- 1Department of Plant Cell Biology, Institute of Cellular and Molecular Botany, University of Bonn, Bonn, Germany
- 2Department of Biological Sciences, Tokyo Metropolitan University, Tokyo, Japan
- 3Department of Pharmacy, University of Salerno, Fisciano, Italy
Despite growing underground, largely in darkness, roots emerge to be very sensitive to light. Recently, several important papers have been published which reveal that plant roots not only express all known light receptors but also that their growth, physiology and adaptive stress responses are light-sensitive. In Arabidopsis, illumination of roots speeds-up root growth via reactive oxygen species-mediated and F-actin dependent process. On the other hand, keeping Arabidopsis roots in darkness alters F-actin distribution, polar localization of PIN proteins as well as polar transport of auxin. Several signaling components activated by phytohormones are overlapping with light-related signaling cascade. We demonstrated that the sensitivity of roots to salinity is altered in the light-grown Arabidopsis roots. Particularly, light-exposed roots are less effective in their salt-avoidance behavior known as root halotropism. Here we discuss these new aspects of light-mediated root behavior from cellular, physiological and evolutionary perspectives.
Light as Important Environmental Factor for Roots
In nature, sessile plants have to respond to diurnal change in the light environment. One of main roles of light in plant’s life is to provide energy for photosynthesis and for the regulation of plant development at different stages such as seed germination, vegetative growth, tropisms and flowering. It is known that plant photoreceptors and related light-sensitive signaling molecules participate in the regulation of physiological conditions and morphological plasticity in response to the light environment. Darwin (1879) has discovered negative phototropism of plant roots. One year later, Francis Darwin and his father, Charles Darwin, published the book, “The Power of Movements in Plants.” They described both root and shoot tropisms. In addition, they also proposed that some form of long-distance signaling connect the sensory organ apices with the actively growing basal parts (Darwin, 1879, 1880). Since then, dedicated research work in plant physiology has discovered the long-distance signaling molecule, auxin, resulting in insights into plant photoreception. This directional growth response to incoming light is called phototropism. Positive phototropism, observed in shoots, is growth toward a light source; whereas negative phototropism, seen in roots, is bending away from the light source. We demonstrated that short (10 s), but strong (the photon flux was 82 μmol m-2 s-1), blue light illumination of Arabidopsis roots induces the immediate generation of reactive oxygen species (ROS) in root apex region, resulting in rapid increase of the root growth rate (Yokawa et al., 2011, 2013). This active response of light-stimulated root growth is termed escape tropism (Xu et al., 2013; Yokawa et al., 2013; for maize roots see Burbach et al., 2012). This tropism would allow Arabidopsis roots to escape from unfavorable light conditions if growing outside of our laboratories in the nature.
Photoreceptors in Roots
It has been shown that Arabidopsis plant expresses 14 photoreceptors, most of which are also present in roots (Briggs and Lin, 2012; Jeong and Choi, 2013; Briggs, 2014). Roots grow in the dark soil to anchor the plant and to absorb mineral nutrients and water. It has been reported that light can penetrate less than several millimeters due to the rather high absorbance of soil (Woolley and Stoller, 1978). Nevertheless, small cracks or mechanical impacts can often happen which allows light to penetrate deeper. For instance, roots may be exposed to light due to sudden temperature changes, earthquake, heavy rain, wind, and so on. In addition, it is very important for emerging radicle to increase the root growth rate shortly after seed germination on the ground. It was necessary to evolve the ability of roots to respond to environmental light when the first flowering plants with modern root system emerged in land plant evolution. In the next section, intriguing interplays between phytohormones and light-related signaling pathways will be discussed.
From Actin Cytoskeleton, via PIN2 Recycling, to Salt Avoidance Tropisms of Roots
At the cellular level, it was reported that PIN2 proteins (PIN-FORMED 2; auxin efflux carrier) in root apices respond to the light environment (Laxmi et al., 2008). Wan et al. (2012) demonstrated that the basipetal (shootward) PIN2-based polar auxin transport is subject to blue light control, which regulates the negative phototropism of Arabidopsis roots (Wan et al., 2012). Moreover, Dyachok et al. (2011) reported that light-activated COP1, E3 ubiquitin ligase, promotes actin polymerization and F-actin bundling, through regulation of the downstream ARP2/3-SCAR pathway in root cells. It results in increased root growth under the illuminated conditions (Dyachok et al., 2011). It was also reported that light controls bundling of F-actin in maize coleoptiles (Waller and Nick, 1997), changing sensitivity of cells to auxin, which is feeding back to control F-actin as well as cell growth (Nick et al., 2009). The interplays between F-actin and polar auxin transport mediated by endocytic vesicle recycling, especially in the transition zone of root apex, control root tropisms (Baluška et al., 1996, 2004, 2005, 2010; Baluška and Mancuso, 2013). Interestingly, precursor of endogenous auxin, indole-3-acetaldehyde (IAAld), is produced non-enzymatically in vitro by illumination of tryptophan in the presence of flavin which is abundant in living plant cells (Koshiba et al., 1993). Recently, we have proposed close links between the redox status and auxin (IAA) biosynthesis in plants (Yokawa et al., 2014). Taken together, it is obvious that roots are extraordinarily sensitive to light exposure due to their inherent evolutionary optimization for the underground life. Therefore, it is not surprising that illuminated roots of young Arabidopsis seedlings enhance their growth with the concomitant phototropism.
A few yeas ago, salt-stressed roots of Arabidopsis have been shown to alter root growth direction in order to avoid high salt areas via so-called salt avoidance tropism (Li and Zhang, 2008; Sun et al., 2008). This active root tropism requires ion gradient sensing pathway which would then control the PIN2 abundance, recycling and degradation (Li and Zhang, 2008; Sun et al., 2008). This unique Arabidopsis root behavior was linked to phospholipase D Zeta2 (PLDζ2) activity which stimulates clathrin-mediated endocytosis of PIN2, and this tropism was also termed root halotropism (Galvan-Ampudia et al., 2013; Rosquete and Kleine-Vehn, 2013; Pierik and Testerink, 2014). Interestingly, similarly, as in halotropism, PLDζ2 is involved in root hydrotropism through the PIN2-mediated suppression of root gravitropism (Taniguchi et al., 2010). Moreover, PLDζ2 is crucial for brefeldin A-sensitive endocytic recycling driving PIN2 recycling (Li and Xue, 2007) and polar auxin transport in the transition zone (Mancuso et al., 2007). Because PIN2 is crucial in this respect, as well as in adaptive responses of roots to light (Laxmi et al., 2008; Sassi et al., 2012; Wan et al., 2012), it is very important to test whether light condition affect the response of Arabidopsis roots to the salt stress.
Plant Hormones are Integrated with Light Signaling Pathways
Karrikin molecule was isolated from the smoke of combusted plant materials and found to potently stimulate the germination of plant seeds (Flematti et al., 2004). This compound was identified as 3-methyl-2H-furo[2,3-c]pyran-2-one, karrikin-1 or karrikinolide-1 (KAR1) shown in Figure 1A-a, and another analogs of karrikins (KAR2-KAR6) commonly possess butenolide structure. The Arabidopsis mutant kai2 lacking KAI2 genes insensitive to KAR1 triggered promotion of seed germination. KAI2 is thought to be a putative receptor of karrikins (Nelson et al., 2010; Waters et al., 2012). KAI2 is a member of α/β-hydrolase family and is also known as HYPOSENSITIVE TO LIGHT (HTL). Role of KAI2/HTL in light responses (Figure 1B) of roots will be described in the next section.
