.02535.X
PubMed Abstract | Full Text | CrossRef Full Text | Google Scholar
Irina, J., Luminita, C., Dacian, L., Marinel, H., Sorin, G., and Florin, M. (2012). Aspects regarding the production and quality of some annual forage mixtures. J. Biotechnol. 161, 19–19. doi: 10.1016/J.Jbiotec.2012.07.040
ISTA. (2012). International Rules for Seed Testing, Bassersdorf: International Seed Testing Association.
Kibinza, S., Vinel, D., Côme, D., Bailly, C., and Corbineau, F. (2006). Sunflower seed deterioration as related to moisture content during ageing, energy metabolism and active oxygen species scavenging. Physiol. Plant. 128, 496–506. doi: 10.1111/j.1399-3054.2006.00771.x
Kishor, P. B. K., and Dange, V. (1990). Sucrose metabolism in callus-cultures of cotton during growth. Indian J. Exp. Biol. 28, 352–355.
Kishor, P. B. K., Hong, Z., Miao, G. H., Hu, C. A., and Verma, D. P. S. (1995). Overexpression of Δ-pyrroline-5-carboxylate synthetase increases proline production and confers osmotolerance in transgenic plants. Plant Physiol. 108, 1387–1394. doi: 10.1104/pp.108.4.1387
Kiyosue, T., Yoshiba, Y., Yamaguchishinozaki, K., and Shinozaki, K. (1996). A nuclear gene encoding mitochondrial proline dehydrogenase, an enzyme involved in proline metabolism, is upregulated by proline but downregulated by dehydration in Arabidopsis. Plant Cell 8, 1323–1335. doi: 10.2307/3870304
PubMed Abstract | Full Text | CrossRef Full Text | Google Scholar
Klose, C., and Arendt, E. K. (2012). Proteins in Ooats; their synthesis and changes during germination: a review. Crit. Rev. Food Sci. Nutr. 52, 629–639. doi: 10.1080/10408398.2010.504902
PubMed Abstract | Full Text | CrossRef Full Text | Google Scholar
Kong, Q., Mao, P. S., Yu, X. D., and Xia, F. S. (2014). Physiological changes in oat seeds aged at different moisture contents. Seed Sci. Technol. 42, 190–201. doi: 10.15258/sst.2014.42.2.08
Kumar, M., Sirhindi, G., Bhardwaj, R., Kumar, S., and Jain, G. (2010). Effect of exogenous H2O2 on antioxidant enzymes of Brassica juncea L. seedlings in relation to 24-epibrassinolide under chilling stress. Indian J. Biochem. Biophys. 47, 378–382.
Kumara, K. D. G., Xia, Y. P., Zhu, Z. J., Basnayake, S. V. M. B., and Beneragama, K. C. (2010). Effects of exogenous salicylic acid on antioxidative enzyme activities and physiological characteristics in gerbera (Gerbera jamesonii L.) grown under NaCl stress. J. Zhe jiang Univ. 36, 591–601.
Lehner, A., Mamadou, N., Poels, P., Côme, D., Bailly, C., and Corbineau, F. (2008). Changes in soluble carbohydrates, lipid peroxidation and antioxidant enzyme activities in the embryo during ageing in wheat grains. J. Cereal Sci. 47, 555–565. doi: 10.1016/j.jcs.2007.06.017
Lei, C. J., and Chang, Y. Y. (2012). Effects of NaCl and Na2CO3 stress on growth and physiological indexes in ’Beta’ grafted’ Red Globe’ grape seedlings. J. GanSu Agr. Univ. 44, 50–55.
Li, L. H., and Yi, H. L. (2012). Effect of sulfur dioxide on ROS production, gene expression and antioxidant enzyme activity in Arabidopsis plants. Plant Physiol. Biochem. 58, 46–53. doi: 10.1016/J.Plaphy.2012.06.009
PubMed Abstract | Full Text | CrossRef Full Text | Google Scholar
Lu, X. X., Chen, X. L., and Guo, Y. H. (2005). Seed germinability of 23 crop species after a decade of storage in the national genebank of China. Agr. Sci. China 4, 408–412.
