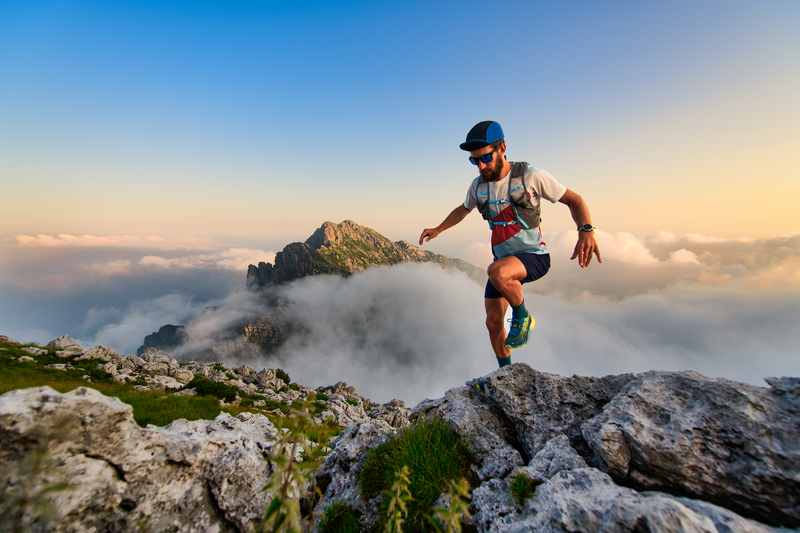
95% of researchers rate our articles as excellent or good
Learn more about the work of our research integrity team to safeguard the quality of each article we publish.
Find out more
ORIGINAL RESEARCH article
Front. Plant Sci. , 16 February 2015
Sec. Plant Physiology
Volume 6 - 2015 | https://doi.org/10.3389/fpls.2015.00066
This article is part of the Research Topic Lipid signalling in plant development and responses to environmental stresses. View all 12 articles
Aluminum ions (Al) have been recognized as a major toxic factor for crop production in acidic soils. The first indication of the Al toxicity in plants is the cessation of root growth, but the mechanism of root growth inhibition is largely unknown. Here we examined the impact of Al on the expression, activity, and function of the non-specific phospholipase C4 (NPC4), a plasma membrane-bound isoform of NPC, a member of the plant phospholipase family, in Arabidopsis thaliana. We observed a lower expression of NPC4 using β-glucuronidase assay and a decreased formation of labeled diacylglycerol, product of NPC activity, using fluorescently labeled phosphatidylcholine as a phospholipase substrate in Arabidopsis WT seedlings treated with AlCl3 for 2 h. The effect on in situ NPC activity persisted for longer Al treatment periods (8, 14 h). Interestingly, in seedlings overexpressing NPC4, the Al-mediated NPC-inhibiting effect was alleviated at 14 h. However, in vitro activity and localization of NPC4 were not affected by Al, thus excluding direct inhibition by Al ions or possible translocation of NPC4 as the mechanisms involved in NPC-inhibiting effect. Furthermore, the growth of tobacco pollen tubes rapidly arrested by Al was partially rescued by the overexpression of AtNPC4 while Arabidopsis npc4 knockout lines were found to be more sensitive to Al stress during long-term exposure of Al at low phosphate conditions. Our observations suggest that NPC4 plays a role in both early and long-term responses to Al stress.
Aluminum (Al) toxicity represents a major growth-limiting factor for the regions with acid soils. Low pH of soil enables the release of toxic Al ions from its insoluble forms fixed in soil minerals. Prolonged exposure to Al ions leads to changes in root morphology, e.g., root thickening, bursting, changes in the cell wall architecture, and even cell death. However, the first indication of the Al toxicity in plants is rapid cessation of root growth. The root tip has been found to be the most Al-responsive part of roots (Panda et al., 2009). Although molecular mechanisms of the prompt Al-mediated root growth inhibition are largely unclear, research on the targets of Al action in plants has demonstrated that Al enters and binds to the apoplast (Wissemeier and Horst, 1995) and changes the properties of the PM. A number of physiologically important processes connected with PM are affected by Al. Well documented early consequences of Al toxicity are lipid peroxidation (Boscolo et al., 2003), the disruption of ion fluxes (Matsumoto, 2000), the disruption of calcium homeostasis (Rengel and Zhang, 2003), the inhibition of nitric oxide synthase (Tian et al., 2007), effects on the cytoskeleton (Sivaguru et al., 1999, 2003; Schwarzerová et al., 2002), and the depolarization of the PM (Sivaguru et al., 2003; Illéš et al., 2006). It has been found that rapid Al-mediated inhibition of root growth is related to the loss of PM fluidity and the inhibition of endocytosis (Illéš et al., 2006; Krtková et al., 2012) and it is controlled through local auxin biosynthesis and signaling (Shen et al., 2008; Yang et al., 2014). The rapid response of root growth suggests that signaling pathways are a part of the mechanism participating in Al toxicity.
Phospholipid-signaling pathway is now considered to be one of the important plant signaling mechanisms involved in many different reactions of plants to environmental factors such as drought, cold, salinity, or pathogen attack [for review see Munnik (2010) and Wang (2014)]. Al has been shown to affect the phospholipid-signaling pathway as well. Changes of phospholipase A2 activity in vitro (Jones and Kochian, 1997), PLD activity (Pejchar et al., 2008; Zhao et al., 2011), and PI-PLC activity (Jones and Kochian, 1995; Martínez-Estévez et al., 2003; Ramos-Díaz et al., 2007) after Al treatment were demonstrated. In addition to PI-PLC, NPC, an enzyme that is able to hydrolyze phosphatidylcholine (PC) instead of PIP2, was characterized in plants (Nakamura et al., 2005) in relation with phospholipid-to-galactosyl DAG exchange (Andersson et al., 2005; Nakamura et al., 2005; Gaude et al., 2008; Tjellström et al., 2008), elicitor signaling (Scherer et al., 2002), root development (Wimalasekera et al., 2010), hormone signaling (Peters et al., 2010; Wimalasekera et al., 2010), salt stress (Kocourková et al., 2011; Peters et al., 2014), and Al stress (Pejchar et al., 2010).
Arabidopsis NPC gene family consists of six members, denoted NPC1-NPC6, exhibiting differences in their localization and in their biochemical properties [for review see Pokotylo et al. (2013)]. Briefly, experimentally non-characterized NPC1, NPC2, and NPC6 were supposed to contain putative N-terminal signal peptide with predicted localization in endomembranes and specific organelles (Pokotylo et al., 2013). NPC3 was described to lack the ability to hydrolyze PC (Reddy et al., 2010), NPC4 to be PM-bound protein (Nakamura et al., 2005), NPC5 to be cytosolic-localized enzyme expressed only in floral organs under normal conditions (Gaude et al., 2008; Pokotylo et al., 2013). NPC4 and NPC5 were able to hydrolyze PC, however, NPC5 possessed 40-fold lower hydrolytic activity than NPC4 (Gaude et al., 2008).