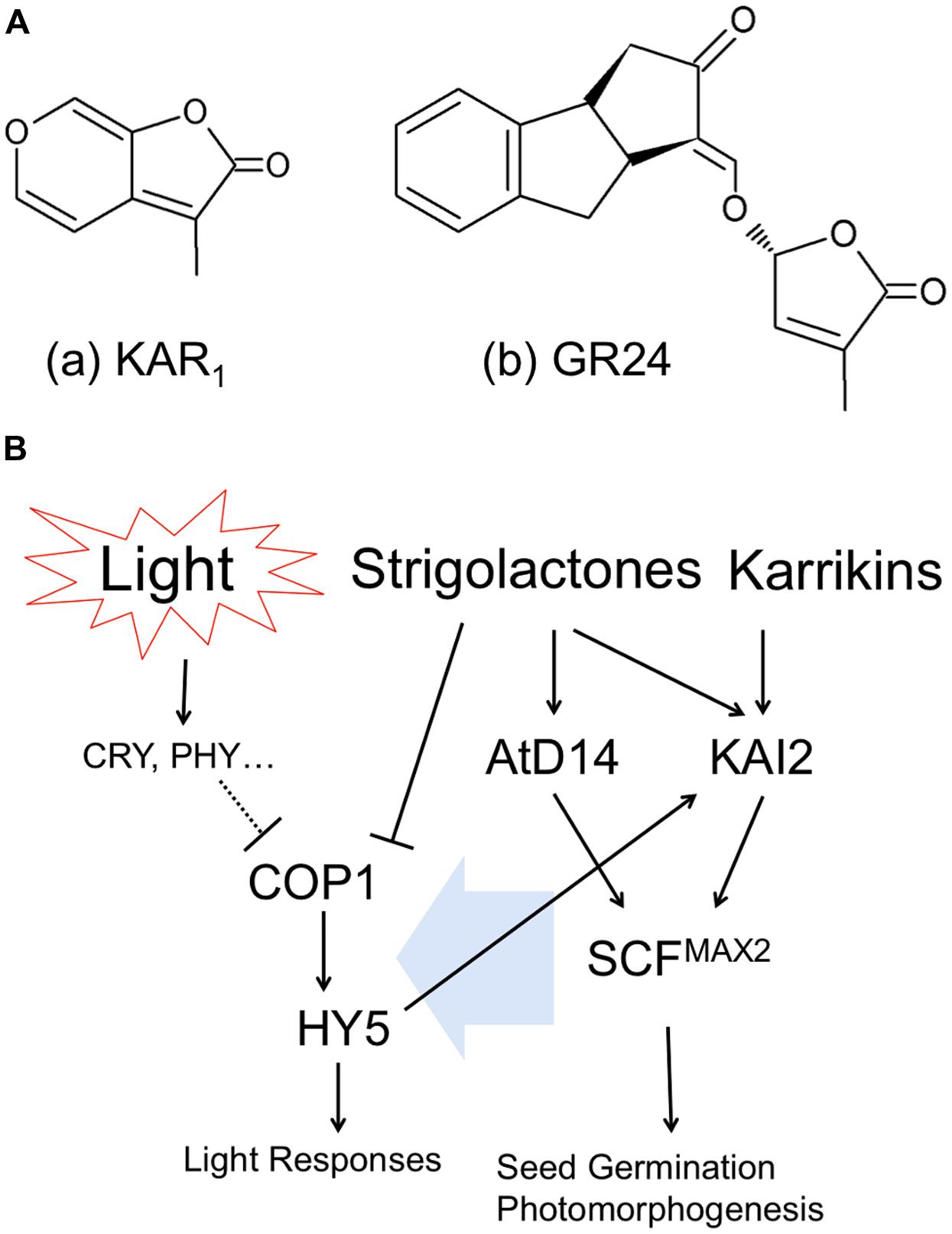
FIGURE 1. Cross talk between butenolide plant hormones and light signaling pathways. (A) Chemical structures of (a) Karrikin1, KAR1 and (b) Strigolactone analog, GR24. (B) Interaction of hormonal and light signaling pathways.
Arabidopsis thaliana AtD14 (ortholog of DWARF14) is a paralog of KAI2/HTL, which belongs to α/β-hydrolase family and plays a role in strigolactone perception (Waters et al., 2012). Strigolactones are synthesized from carotenoids and released into root exudates to promote germination of parasitic weeds (Yoneyama et al., 2007), as well as to stimulate hyphal branching in arbuscular mycorrhizal fungi (Akiyama et al., 2005). Strigolactones act also as a new class of plant hormone that inhibit the growth of axillary buds (Umehara et al., 2008) and alter root architecture (Koltai, 2011). Strigolactones are categorized as a sesquiterpenes lactone and have two moieties of butenolide (shown in Figure 1A-b as synthesized analog, GR24). As described above, karrikins have butenolide moieties as well. Therefore, because of the structural similarity of two molecules, karrikin receptor KAI2/HTL can respond to strigolactones. However, in contrast, the proposed strigolactone receptor AtD14 is insensitive to karrikins; indicating that the activity of KAI2/HTL as α/β-hydrolase is more flexible to detect butenolide structure than AtD14, probably due to evolutionary issues (Waters et al., 2012).
It was reported that F-box protein, MORE AXILLARY BRANCHES2 (MAX2), is located in the downstream of both KAI2/HTL and AtD14 and thus necessary for plants to respond to karrikin and strigolactone (Nelson et al., 2010; Waters et al., 2012). MAX2 forms SCF E3 ubiquitin ligase complex (Skp1, Cullin1, F-box), which modulates further downstream transcriptions (Stirnberg et al., 2007; Shen et al., 2012). However, molecular functions of KAI2/HTL or AtD14-mediated SCFMAX2 regulation of plant morphogenesis are not known yet. Interestingly in this respect, max2 mutants are hyper-sensitive to drought and osmotic stress, including high NaCl, mannitol, and glucose (Bu et al., 2014). Furthermore, strigolactones exert positive roles in plant adaptation to drought and salt stress (Ha et al., 2014). We will discuss these newly emerging aspects in the final section.
Light Signal Transduction via COP1 and HY5 is Integrated with Hormonal Signaling
In principle, plants recognize light as specific wavelengths by specific photoreceptors. Activated (excited) photoreceptors convert the light information into physiological signaling in diverse manners. CONSTITUTIVE PHOTOMORPHOGENIC1(COP1), E3 ubiquitin ligase, participates in the light-perceiving signaling cascade via affecting ubiquitination of target proteins. Once the activity of COP1 is inhibited, the downstream bZIP transcription factor, ELONGATED HYPOCOTYL5 (HY5) is freed from ubiquitination by COP1 and starts specific gene transcriptions related to light responses (Figure 1B left). COP1 and HY5 control root growth, lateral root formation, root hair tip growth, as well as root touch-responses and gravitropism (Oyama et al., 1997; Ang et al., 1998; Cluis et al., 2004; Datta et al., 2006, 2007; Sibout et al., 2006). Importantly, SALT TOLERANCE HOMOLOG2 (STH2) is interacting partner of COP1 and HY5 which controls roots and their anthocyanin levels (Datta et al., 2007).