Machacer, J. E., Robertson, E., Wallace, H. A. H., and Phillips, N. A. (1961). Effect of a high water content in stored wheat, oat, and barley seed on its germinability, susceptibility to invasion by moulds, and response to chemical treatment. Can. J. Plant Sci. 41, 288–303. doi: 10.4141/cjps61-040
Magdalena, R., Isaura, M., and Celia de la, C. (1999). Cereal seed viability after 10 years of storage in active and base germplasm collections. Filed Crops Res. 64, 229–236. doi: 10.1016/S0378-4290(99)00044-1
McDonald, M. B. (1999). Seed deterioration: physiology, repair and assessment. Seed Sci. Technol. 27, 177–237.
Melchiorre, M., Robert, G., Trippi, V., Racca, R., and Lascano, H. R. (2009). Superoxide dismutase and glutathione reductase overexpression in wheat protoplast: photooxidative stress tolerance and changes in cellular redox state. Plant Growth Regul. 57, 57–68. doi: 10.1007/S10725-008-9322–9323
Miller, G., Stein, H., Honig, A., Kapulnik, Y., and Zilberstein, A. (2005). Responsive modes of Medicago sativa proline dehydrogenase genes during salt stress and recovery dictate free proline accumulation. Planta 222, 70–79. doi: 10.1007/S00425-005-1518–1514
PubMed Abstract | Full Text | CrossRef Full Text | Google Scholar
Mira, S., Estrelles, E., and González-Benito, M. E. (2015). Effect of water content and temperature on seed longevity of seven Brassicaceae species after 5 years of storage. Plant Biol. 17, 153–162. doi: 10.1111/plb.12183
PubMed Abstract | Full Text | CrossRef Full Text | Google Scholar
Mittal, S., Kumari, N., and Sharma, V. (2012). Differential response of salt stress on Brassica juncea: Photosynthetic performance, pigment, proline, D1 and antioxidant enzymes. Plant Physiol. Biochem. 54, 17–26. doi: 10.1016/J.Plaphy.2012.02.003
PubMed Abstract | Full Text | CrossRef Full Text | Google Scholar
Moller, I. M., Jensen, P. E., and Hansson, A. (2007). Oxidative modifications to cellular components in plants. Annu. Rev. Plant Biol. 58, 459–481. doi: 10.1146/Annurev.Arplant.58.032806.103946
PubMed Abstract | Full Text | CrossRef Full Text | Google Scholar
Murthy, U. M. N., Liang, Y. H., Kumar, P. P., and Sun, W. Q. (2002). Non-enzymatic protein modification by the Maillard reaction reduces the activities of scavenging enzymes in Vigna radiata. Physiol. Plant. 115, 213–220. doi: 10.1034/J.1399-3054.2002.1150206.X
PubMed Abstract | Full Text | CrossRef Full Text | Google Scholar
Nanjo, T., Kobayashi, M., Yoshiba, Y., Kakubari, Y., Yamaguchi-Shinozaki, K., and Shinozaki, K. (1999). Antisense suppression of proline degradation improves tolerance to freezing and salinity in Arabidopsis thaliana. FEBS Lett. 461, 205–210. doi: 10.1016/S0014-5793(99)01451–1459
PubMed Abstract | Full Text | CrossRef Full Text | Google Scholar
Noctor, G., and Foyer, C. H. (1998). Ascorbate and glutathione: keeping active oxygen under control. Annu. Rev. Plant Physiol. Plant Mol. Biol. 49, 249–279. doi: 10.1146/Annurev.Arplant.49.1.249
PubMed Abstract | Full Text | CrossRef Full Text | Google Scholar
Oliveira, J. T. A., Andrade, N. C., Martins-Miranda, A. S., Soares, A. A., Gondim, D. M. F., Araujo, J. H.,et al. (2012). Differential expression of antioxidant enzymes and PR-proteins in compatible and incompatible interactions of cowpea (Vigna unguiculata) and the root-knot nematode Meloidogyne incognita. Plant Physiol. Biochem. 51, 145–152. doi: 10.1016/J.Plaphy.2011.10.008
PubMed Abstract | Full Text | CrossRef Full Text | Google Scholar
Pekka, L., Katja, K., Ilkka, L., and Simo, L. (2003). Effect of heat treatment on lipid stability in processed oats. J. Cereal Sci. 37, 215–221. doi: 10.1006/jcrs.2002.0496
Price, H. J. (1975). Unilateral seed failure after reciprocal crosses of Secale cereale with Secale africanum. Can. J. Bot. 53, 929–932. doi: 10.1139/b75-110
Pukacka, S., and Ratajczak, E. (2005). Production and scavenging of reactive oxygen species in seeds during storage at varied temperature and humidity. J. Plant Physiol. 162, 873–885. doi: 10.1016/j.jplph.2004.10.012
PubMed Abstract | Full Text | CrossRef Full Text | Google Scholar
Rajjou, L., and Debeaujon, I. (2008). Seed longevity: survival and maintenance of high germination ability of dry seeds. C. R. Biol. 331, 796–805. doi: 10.1016/j.crvi.2008.07.021
PubMed Abstract | Full Text | CrossRef Full Text | Google Scholar
Smirnoff, N., and Cumbes, Q. J. (1989). Hydroxyl radical scavenging activity of compatible solutes. Phytochemistry 28, 1057–1060. doi: 10.1016/0031-9422(89)80182–80187
Spanò, C., Castiglione, M. R., Bottega, S., and Grilli, I. (2004). Natural ageing of wheat seeds. Curr. Top. Plant Biol. 5, 89–94.