We previously demonstrated that Al ions inhibit the formation of DAG generated by NPC in tobacco BY-2 cell line and pollen tubes, inhibit the growth of tobacco pollen tubes and that this growth, arrested by Al, can be rescued by an externally added DAG (Pejchar et al., 2010). This raises the following question: which NPC isoform is Al-targeted and what is the role of DAG in aluminum toxicity?
Here we report our findings that Al ions inhibit the expression of NPC4 and decrease its enzymatic activity. However, the latter effect is caused neither by the direct NPC4 inhibition by Al ions nor by NPC4 translocation. Moreover, the overexpression of AtNPC4 rapidly alleviated Al-mediated retardation of tobacco pollen tubes while Arabidopsis npc4 knockout lines were found to be more sensitive to Al stress during long-term exposure of Al at low phosphate (P) conditions.
Arabidopsis thaliana Columbia (Col-0) seeds were obtained from Lehle seeds and used as wild-type (WT) controls. The T-DNA insertion line npc4 (SALK_046713) used in our experiments was characterized earlier (Wimalasekera et al., 2010). Arabidopsis plants were grown on agar plates containing 2.2 gl-1 1/2 MS basal salts and 1% (w/v) agar (pH 5.8). Seeds were surface sterilized with 30% (v/v) bleach solution for 10 min and rinsed five times with sterile water. To synchronize seed germination, the agar plates were kept for 3 days in a dark at 4°C. The plants were grown in the vertical position in a growth chamber at 22°C under long day conditions (16/8 h light/dark cycle). Tobacco (Nicotiana tabacum cv. Samsun) pollen grains germinated on simple sucrose medium (Pleskot et al., 2012) containing 10% (w/v) sucrose and 0.01% (w/v) boric acid solidified by 0.5% (w/v) agar were used for biolistic transformation.
The NPC activity in Arabidopsis seedlings was measured according to Kocourková et al. (2011). Seven-day-old Arabidopsis seedlings (five seedlings for each sample) were transferred from liquid MS solution to 1/8 MS medium containing 10 μM AlCl3, pH 4 and labeled with 0.66 μg ml-1 of fluorescent PC (BODIPY-PC, D-3771, Invitrogen, USA). Seedlings were incubated on an orbital shaker at 23°C for 2 h. NPC activity in vitro was measured according to Pejchar et al. (2013) using β-BODIPY-PC (D-3792, Invitrogen, USA). The identification of a BODIPY-DAG corresponding spot was based on a comparison with the BODIPY-DAG standard prepared as described earlier (Pejchar et al., 2010).
The construction of promoter:GUS plants was described previously (Wimalasekera et al., 2010). Seeds of pNPC4:GUS were grown on agar plates under the same conditions as described in Section “Plant Material.” Ten-day-old seedlings were transferred to a 24-well plate containing 1 ml of 1/8 MS solution with or without 100 μM AlCl3, pH 4 for 24 h. The histochemical GUS assay (Jefferson et al., 1987) was carried out according to Kocourková et al. (2011).
His:AtNPC4. The AtNPC4 coding sequence was amplified from Arabidopsis Col-0 cDNA with the specific forward primer 5′-CGCGAATTCATGATCGAGACGACCAAA-3′ and the reverse primer 5′-GCCCTCGAGTCAATCATGGCGAATAAAG-3′ by PCR using Phusion DNA polymerase (Finnzymes), digested with XhoI and EcoRI enzymes and cloned into the pET30a(+) vector (Novagen). The expression vector was transformed into the Escherichia coli strain BL21 and cells were grown overnight at 37°C. After subculturing into fresh medium, the cells were grown at 16°C to an OD600 of approximately 0.4, then induced overnight with 0.1 mM isopropyl thio-β-D-galactoside. The cells were harvested by centrifugation (5000 ×g, 10 min), resuspended in an assay buffer (50 mM Tris-HCl; pH 7.3, 50 mM NaCl, 5% glycerol; Nakamura et al., 2005), and sonicated after 10 min treatment with lysozyme (1 mg ml-1). The lysed cell suspension was centrifuged (10000 ×g, 10 min) and supernatant was used for an enzyme activity assay. The western blot analysis was performed under reducing and denaturation conditions with SDS electrophoresis. 6x His tag was detected with Anti-His HRP Conjugate (Qiagen).
35S::AtNPC4/35S::GFP:AtNPC4. AtNPC4 cloned into the pENTR223.1 entry vector (GatewayTM clone G12733, Arabidopsis Biological Resource Center) was recombined by LR reaction into the Gateway binary vector pGWB2 (35S::AtNPC4) or pGWB6 (35S::GFP:AtNPC4) under the control of the CaMV 35S promoter (Nakagawa et al., 2007). Constructs were transferred into Agrobacterium tumefaciens strain GV2260 and used to transform Arabidopsis Col-0 WT plants by the floral dip method (Clough and Bent, 1998). Transformants were selected on agar plates containing 50 μg ml-1 kanamycin and 50 μg ml-1 hygromycin B. Expression levels of NPC4 in 10-day-old T3 seedlings of homozygous lines were measured using the quantitative RT-PCR. Lines with the highest expression levels of NPC4 were used in experiments.
Lat52::AtNPC4:YFP. The AtNPC4 coding sequence flanked by NgoMIV and ApaI sites was generated by PCR with Phusion DNA polymerase (Finnzymes) using the specific forward primer 5′-ATAGCCGGCATGATCGAGACGACCAA-3′ and the reverse primer 5′-TATGGGCCCATCATGGCGAATAAAGCA-3′. An amplified product was introduced into the multiple cloning sites of the pollen expression vector pHD32. The pHD32 vector (Lat52::MCS::GA5::YFP::NOS; Klahre et al., 2006) was kindly provided by Prof. Benedikt Kost (University of Erlangen-Nuremberg, Erlangen, Germany). This construct allowed the pollen-specific expression and visualization of AtNPC4 protein fusions controlled by the Lat52 promoter (Twell et al., 1991). The expression vector was transferred into tobacco pollen grains germinating on solid culture medium by particle bombardment using a helium-driven particle delivery system (PDS-1000/He; Bio-Rad, Hercules, CA, USA) as previously described (Kost et al., 1998). Particles were coated with 1 μg DNA.
For live-cell imaging, a Zeiss LSM 5 DUO confocal laser scanning microscope with a 940 Zeiss C-Apochromat 340/1.2 water-corrected objective was used. For YFP/GFP imaging, singletrack acquisitions with 514 nm excitation, a 458/514 nm dichroic mirror, a 530–600 nm emission filter (YFP) and 488 nm excitation, a 488 nm dichroic mirror, a 505–550 emission filter (GFP) were used.
To analyze root length, five-day-old seedlings grown on agar (for details see Plant Material) were transferred on agar plates containing 1/8 MS, pH 4 supplemented with 200 μM AlCl3. After 9 day incubation, plates were scanned (Canon CanoScan 8800F) and the root growth was measured using the JMicroVision 1.2.7 software.