Interestingly, exposure of plants to strigolactone inhibits the COP1 activity, suggesting that strigolactones can mimick light perception in plants (Tsuchiya et al., 2010). They also demonstrated that max2 mutant of rice produces excess of strigolactones, resulting in the inhibition of COP1 and expression of light-responsive genes (Tsuchiya et al., 2010). In addition, it was reported that max2 mutant is hypersensitive to red, far-red and blue light (Shen et al., 2007). Auxin interaction with the signaling pathway of strigolactones via MAX2 was already reported (Hayward et al., 2009). Finally, the key transcription factor of light signaling HY5 requires strigolactones in order to stimulate Arabidopsis seed germination during thermoinhibiton (Toh et al., 2012). Light also induces auxin biosynthesis via photoexcitation of flavins (Koshiba et al., 1993; Yokawa et al., 2014).
Karrikin receptor KAI2 is also known as HTL. KAI2/HTL expression was strongly increased by blue, red and far-red light and HY5 binds to the promoter region of HTL, indicating the expression of KAI2/HTL is regulated in response to light environment (Sun and Ni, 2011). Furthermore, KAI2/HTL is located downstream of HY5 (Figure 1B) and induced by light treatment (Sun and Ni, 2011). Meanwhile, it was reported that the treatment of seeds with KAR1 (chemical structure is shown in Figure 1A-a) improved light responses in germination and early development of seedlings (Nelson et al., 2010). It implies that karrikins can affect the array of gene expression regarding light responses.
Plant roots exposed to salinity stress increase abscisic acid (ABA) and ABA-related gene expressions (Raghavendra et al., 2010). ABA and strigolactones are synthesized from carotenoids and the levels of these two plant hormones are affecting each other (López-Ráez et al., 2010). Salt stress increases strigolactone levels in roots which then promote arbuscular mycorrhizal symbiosis, resulting in changing ABA contents and alleviating stress response (Aroca et al., 2013). Strigolactones, ABA and cytokinin signaling pathways are integrated to allow plants to cope with high salinity environment very effectively (Ha et al., 2014). Many players of the phytohormone signaling pathways are overlapping and integrating with light-response pathways.
Taken together, these findings suggest that hormonal and light signaling pathway utilizes same junction for plant morphogenesis as well as for adaptation to abiotic stresses. The signaling pathways proposed (Figure 1B) are highly integrated, interacting with each other and, obviously, they represent just a ‘tip of the iceberg.’ In the case of roots, we should consider light solely as information (not as source of energy) into our careful consideration for our understanding of plant photomorphogenesis. In the next session, we are discussing how the root sensitivity to salinity is altered via exposure of roots to light.
UV Light and UVR8 in Drought and Salinity Responses of Roots
It has been reported that the reduction of plant growth under water deficit is driven by the UV-B photoreceptor UV RESISTANCE LOCUS 8 (UVR8; Kliebenstein et al., 2002; Brown et al., 2005; Favory et al., 2009; Fasano et al., 2014). Fasano et al. (2014) previously shown that the UVR8 gene complements the osmosensitive yeast mutant mpk1 ppz1. The expression of UVR8 was found upregulated in Arabidopsis plants grown under salt or osmotic stress conditions. Furthermore, the ectopic expression of UVR8 causes pleiotropic effects on plant growth, such as a general reduction of plant organ size, leaves with smaller cells, reduced root growth, and the accumulation of flavonoids. This suggests that the UV-B morphogenic responses are enhanced in the UVR8-overexpressing plants grown under low levels of UV-B light. The growth defects of the UVR8-overexpressing plants are even more severe under osmotic and salt stress. In contrast, the inactivation of UVR8 expression does not affect shoot or root growth under standard or mild drought stress conditions. Thus, the hypersensitive response to osmotic stress of the 35SUVR8 plants is strictly UVR8-dependent (Fasano et al., 2014).
There are extensive evidences that osmotic stress as well as UV-B light impacts are more prominent on shoots than on roots. Under mild osmotic stress, roots continue to grow (Bartels and Sunkar, 2005; Pardo, 2010; Werner et al., 2010). Moreover, enhanced root growth was observed in transgenic plants with a higher drought or salt-stress tolerance (Bartels and Sunkar, 2005). Whereas the impact of osmotic stress on root development is well known (recently reviewed in Liu et al., 2014), effects of UV-B on root growth are poorly understood. In general UV-B reduces primary root growth of Arabidopsis seedling (Kim et al., 1998; Tong et al., 2008). In the adult plant, increased allocation of biomass to roots has been reported to occur under UV-B stress (Bussell et al., 2012).
Development of a larger root system is considered as a drought-avoidance strategy that plants adopt to improve the uptake of water and nutrients when their availability in the soil is limited (Fukai and Cooper, 1995; Liao et al., 2001; Sharp et al., 2004; Pierik and Testerink, 2014). The UV-B photoreceptor UVR8 is expressed in roots of wild type plants (Rizzini et al., 2011; reviewed in Yokawa and Baluška, 2014). As shown in Figure 2, UVR8::GFP is expressing in Arabidopsis roots and is transported into nuclei upon irradiation of roots with UV-B. Over-expression of UVR8 greatly reduces root growth under light exposure and aggravates their weaker performance under the osmotic stress (Fasano et al., 2014). In comparison to the control plants, the primary root and lateral root densities were 13 and 60%, respectively. This indicates that auxin-dependent lateral root growth was most hampered. Indeed the root-phenotypes of the 35S-UVR8 plants are reminiscent that one of auxin mutant (Zhao, 2010). The analysis of lateral roots showed that the number of lateral root primordia and emerged lateral roots of the 35S-UVR8 plants was 12 and 68%, respectively, reduced in comparison to the control plants (Fasano et al., 2014). Flavonoids accumulation was found to be 2.2-fold increased in the root of the UVR8-over-expressing plants, whereas the content of IAA-conjugates showed a tendency to decrease (Fasano et al., 2014). Thus, the defects in cell expansion of the 35S-UVR8 roots could be associated to the increased levels of flavonoids which, in turn, alter polar auxin transport and/or auxin homeostasis (e.g., Santelia et al., 2008).