Vaseva, I., Akiscan, Y., Simova-Stoilova, L., Kostadinova, A., Nenkova, R.,Anders, I.,et al. (2012). Antioxidant response to drought in red and white clover. Acta Physiol. Plant. 34, 1689–1699. doi: 10.1007/S11738-012-0964–964
Wojtyla,Ł., Garnczarska, M., Zalewski, T., Bednarski, W., Ratajczak, L., and Jurga, S. (2006). A comparative study of water distribution, free radical production and activation of antioxidative metabolism in germinating pea seeds. J. Plant Physiol. 163, 1207–1220. doi: 10.1016/j.jplph.2006.06.014
PubMed Abstract | Full Text | CrossRef Full Text | Google Scholar
Yao, Z., Liu, L. W., Gao, F., Rampitsch, C., Reinecke, D. M., Ozga, J. A.,et al. (2012). Developmental and seed aging mediated regulation of antioxidative genes and differential expression of proteins during pre- and post-germinative phases in pea. J. Plant Physiol. 169, 1477–1488. doi: 10.1016/ J.Jplph. 2012.06.001
PubMed Abstract | Full Text | CrossRef Full Text | Google Scholar
Yin, G. K., Xin, X., Song, C., Chen, X. L., Zhang, J. M., Wu, S. H.,et al. (2014). Activity levels and expression of antioxidant enzymes in the ascorbate-glutathione cycle in artificially aged rice seed. Plant Physiol. Biochem. 80, 1–9. doi: 10.1016/J.Plaphy.2014.03.006
PubMed Abstract | Full Text | CrossRef Full Text | Google Scholar
Zhang, M., Zhuo, J. J., Wang, X., Wu, S., and Wang, X. F. (2010). Optimizing seed water content: relevance to storage stability and molecular mobility. J. Integr. Plant Biol. 52, 324–331. doi: 10.1111/J.1744-7909.2010. 00916.X
PubMed Abstract | Full Text | CrossRef Full Text | Google Scholar
Keywords: oat seed, antioxidant enzymes, proline, storage duration, moisture content, gene expression regulation
Citation: Kong L, Huo H and Mao P (2015) Antioxidant response and related gene expression in aged oat seed. Front. Plant Sci. 6:158. doi: 10.3389/fpls.2015.00158
Received: 24 December 2014; Accepted: 28 February 2015;
Published online: 19 March 2015.
Edited by:
Zhulong Chan, Wuhan Botanic Garden – Chinese Academy of Sciences, ChinaReviewed by:
Giridara Kumar Surabhi, Regional Plant Resource Centre, IndiaGongshe Liu, Institute of Botany, China
Copyright © 2015 Kong, Huo and Mao. This is an open-access article distributed under the terms of the Creative Commons Attribution License (CC BY). The use, distribution or reproduction in other forums is permitted, provided the original author(s) or licensor are credited and that the original publication in this journal is cited, in accordance with accepted academic practice. No use, distribution or reproduction is permitted which does not comply with these terms.
*Correspondence: Peisheng Mao, Forage Seed Lab, China Agriculture University, No 2, Yuanmingyuan West Road, Haidian, Beijing 100193, ChinabWFvcHNAY2F1LmVkdS5jbg==