To measure pollen tubes length, tobacco pollen was transiently transformed with AtNPC4:YFP by particle bombardment. After 6 h of germination in dark, pollen tubes were incubated in liquid simple sucrose medium (pH 5) with or without 50 μM AlCl3 for additional 2 h. Mean growth rate of pollen tubes expressing AtNPC4:YFP and vector-only control was evaluated using the fluorescence microscope Olympus BX-51.
To determine survival rate, 7-day-old seedlings grown on agar (for details see Plant Material) were transferred to 6-well plates with liquid 1/8 Hoagland’s (Kocourková et al., 2011) solution (pH 4) with 100 μM AlCl3 for 22 days. Plates pictures were taken by Nikon SMZ 1500 zoom stereoscopic microscope coupled to a Nikon DS-5M digital camera. The survival rate was calculated as a number of viable true leaves.
Aluminum ions were described to inhibit the formation of DAG generated by NPC in tobacco cell line BY-2 and in tobacco pollen tubes (Pejchar et al., 2010). In order to find an NPC isoform that is responsible for the decrease of DAG formation during Al stress, described biochemical properties and localizations of all NPC isoforms were taken into account (see Introduction for details). Altogether, NPC4 was the first candidate to be investigated.
Considering all available data about the NPC gene family and given that the root and PM are the main targets of Al toxicity, we chose NPC4 to study its role in Al stress. Although NPC4 is not the most abundant NPC gene expressed in roots (Peters et al., 2010; Wimalasekera et al., 2010; Pokotylo et al., 2013) it was described as the isoform with the strongest response to abiotic stress in plants (Kocourková et al., 2011). In our previous studies, we have shown that the expression of NPC4, investigated using pNPC4:GUS plants, was largely localized in the root tip (Wimalasekera et al., 2010; Kocourková et al., 2011). In this study, we performed histochemical analysis of Arabidopsis pNPC4:GUS seedlings treated with AlCl3 to observe changes in the expression pattern of NPC4 during Al stress. GUS staining in both control and Al-treated seedlings was found in the apical meristem and partly in the elongation zone of main and lateral root but the intensity of GUS staining signal was lower in Al-treated seedlings (Figure 1). These observations suggest that the NPC4 expression is decreased during Al stress in Arabidopsis seedlings.
FIGURE 1. Histochemical analysis of pNPC4:GUS expression in Al-treated Arabidopsis seedlings. Ten-day-old Arabidopsis seedlings grown on agar were transferred to liquid 1/8 MS solution with or without AlCl3 for 24 h. Final observations were done on a zoom stereoscopic microscope. This experiment was repeated twice with similar results.
The involvement of NPC4 in Al stress was also examined in the level of its activity. Because we used a different plant model organism than in our previous work (Pejchar et al., 2010), we first tested the reaction of Arabidopsis seedlings to Al stress. To study changes in the DAG pattern under Al stress, we used the fluorescent derivative of PC (BODIPY-PC) as a phospholipase substrate. When seven-day-old seedlings were treated with different concentrations of AlCl3 in the presence of BODIPY-PC for 2 h, a concentration-dependent inhibiting effect of Al on BODIPY-DAG formation was observed (Pejchar, unpublished), revealing 10 μM AlCl3 as a working concentration for in situ activity measurement (Figure 2).
FIGURE 2. BODIPY-diacylglycerol (DAG) production in Arabidopsis seedlings treated with Al for different times. Seven-day-old WT and NPC4-overexpressing seedlings were treated with 10 μM AlCl3 for different time intervals (0, 6, and 12 h) and then incubated with BODIPY-phosphatidylcholine (PC) for 2 h. Lipids were extracted at the time intervals indicated, separated by high-performance thin layer chromatography and quantified. Each value is related to the control non-treated cells (100%). The plotted values are the means + SEM from three independent experiments with parallel samples. NPC, non-specific phospholipase C.
Consequently, we tested the hypothesis that NPC4 is also the targeted isoform at activity level during Al stress and it is responsible for the inhibition of DAG formation. Therefore, a stable Arabidopsis line overexpressing NPC4 under the control of 35S promoter (NPC4-OE) was prepared and was monitored to BODIPY-DAG formation after Al treatment and compared to Al-treated WT seedlings. First, our HP-TLC analysis of the labeled products showed that the trend of the Al-induced BODIPY-DAG inhibition (∼35% of control, non-treated seedlings) in WT seedlings was similar also for prolonged treatments with 10 μM AlCl3 (Figure 2) with a slightly diminished effect of Al after 14 h of treatment (∼47% of control). NPC4-OE seedlings were slightly less sensitive to Al compared to WT when treated for 2 and 8 h. Intriguingly, the overexpression of NPC4 resulted in a more pronounced difference (∼74% compared to ∼47% of control) after 14 h Al treatment. This suggests that NPC4 is an Al-sensitive NPC isoform on activity level during Al stress, as well.
To test possible mechanisms that influenced NPC4 activity in Al stress, a heterologously expressed NPC4 protein was prepared (Figure 3A) and incubated with Al in vitro to detect possible direct inhibition of NPC4 by Al. However, β-BODIPY-DAG formation in Al-treated samples was not affected compared to non-treated samples (Figure 3B) indicating that NPC4 is not directly inhibited by Al.
FIGURE 3. In vitro activity of NPC4 is not altered by Al. (A) Western blot of His:NPC4 and vector control was probed with Anti-His HRP Conjugate (Qiagen). Arrow head indicates His:NPC4 protein. (B) NPC activity was determined in vitro using fluorescent substrate β-BODIPY-PC in the presence of AlCl3. The plotted values are the means + SD from two independent experiments performed in duplicates (n = 4). DAG, diacylglycerol.
Given that PM is well-documented cellular target of Al and that NPC4 was described as a PM-localized protein (Nakamura et al., 2005), we studied possible translocation of NPC4 from PM during Al treatment, which should cause a decrease in the DAG formation. The protein translocation under stress conditions was previously described in plants for another phospholipase type, PLD (Wang et al., 2000; Bargmann et al., 2006). To check this mechanism, stable Arabidopsis transformants harboring fusion protein GFP:NPC4 were prepared. Seven-day-old seedlings were transferred to 1% (w/v) sucrose (pH 4.3) solution containing 50 μM AlCl3 and the localization of GFP:NPC4 in roots was investigated with a laser scanning confocal microscope. In control, non-treated seedlings, the PM localization of GFP:NPC4 was detected confirming previously published results (Nakamura et al., 2005). The localization of GFP:NPC4 remained unchanged in Al-treated seedlings (Figure 4, upper panels). The same results were obtained for transiently transformed tobacco pollen tubes expressing AtNPC4:YFP under the control of pollen specific Lat52 promoter (Figure 4, lower panels), another plant model organism used in this study. These results provide evidence that NPC4 translocation is not a mechanism that induces DAG decrease during Al stress.