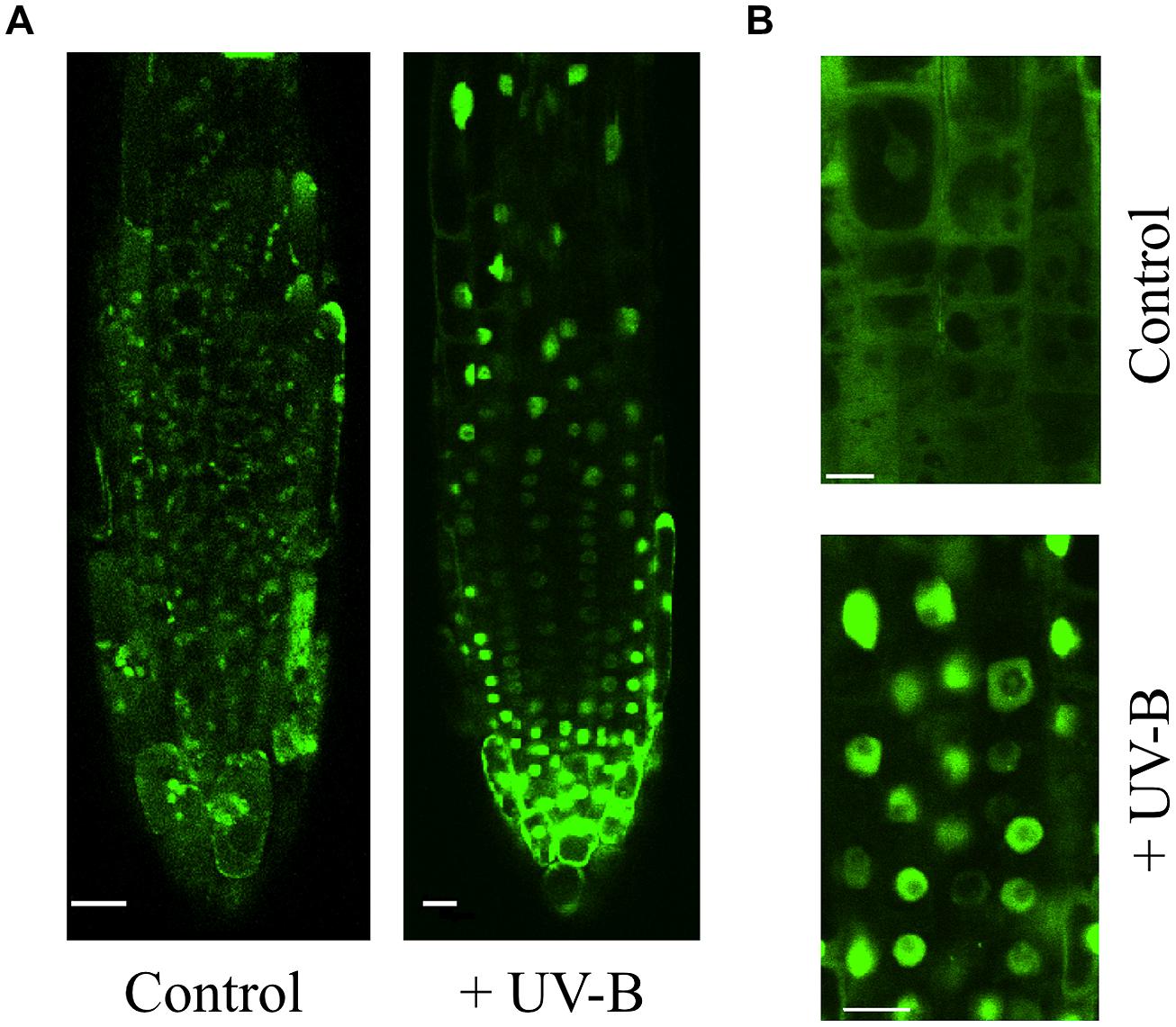
FIGURE 2. Relocation of UVR8::GFP to nuclei in Arabidopsis root cells. (A) Root apex region. (B) Magnified view of cells in the transition zone. Bar indicates 10 μm. Arabidopsis roots were exposed to 1 h UV-B treatment (3.9 μmol m-2 s-1).
Flavonoids are synthesized in roots, regulating root branching, gravitropism, and stress adaptation (Brown et al., 2001; Buer and Muday, 2004; Agati et al., 2011; Emiliani et al., 2013). Flavonoid concentrations are significantly higher in combined stress-treated plants than in those treated with UV-B alone (Hughes et al., 2010; Comont et al., 2012). Together, all these reports suggest that UVR8, via the control of flavonoid accumulation, might be a common intermediate in light and hormone signaling pathways to regulate root growth and development under abiotic stress challenges. Besides UV-B, drought and salinity have also been associated with anthocyanin accumulations in various tissues including roots (Agati et al., 2011; Emiliani et al., 2013; Meng, 2014). Importantly in this respect, the light-induced stimulation of Arabidopsis root growth is mediated via the COP1-mediated accumulation of anthocyanins (Meng, 2014).
Impact of Light on Salinity Avoidance via Root Halotropism
Although root apices growing in soil are known to be at a front line that can be exposed to salt stress, an impact of light on modulation of the sensitivity of roots to salinity has not been considered to date. Importantly, most experiments using the laboratory grown Arabidopsis seedlings are using roots exposed to light, although root apices outside in the nature are mostly in darkness.
As shown in the Figure 3, we have demonstrated that light exposure affects the root response to the salinity stress. Columbia WT seedlings were grown on agar plates containing NaCl only at their bottom parts. With this system, root tropism responses to salinity, halotropism, can be observed. Our methods followed the protocol described in (Galvan-Ampudia et al., 2013; Rosquete and Kleine-Vehn, 2013). After replacing bottom part of agar with the NaCl-agar, plastic dishes were covered with aluminum foil to allow dark treatment. Light/dark period was 16 h/8 h and light intensity was about the 120 μmol m-2 s-1, comparable to conditions in Galvan-Ampudia et al. (2013). Both the dark- and light-grown roots grew straight to the boundary of two agar media in control experiments with 0 mM NaCl (Figure 3). On the other hand, roots growing in darkness have higher sensitivity to NaCl than that roots exposed to light (Table 1). Dark-grown roots showed halotropism by avoiding salt-enriched agar areas at all concentrations of NaCl (Figure 3; Table 1). At the 100 mM concentration of NaCl, light-grown roots kept growing into the salty part of agar across the borderline between two agar parts even after several days. This suggests that light either prevents roots to accomplish the halotropism or that light lowers the sensitivity of Arabidopsis roots to perceive a gradient of salinity. The fact that light grown roots performed halotropism at higher concentrations of NaCl (Table 1) suggests that light interferes with the elusive root’s ability to sense the Na+ gradients (Maathuis, 2014). As root apices of roots growing outside in the nature are typically located deep in the soil in darkness, it can be expected that their halotropism is more efficient than for roots of laboratory grown seedlings.
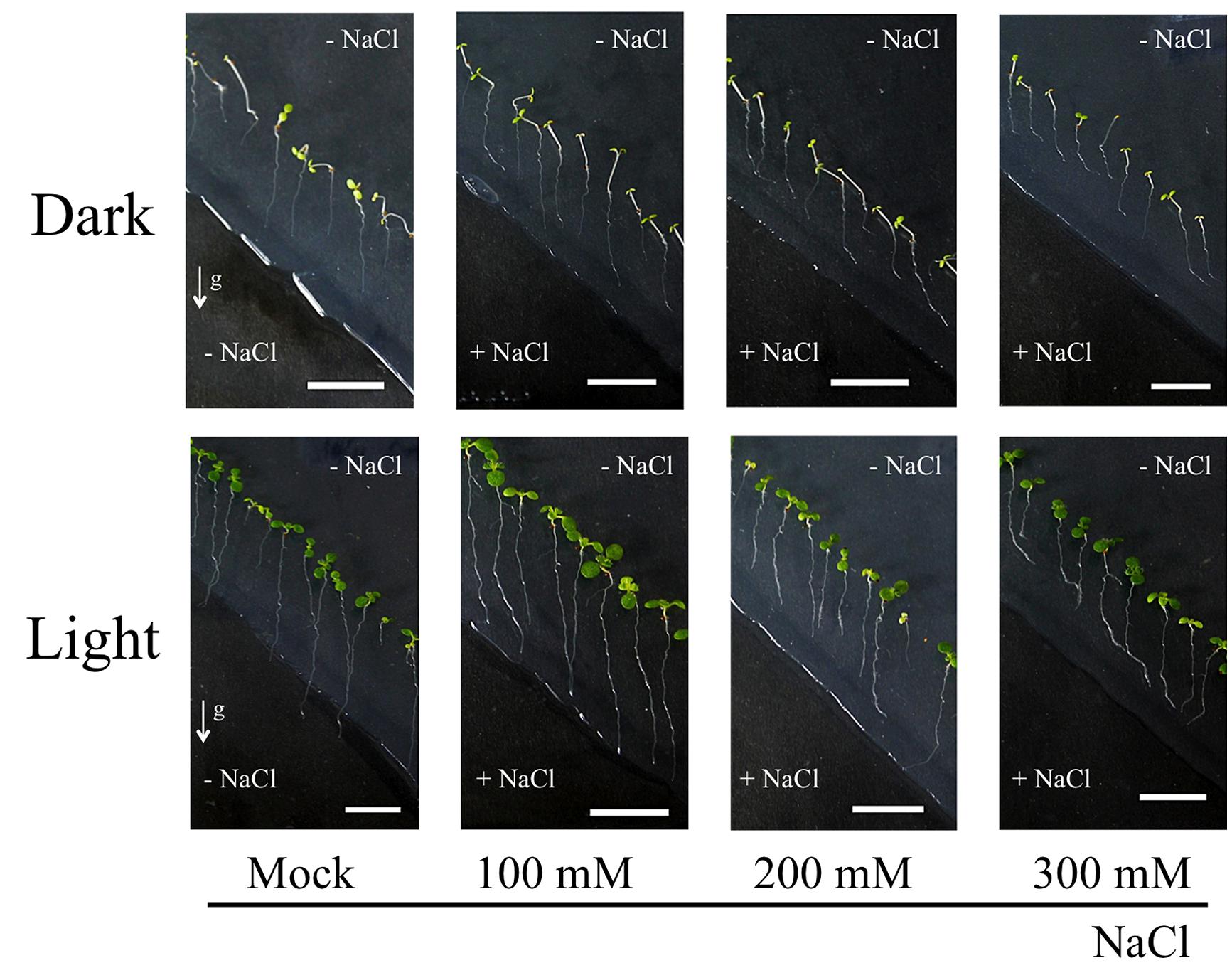
FIGURE 3. Light environment affects sensitivity of Arabidopsis roots to salinity. Seedlings of Col-0 were firstly grown on 1/2 MS media for 5 days. Bottom part of agar was removed diagonally and new 1/2 MS media containing NaCl was added. Petri dishes were placed either in light or dark condition for 3 days. Roots growing on agar plates in dark/light conditions with 0, 100, 200, and 300 mM NaCl media in the bottom half of Petri dishes. The method used for imposing the root halotropism is following exactly the protocol as published by Galvan-Ampudia et al. (2013). White arrows indicate the gravity vector. All seedlings were grown in vertical position. Bar indicates 1 cm.
Light-Induced Root Growth is Expensive and Alters Physiology and Morphology of Whole Seedlings
It is important to be aware that light-induced stimulation of root growth is changing the physiology of whole seedlings/plants. Figure 4 summarizes light-induced root growth mediated via COP1 interactions with the actin cytoskeleton (Dyachok et al., 2011) and anthocyanin biosynthesis (Meng, 2014). Both SCAR and PAP mediated pathways require sucrose as energy source (Kurata and Yamamoto, 1997; Kircher and Schopfer, 2012; Maier et al., 2013; Meng, 2014). Roots lacking ANGUSTIFOLIA3 (AN3) transcription coactivator have reduced anthocyanin levels and longer roots in light than WT roots (Meng, 2014). In contrast, the an3 mutant roots are shorter that WT roots when grown in darkness. Interestingly, AN3 binds to COP1 promoter to inhibit the light-induced root elongation (Meng, 2014). Besides anthocyanins, light-exposed roots show also rather dramatic increase of phenylpropanoid metabolism, inducing not only flavonoids but also monolignol glucosides (Hemm et al., 2004). Possible impacts of light on the Casparian bands formation in endodermis can be expected and easily tested.
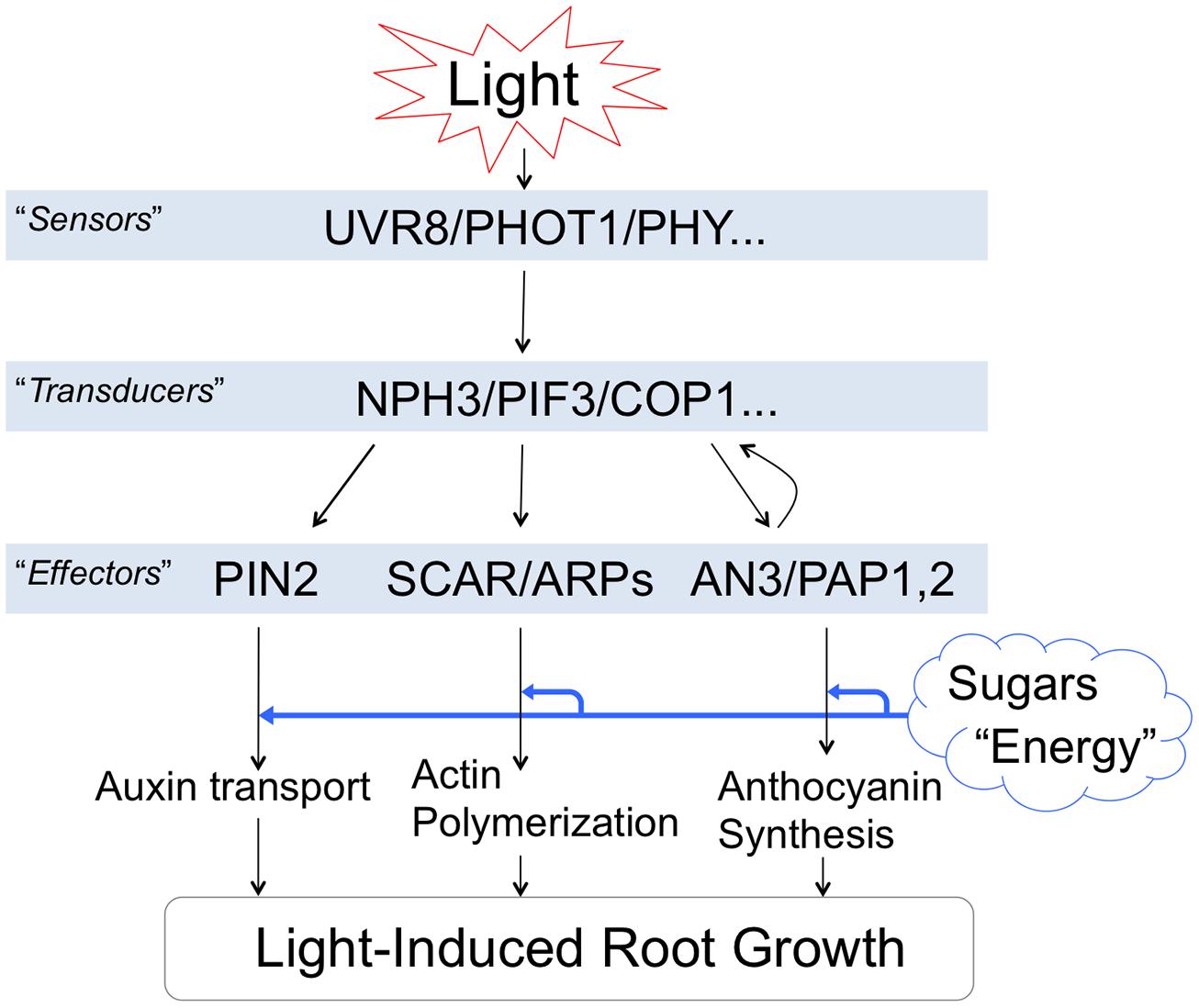
FIGURE 4. Light-induced root growth is expensive. Light-induced root elongation is mediated via COP1 interactions. Both SCAR and PAP mediated pathways require enough sucrose as an energy source. This figure is based on following references: Dyachok et al. (2011), Wan et al. (2012), Bai et al. (2014), Fasano et al. (2014), and Meng (2014).