FIGURE 4. Localization of NPC4 is not changed by Al treatment. Influence of AlCl3 on localization of AtNPC4 was observed in 7-day-old stable Arabidopsis transformants (GFP:AtNPC4, upper panels) and transiently transformed tobacco pollen tubes (AtNPC4:YFP, lower panels) by confocal laser scanning microscopy. Bars, 10 μm. NPC, non-specific phospholipase C.
Next, a root growth phenotype under Al stress was investigated in Arabidopsis WT, npc4 knockout line and NPC4-OE. Five-day-old seedlings grown on agar MS medium were transferred on agar 1/8 MS medium containing 200 μM AlCl3. The growth of the main root of all lines tested was retarded in the presence of Al. However, the root growth ratio between Al-treated and non-treated seedlings was not different for examined lines (Figure 5).
FIGURE 5. Root growth assay of WT, npc4 and NPC4-overexpressing Arabidopsis seedlings treated with Al. Five-day-old seedlings were transferred on agar plates containing 1/8 MS supplemented with AlCl3. After 9 day incubation, the root growth was measured and compared to non-treated controls. The plotted values represent the means (left panel) and ratios of Al-treated/non-treated control seedlings (right panel) + SD from two independent experiments. At least 16 seedlings were measured for each variant. NPC, non-specific phospholipase C.
This could be explained by possible compensation of NPC4 function in stable transformants by another member of lipid signaling enzyme network. To bypass this, we employed transient transformation in heterologous system of tobacco pollen. Moreover, in our previously published study (Pejchar et al., 2010), DAG was shown to restore growth inhibition caused by Al treatment in tobacco pollen tubes. To test the possible role of NPC4 in this DAG function, tobacco pollen tubes were transiently transformed with AtNPC4:YFP under the control of pollen specific Lat52 promoter and the length of pollen tubes overexpressing AtNPC4:YFP was determined in the presence of Al. In the control pollen tubes overexpressing YFP alone, the cytoplasmic YFP localization was found (data not shown) and the mean growth rate was 2.36 ± 0.12 μm min-1 (Figure 6). Al-treatment caused the inhibition of control pollen tubes growth to approximately 15% (0.34 ± 0.03 μm min-1) of non-treated cells. Control pollen tubes overexpressing AtNPC4:YFP showed a slightly decreased mean growth rate (2.17 ± 0.09 μm min-1) compared to YFP only. However, in the presence of Al the mean growth of pollen tubes overexpressing AtNPC4:YFP (1.01 ± 0.05 μm min-1) was higher compared to Al-treated vector-only control (Figure 6). Taken together, these results clearly demonstrate that the role of DAG as a growth activator in Al stress is mediated by NPC4 activity.
FIGURE 6. Effect of Al on the growth rate of tobacco pollen tubes transiently expressing AtNPC4:YFP. Tobacco pollen was sown on solid germination medium and transiently transformed with AtNPC4:YFP by particle bombardment. After 6 h of germination, pollen tubes were incubated with 50 μM AlCl3 for additional 2 h. Mean growth rate of pollen tubes expressing AtNPC4:YFP or vector-only control was evaluated using a fluorescence microscope. Data shown are from two independent experiments and represent means + SEM. At least 21 pollen tubes were measured for each variant. NPC, non-specific phospholipase C.
Based on the results concerning the role of NPC4 activity in longer time period of Al treatment (Figure 2), a long-term survival experiment was also performed. Seven-day-old seedlings grown on agar were transferred to liquid 1/8 Hoagland’s solution with AlCl3 for 22 days. However, all tested lines (WT, npc4, NPC4-OE) showed no difference in their survival rate (data not shown). Since NPC4 play a role in P starvation (Nakamura et al., 2005) and aluminum stress and P deficiency co-exist in acid soils (Ruíz-Herrera and López-Bucio, 2013), the same experiment was repeated under P deficiency conditions. Differences in both root abundance (Figure 7A) and survival rate (Figures 7A,B) were found between WT and npc4 seedlings after Al treatment. Seedlings of npc4 were able to form only a weaker root system and significantly (t-test, p < 0.0001) less true leaves comparing to WT. This suggests that npc4 seedlings are more sensitive to Al stress at low P conditions. In contrast to this finding, we also saw that NPC4-OE seedlings formed a more abundant root system compared to WT (Figure 7A). However, only a minimal increase of survival rate was found for NPC4-OE (Figures 7A,B). Collectively, these data strongly support the involvement of NPC4 in response to long-term Al exposure.
FIGURE 7. Effect of Al on the survivance of WT, npc4, and NPC4-overexpressing Arabidopsis seedlings under phosphate deficiency. (A) Seven-day-old seedlings grown on agar were transferred to liquid 1/8 Hoagland’s solution with AlCl3 for 22 days. (B) Quantitative analysis of survival rate calculated as a number of viable true leaves. The plotted values are the means + SD from two independent experiments (n = 50). NPC, non-specific phospholipase C.
Several physiologically important cellular processes are affected by Al, the major growth-limiting factor for the regions with acid soils. However, time sequence and the exact mechanism of processes involved in Al stress are still under investigation. Phospholipases, namely PI-PLC and PLD, have been shown to be affected within minutes as well as in longer time periods after Al treatment (Martínez-Estévez et al., 2003; Ramos-Díaz et al., 2007; Pejchar et al., 2008; Zhao et al., 2011). We previously described that the formation of DAG generated by NPC is rapidly inhibited by Al in tobacco cell line BY-2 and in tobacco pollen tubes and that Al inhibits growth of tobacco pollen tubes. These results, together with the fact that Al-mediated growth arrest can be rescued by an externally added DAG (Pejchar et al., 2010), raised a question which NPC isoform is Al-targeted in aluminum toxicity. Here we showed that NPC isoform NPC4 is involved in the response of A. thaliana to Al exposure.
We selected Arabidopsis NPC4 as the primary candidate gene based on the expression and localization criteria (see above). The expression analysis using pNPC4:GUS assay showed that the localization and the intensity of NPC4 expression in the non-treated main root was the same as published previously (Wimalasekera et al., 2010). However, we found stronger GUS staining in non-treated lateral roots (Figure 1). This difference could be explained by different experimental conditions used as a control for Al treatments (pH 4) or by small variation of seedling age used for GUS assay. More importantly, Al treatment caused reduction in GUS staining in both main and lateral roots suggesting that the NPC4 expression is decreased during Al treatment. In addition, pNPC4:GUS expression was confined mainly to the root tip (Figure 1), a plant tissue that was found to be the most Al-responsive part of roots (Panda et al., 2009).