Light-induced actin polymerization, auxin polar transport, root growth and anthocyanin biosynthesis are energetically demanding processes. The increased demand of sucrose for the root photomorphogenesis (Costigan et al., 2011; Warnasooriya and Montgomery, 2011; Yokawa et al., 2013) is going at the expense of other potential sinks of the plant body (Kurata and Yamamoto, 1997; Yazdanbakhsh et al., 2011; Kircher and Schopfer, 2012). Moreover, salt stress perceived locally at the root apex is rapidly spread throughout the plant body via systemic signaling (Choi et al., 2014; Gilroy et al., 2014). Therefore, it is important to be aware that illumination of roots has rather dramatic consequences for the whole seedling’s physiology and morphology.
Conclusions and Perspectives
Our results using salt stress reveal that light exposed roots show different behavior and responses under salt stress. One possible scenario, based on reports that illuminated roots enhance strigolactone levels (Koltai et al., 2011) and strigolactone increases drought and salt tolerance of roots (Ha et al., 2014), is that the light-exposed roots are less sensitive to salt stress. Whereas the dark-grown roots show halotropism (salt avoidance tropism) by growing around the salt-enriched agar parts, the light-exposed roots grow into the salt-enriched agar if NaCl levels are not too high (Tables 1 and 2). As light-induced strigolactone levels modify also root responses to low phosphate (Mayzlish-Gati et al., 2012), one can propose that exposure of Arabidopsis roots to light is modifying their whole physiology. In fact, our preliminary data reveal that Arabidopsis roots exposed to light show changes the sensitivity also to the aluminum toxicity (Kagenishi et al., in preparation). In addition, IAA levels in light exposed (1 h) maize roots were more than doubled compared to control levels (Yokawa et al., in preparation). It is intriguing that enhanced strigolactone levels resemble light-induced effects on roots. It was shown that light impacts on the actin cytoskeleton, also increased abundance and recycling of PIN2 auxin transporter (Laxmi et al., 2008; Dyachok et al., 2011; Wan et al., 2012; Pandya-Kumar et al., 2014). Moreover, salt stress, drought stress, cold stress, alkaline stress, aluminum toxicity; all these challenges target the PIN2 auxin transporter which is expressed in the root apex transition zone (discussed in Baluška et al., 2010; Baluška and Mancuso, 2013). In order to avoid light exposure of roots, we recommend using of the partially darkened Petri dishes (Yokawa et al., 2011, 2013; Xu et al., 2013). This is important as stressing roots with light is modifying not only root responses to diverse stresses but also the overall physiology of such seedlings. For example, it has been reported that circadian rhythms in Arabidopsis roots are governed by the shoot part (James et al., 2008). Importantly in this respect, ROS signaling and homeostasis was shown to be a major modulator of circadian rhythms both in prokaryotes and eukaryotes (Edgar et al., 2012; Lai et al., 2012; Stangherlin and Reddy, 2013). It is possible that seedlings will change even their circadian clock when roots are illuminated.
Although plant roots are heterotrophic plant organs, they require active suppression of photosynthetic gene expression. Tyrosylprotein sulfotransferase (TPST) protein HPS7 emerged recently as crucial player controlling this active suppression (Kang et al., 2014). Interestingly enough, hsp7 mutant roots show enhanced photosynthesis-related effects under phosphate deficiency stress. Previous studies identified Golden-Like transcription factors GLK1 and GLK2 involved in activation of photosynthetic genes in roots (Waters et al., 2009; Kobayashi et al., 2013). Both hsp7 and GLK-ox mutant roots do not show stunted root growth and any other phenotypes when grown in darkness (Kang et al., 2014).
Finally, the plant physiology perspective of the light-induced root growth in Arabidopsis is considering the high root growth rate as a sign of optimal root growth conditions. In stark contrast, the plant neurobiology interpretation of this increased root growth rate is that the light-exposed roots are experiencing stress and that such stressed roots are trying to escape from this unfavorable situation (Yokawa et al., 2011, 2013; Xu et al., 2013). Alpi et al. (2007), critics of the plant neurobiology initiative claimed that ‘… plant neurobiology does not add to our understanding of plant physiology, plant cell biology or signaling.’ Now, some 7 years later, it is getting obvious that plants and their roots are behaviorally much more complex than envisioned in the framework of classical plant physiology (Trewavas, 2005, 2009, 2014; Trewavas and Baluška, 2011; Baluška and Mancuso, 2013; Pierik and Testerink, 2014). Illumination of the roots is common laboratory praxis ever since Arabidopsis thaliana was introduced as model organism to plant sciences. However, it is important to be aware that light exposure of roots emerges as stress factor for the laboratory grown Arabidopsis seedlings. This is clear example for the usefulness of viewing plants as actively living and sensitive organisms solving their own plant-specific problems in intelligent manner.
Conflict of Interest Statement
The authors declare that the research was conducted in the absence of any commercial or financial relationships that could be construed as a potential conflict of interest.
Acknowledgments
Ken Yokawa was supported by the JSPS (Japanese Society for the Promotion of Science) Postdoctoral Fellowship. We thank Prof. Gareth I. Jenkins (University of Glasgow) for providing us with seeds of the UVR8::GFP line.