Although we confirmed the generally accepted symptom of Al toxicity, root growth inhibition, and we observed diminished NPC4 expression after Al treatment, we were not able to determine differences between Al-treated WT, npc4, and NPC4-OE Arabidopsis seedlings (Figure 5). We have two hypotheses regarding the lack of the differences. First, it is possible that no differences were found because NPC4 is involved in other aspects of the response to Al stress than in the studied root growth phenotype. Alternatively, the lack of the differences is caused by the positive or negative compensatory effect of another NPC isoform (or other lipid signaling genes) that may be up- or down-regulated in npc4 knockout or stable NPC4-OE line, respectively. Similar observations were indeed described for studies dealing with other lipid signaling genes, such as PLD (Bargmann et al., 2009; Johansson et al., 2014) or phospholipase A (Rietz et al., 2010). All six NPC sequences are highly conserved with four invariable motives, however, the C-termini form the most divergent part of NPC sequences, with distinct lengths and sequence conservation among NPC subfamilies. This may be the part of the molecule responsible for the functional differences of various NPC isoforms through facilitating interactions with other proteins or defining protein localization (Pokotylo et al., 2013). Interestingly, while NPC3-5 are found in triplicate in Arabidopsis genome and members of this subfamily can also be found in other monocot and dicot species, it seems to be missing in gymnosperms and it is also absent in Solanaceae (Potocký, unpublished). Taking advantage of this, we employed a strategy of studying the effect of Al in tobacco pollen tubes heterologously overexpressing AtNPC4:YFP under the control of strong pollen specific Lat52 promoter. The growth of tobacco pollen tubes was rapidly arrested by Al, supporting our previous results (Pejchar et al., 2010), and was partially rescued by the overexpression of AtNPC4:YFP (Figure 6). Together with Al-mediated pollen growth inhibition rescue by exogenously added DAG (Pejchar et al., 2010), this strongly suggests that NPC4-generated DAG plays a role in the response to the Al-mediated toxicity.
Because a different plant model organism than in our previous work was used, we next tested the reaction of Arabidopsis seedlings to Al stress in the view of NPC activity. We utilized the same fluorescent derivative of PC as a phospholipase substrate and found a similar Al-mediated NPC-inhibiting effect (Figure 2) as for the tobacco cell line BY-2 and pollen tubes (Pejchar et al., 2010) suggesting that this phenomenon could be conserved across the plant kingdom. Interestingly, effects on pNPC4:GUS expression and NPC activity in Al-treated plants were in the opposite way than described for another abiotic stress that targets root, salt treatment (Kocourková et al., 2011). To test the hypothesis whether NPC4 is responsible for inhibition of DAG formation during Al stress, we prepared the stable Arabidopsis lines overexpressing NPC4 and we compared the ratio of NPC activity in Al-treated/non-treated seedlings to WT seedlings. The differences between WT and NPC4-OE seedlings were slowly pronounced in time with the most evident change obtained for the seedlings treated with Al for 14 h (Figure 2) indicating that NPC4 activity is altered by Al gradually. Two possible mechanisms that could be responsible for the decrease of NPC activity were examined. The inhibition of phospholipase activity in vitro in cellular fractions is well documented for different toxic metals (Pokotylo et al., 2014) and for Al as well (Martínez-Estévez et al., 2003; Pejchar et al., 2008). Here, the direct inhibition of heterologously expressed NPC4 enzyme by Al was tested with no alteration in activity detected even for high AlCl3 concentrations (Figure 3). The second possible mechanism, enzyme translocation, was previously described in plants under stress conditions for another phospholipase type, PLD (Wang et al., 2000; Bargmann et al., 2006). However, Al treatment had no effect on NPC4 localization neither in Arabidopsis seedlings nor tobacco pollen tubes (Figure 4).
Moreover, AtNPC4:YFP was found on PM in subapical region of growing pollen tube (Figure 4) and thus partially co-localize with Cys1:YFP that was used as a DAG marker in tobacco pollen tubes (Potocký et al., 2014). DAG is an important signaling phospholipid in animals but its signaling role in plant cells is still under debate. Meijer and Munnik (2003) reported that DAG as a product of PIP2 hydrolysis is rapidly phosphorylated by DAG kinase to PA, which plays an active role in the plant signaling processes. However, a number of studies imply that DAG is likely to act as a signaling molecule in some plant systems including Arabidopsis seedlings and tobacco pollen tubes [reviewed in Dong et al. (2012)]. DAG is also known to be important in the structure and dynamics of biological membranes, where it can influence membrane curvature and induce unstable, asymmetric regions in membrane bilayers important for membrane fusion processes (Carrasco and Mérida, 2007; Haucke and Di Paolo, 2007), events that occur in many physiological processes, such as exocytosis, endocytosis, membrane biogenesis, and cell division. Moreover Al-mediated inhibition of root growth was found to be connected with the inhibition of endocytosis (Illéš et al., 2006; Krtková et al., 2012) and controlled through local auxin biosynthesis and signaling (Shen et al., 2008; Yang et al., 2014). Not incurious, expression of NPC4 was increased and npc4 seedlings exhibit shorter primary root and smaller density of lateral roots after auxin treatment (Wimalasekera et al., 2010). Thus, it is worthwhile to note that the inhibition of DAG formation during Al stress might affect the mentioned processes and rapidly inhibit growth. On the other hand, our long-term experiment revealed that npc4 seedlings were more sensitive to Al stress while NPC4-OE seedlings formed a more abundant root system (Figure 7). This suggests that NPC4/DAG functions differently, more likely participating in lipid turnover and membrane remodeling, respectively.
In summary, our results suggest that the previously described involvement of NPC in response to Al stress is mediated by NPC4 in A. thaliana.
The authors declare that the research was conducted in the absence of any commercial or financial relationships that could be construed as a potential conflict of interest.
This work was supported by the Czech Science Foundation (GACR) grant no. P501/12/P950 to P.P. The authors thank Daniela Kocourková and Kateřina Raková for their excellent technical assistance.
BODIPY, 4,4-difluoro-4-bora-3a,4a-diaza-s-indacene; BY-2, bright yellow 2; DAG, diacylglycerol; GUS, β-glucuronidase; HP-TLC, high-performance thin-layer chromatography; MS, Murashige-Skoog; NPC, non-specific phospholipase C; PA, phosphatidic acid; PIP2, phosphatidylinositol 4,5-bisphosphate; PI-PLC, phosphatidylinositol-specific phospholipase C; PLD, phospholipase D; PM, plasma membrane
Andersson, M. X., Larsson, K. E., Tjellström, H., Liljenberg, C., and Sandelius, A. S. (2005). Phosphate-limited oat. The plasma membrane and the tonoplast as major targets for phospholipid-to-glycolipid replacement and stimulation of phospholipases in the plasma membrane. J. Biol. Chem. 280, 27578–27586. doi: 10.1074/jbc.M503273200
Pubmed Abstract | Pubmed Full Text | CrossRef Full Text | Google Scholar
Bargmann, B. O. R., Laxalt, A. M., Ter Riet, B., Schouten, E., van Leeuwen, W., Dekker, H. L.,et al. (2006). LePLDβ1 activation and relocalization in suspension-cultured tomato cells treated with xylanase. Plant J. 45, 358–368. doi: 10.1111/j.1365-313X.2005.02631.x
Pubmed Abstract | Pubmed Full Text | CrossRef Full Text | Google Scholar
Bargmann, B. O. R., Laxalt, A. M., Ter Riet, B., van Schooten, B., Merquiol, E., Testerink, C.,et al. (2009). Multiple PLDs required for high salinity and water deficit tolerance in plants. Plant Cell Physiol. 50, 78–89. doi: 10.1093/pcp/pcn173
Pubmed Abstract | Pubmed Full Text | CrossRef Full Text | Google Scholar
Boscolo, P. R. S., Menossi, M., and Jorge, R. A. (2003). Aluminum-induced oxidative stress in maize. Phytochemistry 62, 181–189. doi: 10.1016/S0031-9422(02)00491-0
Pubmed Abstract | Pubmed Full Text | CrossRef Full Text | Google Scholar
Carrasco, S., and Mérida, I. (2007). Diacylglycerol, when simplicity becomes complex. Trends Biochem. Sci. 32, 27–36. doi: 10.1016/j.tibs.2006.11.004
Pubmed Abstract | Pubmed Full Text | CrossRef Full Text | Google Scholar
Clough, S. J., and Bent, A. F. (1998). Floral dip: a simplified method for Agrobacterium-mediated transformation of Arabidopsis thaliana. Plant J. 16, 735–743. doi: 10.1046/j.1365-313x.1998.00343.x
Pubmed Abstract | Pubmed Full Text | CrossRef Full Text | Google Scholar
Dong, W., Lv, H., Xia, G., and Wang, M. (2012). Does diacylglycerol serve as a signaling molecule in plants? Plant Signal. Behav. 7, 1–4. doi: 10.4161/psb.19644
Pubmed Abstract | Pubmed Full Text | CrossRef Full Text | Google Scholar
Gaude, N., Nakamura, Y., Scheible, W. R., Ohta, H., and Dormann, P. (2008). Phospholipase C5 (NPC5) is involved in galactolipid accumulation during phosphate limitation in leaves of Arabidopsis. Plant J. 56, 28–39. doi: 10.1111/j.1365-313X.2008.03582.x
Pubmed Abstract | Pubmed Full Text | CrossRef Full Text | Google Scholar
Haucke, V., and Di Paolo, G. (2007). Lipids and lipid modifications in the regulation of membrane. Curr. Opin. Cell Biol. 19, 426–435. doi: 10.1016/j.ceb.2007.06.003
Pubmed Abstract | Pubmed Full Text | CrossRef Full Text | Google Scholar
Illéš, P., Schlicht, M., Pavlovkin, J., Lichtscheidl, I., Baluška, F., and Ovečka, M. (2006). Aluminium toxicity in plants: internalization of aluminium into cells of the transition zone in Arabidopsis root apices related to changes in plasma membrane potential, endosomal behaviour, and nitric oxide production. J. Exp. Bot. 57, 4201–4213. doi: 10.1093/jxb/erl197
Pubmed Abstract | Pubmed Full Text | CrossRef Full Text | Google Scholar
Jefferson, R. A., Kavanagh, T. A., and Bevan, M. W. (1987). GUS fusions: β-glucuronidase as a sensitive and versatile gene fusion marker in higher plants. EMBO J. 6, 3901–3907.
Johansson, O. N., Fahlberg, P., Karimi, E., Nilsson, A. K., Ellerström, M., and Andersson, M. X. (2014). Redundancy among phospholipase D isoforms in resistance triggered by recognition of the Pseudomonas syringae effector AvrRpm1 in Arabidopsis thaliana. Front. Plant Sci. 5:639. doi: 10.3389/fpls.2014.00639
Pubmed Abstract | Pubmed Full Text | CrossRef Full Text | Google Scholar
Jones, D. L., and Kochian, L. V. (1995). Aluminium inhibition of the inositol 1,4,5-trisphosphate signal transduction pathway in wheat roots: a role in aluminium toxicity? Plant Cell 7, 1913–1922. doi: 10.1105/tpc.7.11.1913
Pubmed Abstract | Pubmed Full Text | CrossRef Full Text | Google Scholar
Jones, D. L., and Kochian, L. V. (1997). Aluminum interaction with plasma membrane lipids and enzyme metal binding sites and its potential role in Al cytotoxicity. FEBS Lett. 400, 51–57. doi: 10.1016/S0014-5793(96)01319-1
Pubmed Abstract | Pubmed Full Text | CrossRef Full Text | Google Scholar
Klahre, U., Becker, C., Schmitt, A. C., and Kost, B. (2006). Nt-RhoGDI2 regulates Rac/Rop signaling and polar cell growth in tobacco pollen tubes. Plant J. 46, 1018–1031. doi: 10.1111/j.1365-313X.2006.02757.x
Pubmed Abstract | Pubmed Full Text | CrossRef Full Text | Google Scholar
Kocourková, D., Krčková, Z., Pejchar, P., Veselková,Š., Valentová, O., Wimalasekera, R.,et al. (2011). The phosphatidylcholine-hydrolyzing phospholipase C NPC4 plays a role in response of Arabidopsis roots to salt stress. J. Exp. Bot. 62, 3753–3763. doi: 10.1093/jxb/err039
Pubmed Abstract | Pubmed Full Text | CrossRef Full Text | Google Scholar
Kost, B., Spielhofer, P., and Chua, N.-H. (1998). A GFP-mouse talin fusion protein labels plant actin filaments in vivo and visualizes the actin cytoskeleton in growing pollen tubes. Plant J. 16, 393–401. doi: 10.1046/j.1365-313x.1998.00304.x
Pubmed Abstract | Pubmed Full Text | CrossRef Full Text | Google Scholar
Krtková, J., Havelková, L., Křepelová, A., Fišer, R., Vosolsobě, S., Novotná, Z.,et al. (2012). Loss of membrane fluidity and endocytosis inhibition are involved in rapid aluminum-induced root growth cessation in Arabidopsis thaliana. Plant Physiol. Biochem. 60, 88–97. doi: 10.1016/j.plaphy.2012.07.030
Pubmed Abstract | Pubmed Full Text | CrossRef Full Text | Google Scholar