References
Agati, G., Biricolti, S., Guidi, L., Ferrini, F., Fini, A., and Tattini, M. (2011). The biosynthesis of flavonoids is enhanced similarly by UV radiation and root zone salinity in L. vulgare leaves. J. Plant Physiol. 168, 204–212. doi: 10.1016/j.jplph.2010.07.016
Pubmed Abstract | Pubmed Full Text | CrossRef Full Text | Google Scholar
Akiyama, K., Matsuzaki, K., and Hayashi, H. (2005). Plant sesquiterpenes induce hyphal branching in arbuscular mycorrhizal fungi. Nature 435, 824–827. doi: 10.1038/nature03608
Pubmed Abstract | Pubmed Full Text | CrossRef Full Text | Google Scholar
Alpi, A., Amrhein, N., Bertl, A., Blatt, M. R., Blumwald, E., Cervone, F.,et al. (2007). Plant neurobiology: no brain, no gain? Trends Plant Sci. 12, 135–136. doi: 10.1016/j.tplants.2007.03.002
Pubmed Abstract | Pubmed Full Text | CrossRef Full Text | Google Scholar
Ang, L. H., Chattopadhyay, S., Wei, N., Oyama, T., Okada, K., Batschauer, A.,et al. (1998). Molecular interaction between COP1 and HY5 defines a regulatory switch for light control of Arabidopsis development. Mol. Cell 1, 213–222. doi: 10.1016/S1097-2765(00)80022-2
Pubmed Abstract | Pubmed Full Text | CrossRef Full Text | Google Scholar
Aroca, R., Ruiz-Lozano, J. M., Zamarreño, A. M., Paz, J. A., García-Mina, J. M., Pozo, M. J.,et al. (2013). Arbuscular mycorrhizal symbiosis influences strigolactone production under salinity and alleviates salt stress in lettuce plants. J. Plant Physiol. 170, 47–55. doi: 10.1016/j.jplph.2012.08.020
Pubmed Abstract | Pubmed Full Text | CrossRef Full Text | Google Scholar
Bai, S., Yao, T., Li, M., Guo, X., Zhang, Y., Zhu, S.,et al. (2014). PIF3 is involved in the primary root growth inhibition of Arabidopsis induced by nitric oxide in the light. Mol. Plant 7, 616–625. doi: 10.1093/mp/sst142
Pubmed Abstract | Pubmed Full Text | CrossRef Full Text | Google Scholar
Baluška, F., and Mancuso, S. (2013). Root apex transition zone as oscillatory zone. Front. Plant Sci. 4:354. doi: 10.3389/fpls.2013.00354
Pubmed Abstract | Pubmed Full Text | CrossRef Full Text | Google Scholar
Baluška, F., Mancuso, S., Volkmann, D., and Barlow, P. W. (2004). Root apices as plant command centres: the unique ‘brain-like’ status of the root apex transition zone. Biologia 59, 9–17.
Baluška, F., Mancuso, S., Volkmann, D., and Barlow, P. W. (2010). Root apex transition zone: a signalling – response nexus in the root. Trends Plant Sci. 15, 402–408. doi: 10.1016/j.tplants.2010.04.007
Pubmed Abstract | Pubmed Full Text | CrossRef Full Text | Google Scholar
Baluška, F., Volkmann, D., and Barlow, P. W. (1996). Specialized zones of development in roots: view from the cellular level. Plant Physiol. 112, 3–4.
Baluška, F., Volkmann, D., and Menzel, D. (2005). Plant synapses: actin-based domains for cell-to-cell communication. Trends Plant Sci. 10, 106–111. doi: 10.1016/j.tplants.2005.01.002
Pubmed Abstract | Pubmed Full Text | CrossRef Full Text | Google Scholar
Bartels, D., and Sunkar, R. (2005). Drought and salt tolerance in plants. Crit. Rev. Plant Sci. 24, 23–58. doi: 10.1080/07352680590910410
Briggs, W. R. (2014). Phototropism: some history, some puzzles, and a look ahead. Plant Physiol. 164, 13–23. doi: 10.1104/pp.113.230573
Pubmed Abstract | Pubmed Full Text | CrossRef Full Text | Google Scholar
Briggs, W., and Lin, C. T. (2012). Photomorphogenesis – from one photoreceptor to 14: 40 years of progress. Mol. Plant 3, 531–532. doi: 10.1093/mp/sss059
Pubmed Abstract | Pubmed Full Text | CrossRef Full Text | Google Scholar
Brown, B. A., Cloix, C., Jiang, G. H., Kaiserli, E., Herzyk, P., Kliebenstein, D. J.,et al. (2005). A UV-B-specific signaling component orchestrates plant UV protection. Proc. Natl. Acad. Sci. U.S.A. 102, 18225–18230. doi: 10.1073/pnas.0507187102
Pubmed Abstract | Pubmed Full Text | CrossRef Full Text | Google Scholar
Brown, D. E., Rashotte, A. M., Murphy, A. S., Normanly, J., Tague, B. W., Peer, W. A.,et al. (2001). Flavonoids act as negative regulators of auxin transport in vivo in Arabidopsis. Plant Physiol. 126, 524–535. doi: 10.1104/pp.126.2.524
Pubmed Abstract | Pubmed Full Text | CrossRef Full Text | Google Scholar
Bu, Q., Lv, T., Shen, H., Luong, P., Wang, J., Wang, Z.,et al. (2014). Regulation of drought tolerance by the F-box protein MAX2 in Arabidopsis. Plant Physiol. 164, 424–439. doi: 10.1104/pp.113.226837
Pubmed Abstract | Pubmed Full Text | CrossRef Full Text | Google Scholar
Buer, C. S., and Muday, G. K. (2004). The transparent testa4 mutation prevents flavonoid synthesis and alters auxin transport and the response of Arabidopsis roots to gravity and light. Plant Cell 16, 1191–1205. doi: 10.1105/tpc.020313
Pubmed Abstract | Pubmed Full Text | CrossRef Full Text | Google Scholar
Burbach, C., Markus, K., Zhang, Y., Schlicht, M., and Baluška, F. (2012). Photophobic behavior of maize roots. Plant Signal. Behav. 7, 874–878. doi: 10.4161/psb.21012
Pubmed Abstract | Pubmed Full Text | CrossRef Full Text | Google Scholar
Bussell, J. S., Gwynn-Jones, D., Griffith, G. W., and Scullion, J. (2012). Above- and below-ground responses of Calamagrostis purpurea to UV-B radiation and elevated CO2 under phosphorus limitation. Physiol. Plant. 145, 619–628. doi: 10.1111/j.1399-3054.2012.01595.x
Pubmed Abstract | Pubmed Full Text | CrossRef Full Text | Google Scholar
Choi, W. G., Toyota, M., Kim, S. H., Hilleary, R., and Gilroy, S. (2014). Salt stress-induced Ca2+ waves are associated with rapid, long-distance root-to-shoot signaling in plants. Proc. Natl. Acad. Sci. U.S.A. 111, 6497–6502. doi: 10.1073/pnas.1319955111
Pubmed Abstract | Pubmed Full Text | CrossRef Full Text | Google Scholar
Cluis, C. P., Mouchel, C. F., and Hardtke, C. S. (2004). The Arabidopsis transcription factor HY5 integrates light and hormone signaling pathways. Plant J. 38, 332–347. doi: 10.1111/j.1365-313X.2004.02052.x
Pubmed Abstract | Pubmed Full Text | CrossRef Full Text | Google Scholar
Comont, D., Winters, A., and Gwynn-Jones, D. (2012). Acclimation and interaction between drought and elevated UV-B in A. thaliana: differences in response over treatment, recovery and reproduction. Ecol. Evol. 2, 2695–2709. doi: 10.1002/ece3.387
Pubmed Abstract | Pubmed Full Text | CrossRef Full Text | Google Scholar
Costigan, S. E., Warnasooriya, S. N., Humphries, B. A., and Montgomery, B. L. (2011). Root-localized phytochrome chromophore synthesis is required for photoregulation of root elongation and impacts root sensitivity to jasmonic acid in Arabidopsis. Plant Physiol. 157, 1138–1150. doi: 10.1104/pp.111.184689
Pubmed Abstract | Pubmed Full Text | CrossRef Full Text | Google Scholar
Darwin, F. (1879). Über das negativ wachstum heliotropischer wurzeln im licht und im finstern. Arb. D. Bot. Inst. Würzburg 2, 521–528.