Martínez-Estévez, M., Racagni-Di Palma, G., Muñoz-Sánchez, J. A., Brito-Argáez, L., Loyola-Vargas, V. M., and Hernández-Sotomayor, S. M. T. (2003). Aluminium differentially modifies lipid metabolism from the phosphoinositide pathway in Coffea arabica cells. J. Plant Physiol. 160, 1297–1303. doi: 10.1078/0176-1617–1168
Pubmed Abstract | Pubmed Full Text | CrossRef Full Text | Google Scholar
Matsumoto, H. (2000). Cell biology of aluminum toxicity and tolerance in higher plants. Int. Rev. Cytol. 200, 1–46. doi: 10.1016/S0074-7696(00)00001-2
Meijer, H. J. G., and Munnik, T. (2003). Phospholipid-based signaling in plants. Annu. Rev. Plant Biol. 54, 265–306. doi: 10.1146/annurev.arplant.54.031902.134748
Pubmed Abstract | Pubmed Full Text | CrossRef Full Text | Google Scholar
Munnik, T. (ed.). (2010). Lipid Signaling in Plants. Berlin: Springer. doi: 10.1007/978-3-642-03873-0
Nakagawa, T., Kurose, T., Hino, T., Tanaka, K., Kawamukai, M., Niwa, Y.,et al. (2007). Development of series of gateway binary vectors, pGWBs, for realizing efficient construction of fusion genes for plant transformation. J. Biosci. Bioeng. 104, 34–41. doi: 10.1263/jbb.104.34
Pubmed Abstract | Pubmed Full Text | CrossRef Full Text | Google Scholar
Nakamura, Y., Awai, K., Masuda, T., Yoshioka, Y., Takamiya, K., and Ohta, H. (2005). A novel phosphatidylcholine-hydrolyzing phospholipase C induced by phosphate starvation in Arabidopsis. J. Biol. Chem. 280, 7469–7476. doi: 10.1074/jbc.M408799200
Pubmed Abstract | Pubmed Full Text | CrossRef Full Text | Google Scholar
Panda, S. K., Baluška, F., and Matsumoto, H. (2009). Aluminum stress signaling in plants. Plant Signal. Behav. 4, 592–597. doi: 10.4161/psb.4.7.8903
Pejchar, P., Pleskot, R., Schwarzerová, K., Martinec, J., Valentová, O., and Novotná, Z. (2008). Aluminum ions inhibit phospholipase D in a microtubule-dependent manner. Cell Biol. Int. 32, 554–556. doi: 10.1016/j.cellbi.2007.11.008
Pubmed Abstract | Pubmed Full Text | CrossRef Full Text | Google Scholar
Pejchar, P., Potocký, M., Novotná, Z., Veselková,Š., Kocourková, D., Valentová, O.,et al. (2010). Aluminium ions inhibit formation of diacylglycerol generated by phosphatidylcholine-hydrolysing phospholipase C in tobacco cells. New Phytol. 188, 150–160. doi: 10.1111/j.1469-8137.2010.03349.x
Pubmed Abstract | Pubmed Full Text | CrossRef Full Text | Google Scholar
Pejchar, P., Scherer, G. F. E., and Martinec, J. (2013). Assaying nonspecific phospholipase C activity. Methods Mol. Biol. 1009, 193–203. doi: 10.1007/978-1-62703-401-2_18
Pubmed Abstract | Pubmed Full Text | CrossRef Full Text | Google Scholar
Peters, C., Kim, S.-C., Devaiah, S., Li, M., and Wang, X. (2014). Non-specific phospholipase C5 and diacylglycerol promote lateral root development under mild salt stress in Arabidopsis. Plant Cell Environ. 37, 2002–2013. doi: 10.1111/pce.12334
Pubmed Abstract | Pubmed Full Text | CrossRef Full Text | Google Scholar
Peters, C., Li, M. Y., Narasimhan, R., Roth, M., Welti, R., and Wang, X. M. (2010). Nonspecific phospholipase C NPC4 promotes responses to abscisic acid and tolerance to hyperosmotic stress in Arabidopsis. Plant Cell 22, 2642–2659. doi: 10.1105/tpc.109.071720
Pubmed Abstract | Pubmed Full Text | CrossRef Full Text | Google Scholar
Pleskot, R., Pejchar, P., Bezvoda, R., Lichtscheidl, I. K., Wolters-Arts, M., Marc, J.,et al. (2012). Turnover of phosphatidic acid through distinct signalling pathways affects multiple aspects of tobacco pollen tube tip growth. Front. Plant Sci. 3:54. doi: 10.3389/fpls.2012.00054
Pubmed Abstract | Pubmed Full Text | CrossRef Full Text | Google Scholar
Pokotylo, I., Kolesnikov, Y., Kravets, V., Zachowski, A., and Ruelland, E. (2014). Plant phosphoinositide-dependent phospholipases C: variations around a canonical theme. Biochimie 96, 144–157. doi: 10.1016/j.biochi.2013.07.004
Pubmed Abstract | Pubmed Full Text | CrossRef Full Text | Google Scholar
Pokotylo, I., Pejchar, P., Potocký, M., Kocourková, D., Krčková, Z., Ruelland, E.,et al. (2013). The plant non-specific phospholipase C gene family. Novel competitors in lipid signalling. Prog. Lipid Res. 52, 62–79. doi: 10.1016/j.plipres.2012.09.001
Pubmed Abstract | Pubmed Full Text | CrossRef Full Text | Google Scholar
Potocký, M., Pleskot, R., Pejchar, P., Vitale, N., Kost, B., and Žárský, V. (2014). Live-cell imaging of phosphatidic acid dynamics in pollen tubes visualized by Spo20p-derived biosensor. New Phytol. 203, 483–494. doi: 10.1111/nph.12814
Pubmed Abstract | Pubmed Full Text | CrossRef Full Text | Google Scholar
Ramos-Díaz, A., Brito-Argáez, L., Munnik, T., and Hernández-Sotomayor, S. (2007). Aluminum inhibits phosphatidic acid formation by blocking the phospholipase C pathway. Planta 225, 393–401. doi: 10.1007/s00425-006-0348-3
Pubmed Abstract | Pubmed Full Text | CrossRef Full Text | Google Scholar
Reddy, V. S., Rao, D. K. V., and Rajasekharan, R. (2010). Functional characterization of lysophosphatidic acid phosphatase from Arabidopsis thaliana. Biochim. Biophys. Acta 1801, 455–461. doi: 10.1016/j.bbalip.2009.12.005
Pubmed Abstract | Pubmed Full Text | CrossRef Full Text | Google Scholar
Rengel, Z., and Zhang, W. H. (2003). Role of dynamics of intracellular calcium in aluminium-toxicity syndrome. New Phytol. 159, 295–314. doi: 10.1046/j.1469-8137.2003.00821.x
Rietz, S., Dermendjiev, G., Oppermann, E., Tafesse, F. G., Effendi, Y., Holk, A.,et al. (2010). Roles of Arabidopsis patatin-related phospholipases A in root development are related to auxin responses and phosphate deficiency. Mol. Plant 3, 524–538. doi: 10.1093/mp/ssp109
Pubmed Abstract | Pubmed Full Text | CrossRef Full Text | Google Scholar
Ruíz-Herrera, L.-F., and López-Bucio, J. (2013). Aluminum induces low phosphate adaptive responses and modulates primary and lateral root growth by differentially affecting auxin signaling in Arabidopsis seedlings. Plant Soil 371, 593–609. doi: 10.1007/s11104-013-1722-0
Scherer, G. F. E., Paul, R. U., Holk, A., and Martinec, J. (2002). Down-regulation by elicitors of phosphatidylcholine-hydrolyzing phospholipase C and up-regulation of phospholipase A in plant cells. Biochem. Biophys. Res. Commun. 293, 766–770. doi: 10.1016/S0006-291X(02)00292-9
Pubmed Abstract | Pubmed Full Text | CrossRef Full Text | Google Scholar
Schwarzerová, K., Zelenková, S., Nick, P., and Opatrný, Z. (2002). Aluminum-induced rapid changes in the microtubular cytoskeleton of tobacco cell lines. Plant Cell Physiol. 43, 207–216. doi: 10.1093/pcp/pcf028
Pubmed Abstract | Pubmed Full Text | CrossRef Full Text | Google Scholar
Shen, H., Hou, N., Schlicht, M., Wan, Y., Mancuso, S., and Baluška, F. (2008). Aluminium toxicity targets PIN2 in Arabidopsis root apices: effects on PIN2 endocytosis, vesicular recycling, and polar auxin transport. Chin. Sci. Bull. 53, 2480–2487. doi: 10.1007/s11434-008-0332-3
Sivaguru, M., Baluška, F., Volkmann, D., Felle, H. H., and Horst, W. J. (1999). Impacts of aluminum on the cytoskeleton of the maize root apex. Short-term effects on the distal part of the transition zone. Plant Physiol. 119, 1073–1082. doi: 10.1104/pp.119.3.1073
Pubmed Abstract | Pubmed Full Text | CrossRef Full Text | Google Scholar
Sivaguru, M., Pike, S., Gassmann, W., and Baskin, T. I. (2003). Aluminum rapidly depolymerizes cortical microtubules and depolarizes the plasma membrane: evidence that these responses are mediated by a glutamate receptor. Plant Cell Physiol. 44, 667–675. doi: 10.1093/pcp/pcg094
Pubmed Abstract | Pubmed Full Text | CrossRef Full Text | Google Scholar
Tian, Q.-Y., Sun, D.-H., Zhao, M.-G., and Zhang, W.-H. (2007). Inhibition of nitric oxide synthase (NOS) underlies aluminum-induced inhibition of root elongation in Hibiscus moscheutos. New Phytol. 174, 322–331. doi: 10.1111/j.1469-8137.2007.02005.x
Pubmed Abstract | Pubmed Full Text | CrossRef Full Text | Google Scholar
Tjellström, H., Andersson, M. X., Larsson, K. L., and Sandelius, A. S. (2008). Membrane phospholipids as a phosphate reserve: the dynamic nature of phospholipid-to-digalactosyl diacylglycerol exchange in higher plants. Plant Cell Environ. 31, 1388–1398. doi: 10.1111/j.1365-3040.2008.01851.x
Pubmed Abstract | Pubmed Full Text | CrossRef Full Text | Google Scholar
Twell, D., Yamaguchi, J., Wing, R. A., Ushiba, J., and McCormick, S. (1991). Promoter analysis of genes that are coordinately expressed during pollen development reveals pollen-specific enhancer sequences and shared regulatory elements. Genes Dev. 5, 496–507. doi: 10.1101/gad.5.3.496
Pubmed Abstract | Pubmed Full Text | CrossRef Full Text | Google Scholar
Wang, C., Zien, C. A., Afitlhile, M., Welti, R., Hildebrand, D. F., and Wang, X. (2000). Involvement of phospholipase D in wound-induced accumulation of jasmonic acid in Arabidopsis. Plant Cell 12, 2237–2246. doi: 10.1105/tpc.12.11.2237
Pubmed Abstract | Pubmed Full Text | CrossRef Full Text | Google Scholar
Wang, X. (ed.). (2014). Phospholipases in Plant Signaling. Berlin: Springer-Verlag. doi: 10.1007/978-3-642-42011-5
Wimalasekera, R., Pejchar, P., Holk, A., Martinec, J., and Scherer, G. F. E. (2010). Plant phosphatidylcholine-hydrolyzing phospholipases C NPC3 and NPC4 with roles in root development and brassinolide signalling in Arabidopsis thaliana. Mol. Plant 3, 610–625. doi: 10.1093/mp/ssq005
Pubmed Abstract | Pubmed Full Text | CrossRef Full Text | Google Scholar
Wissemeier, A. H., and Horst, W. J. (1995). Effect of calcium supply on aluminium-induced callose formation, its distribution and persistence in roots of soybean (Glycine max (L.) Merr.). J. Plant Physiol. 145, 470–476. doi: 10.1016/S0176-1617(11)81773-6
Yang, Z. B., Geng, X., He, C., Zhang, F., Wang, R., Horst, W. J.,et al. (2014). TAA1-regulated local auxin biosynthesis in the root-apex transition zone mediates the aluminum-induced inhibition of root growth in Arabidopsis. Plant Cell 26, 2889–2904. doi: 10.1105/tpc.114.127993
Pubmed Abstract | Pubmed Full Text | CrossRef Full Text | Google Scholar
Zhao, J., Wang, C., Bedair, M., Welti, R. W., Sumner, L., Baxter, I.,et al. (2011). Suppression of phospholipase Dγs confers increased aluminum resistance in Arabidopsis thaliana. PLoS ONE 6:e28086. doi: 10.1371/journal.pone.0028086
Pubmed Abstract | Pubmed Full Text | CrossRef Full Text | Google Scholar
Keywords: aluminum toxicity, Arabidopsis, diacylglycerol, non-specific phospholipase C, plasma membrane, pollen tube, signaling, tobacco
Citation: Pejchar P, Potocký M, Krčková Z, Brouzdová J, Daněk M and Martinec J (2015) Non-specific phospholipase C4 mediates response to aluminum toxicity in Arabidopsis thaliana. Front. Plant Sci. 6:66. doi: 10.3389/fpls.2015.00066
Received: 22 December 2014; Accepted: 26 January 2015;
Published online: 16 February 2015.
Edited by:
Eric Ruelland, Centre National de la Recherche Scientifique, FranceReviewed by:
Frantisek Baluska, University of Bonn, GermanyCopyright © 2015 Pejchar, Potocký, Krčková, Brouzdová, Daněk and Martinec. This is an open-access article distributed under the terms of the Creative Commons Attribution License (CC BY). The use, distribution or reproduction in other forums is permitted, provided the original author(s) or licensor are credited and that the original publication in this journal is cited, in accordance with accepted academic practice. No use, distribution or reproduction is permitted which does not comply with these terms.
*Correspondence: Přemysl Pejchar, Laboratory of Signal Transduction, Institute of Experimental Botany, Academy of Sciences of the Czech Republic, v. v. i., Rozvojová 263, 16502 Prague 6 – Lysolaje, Czech Republic e-mail:cGVqY2hhckB1ZWIuY2FzLmN6
Disclaimer: All claims expressed in this article are solely those of the authors and do not necessarily represent those of their affiliated organizations, or those of the publisher, the editors and the reviewers. Any product that may be evaluated in this article or claim that may be made by its manufacturer is not guaranteed or endorsed by the publisher.
Research integrity at Frontiers
Learn more about the work of our research integrity team to safeguard the quality of each article we publish.