Datta, S., Hettiarachchi, C., Johansson, H., and Holm, M. (2007). SALT TOLERANCE HOMOLOG2, a B-box protein in Arabidopsis that activates transcription and positively regulates light-mediated development. Plant Cell 19, 3242–3255. doi: 10.1105/tpc.107.054791
Pubmed Abstract | Pubmed Full Text | CrossRef Full Text | Google Scholar
Datta, S., Hettiarachchi, G. H., Deng, X. W., and Holm, M. (2006). Arabidopsis CONSTANS-LIKE3 is a positive regulator of red light signaling and root growth. Plant Cell 18, 70–84. doi: 10.1105/tpc.105.038182
Pubmed Abstract | Pubmed Full Text | CrossRef Full Text | Google Scholar
Dyachok, J., Zhu, L., Liao, F., He, J., Huq, E., and Blancaflor, E. B. (2011). SCAR mediates light-induced root elongation in Arabidopsis through photoreceptors and proteasomes. Plant Cell 23, 3610–3626. doi: 10.1105/tpc.111.088823
Pubmed Abstract | Pubmed Full Text | CrossRef Full Text | Google Scholar
Edgar, R. S., Green, E. W., Zhao, Y., van Ooijen, G., Olmedo, M., Qin, X.,et al. (2012). Peroxiredoxins are conserved markers of circadian rhythms. Nature 485, 459–464. doi: 10.1038/nature11088
Pubmed Abstract | Pubmed Full Text | CrossRef Full Text | Google Scholar
Emiliani, J., Grotewold, E., Falcone Ferreyra, M. L., and Casati, P. (2013). Flavonols protect Arabidopsis plants against UV-B deleterious effects. Mol. Plant 6, 1376–1379. doi: 10.1093/mp/sst021
Pubmed Abstract | Pubmed Full Text | CrossRef Full Text | Google Scholar
Fasano, R., Gonzalez, N., Tosco, A., Piaz, F. D., Docimo, T., Serrano, R.,et al. (2014). Role of Arabidopsis–UV RESISTANCE LOCUS 8 in plant growth reduction under osmotic stress and low levels of UV-B. Mol. Plant 5, 773–791. doi: 10.1093/mp/ssu002
Pubmed Abstract | Pubmed Full Text | CrossRef Full Text | Google Scholar
Favory, J. J., Stec, A., Gruber, H., Rizzini, L., Oravecz, A., Funk, M.,et al. (2009). Interaction of COP1 and UVR8 regulates UV-B-induced photomorphogenesis and stress acclimation in Arabidopsis. EMBO J. 28, 591–601. doi: 10.1038/emboj.2009.4
Pubmed Abstract | Pubmed Full Text | CrossRef Full Text | Google Scholar
Flematti, G. R., Ghisalberti, E. L., Dixon, K. W., and Trengove, R. D. (2004). A compound from smoke that promotes seed germination. Science 305:977. doi: 10.1126/science.1099944
Pubmed Abstract | Pubmed Full Text | CrossRef Full Text | Google Scholar
Fukai, S., and Cooper, M. (1995). Development of drought-resistant cultivars using physio-morphological traits in rice. Field Crops Res. 40, 67–86. doi: 10.1016/0378-4290(94)00096-U
Galvan-Ampudia, C. S., Julkowska, M. M., Darwish, E., Gandullo, J., Korver, R. A., Brunoud, G.,et al. (2013). Halotropism is a response of plant roots to avoid a saline environment. Curr. Biol. 23, 2044–2050. doi: 10.1016/j.cub.2013.08.042
Pubmed Abstract | Pubmed Full Text | CrossRef Full Text | Google Scholar
Gilroy, S., Suzuki, N., Miller, G., Choi, W. G., Toyota, M., Devireddy, A. R.,et al. (2014). A tidal wave of signals: calcium and ROS at the forefront of rapid systemic signaling. Trends Plant Sci. 19, 623–630. doi: 10.1016/j.tplants.2014.06.013
Pubmed Abstract | Pubmed Full Text | CrossRef Full Text | Google Scholar
Guo, Y., Zheng, Z., La Clair, J. J., Chory, J., and Noel, J. P. (2013). Smoke-derived karrikin perception by the α/β-hydrolase KAI2 from Arabidopsis. Proc. Natl. Acad. Sci. U.S.A. 110, 8284–8289. doi: 10.1073/pnas.1306265110
Pubmed Abstract | Pubmed Full Text | CrossRef Full Text | Google Scholar
Ha, C. V., Leyva-González, M. A., Osakabe, Y., Tran, U. T., Nishiyama, R., Watanabe, Y.,et al. (2014). Positive regulatory role of strigolactone in plant responses to drought and salt stress. Proc. Natl. Acad. Sci. U.S.A. 111, 851–856. doi: 10.1073/pnas.1322135111
Pubmed Abstract | Pubmed Full Text | CrossRef Full Text | Google Scholar
Hayward, A., Stirnberg, P., Beveridge, C., and Leyser, O. (2009). Interactions between auxin and strigolactone in shoot branching control. Plant Physiol. 151, 400–412. doi: 10.1104/pp.109.137646
Pubmed Abstract | Pubmed Full Text | CrossRef Full Text | Google Scholar
Hemm, M. R., Rider, S. D., Ogas, J., Murry, D. J., and Chapple, C. (2004). Light induces phenylpropanoid metabolism in Arabidopsis roots. Plant J. 38, 765–778. doi: 10.1111/j.1365-313X.2004.02089.x
Pubmed Abstract | Pubmed Full Text | CrossRef Full Text | Google Scholar
Hughes, N. M., Reinhardt, K., Feild, T. S., Gerardi, A. R., and Smith, W. K. (2010). Association between winter anthocyanin production and drought stress in angiosperm evergreen species. J. Exp. Bot. 61, 1699–1709. doi: 10.1093/jxb/erq042
Pubmed Abstract | Pubmed Full Text | CrossRef Full Text | Google Scholar
James, A. B., Monreal, J. A., Nimmo, G. A., Kelly, C. L., Herzyk, P., Jenkins, G. I.,et al. (2008). The circadian clock in Arabidopsis roots is a simplified slave version of the clock in shoots. Science 322, 1832–1835. doi: 10.1126/science.1161403
Pubmed Abstract | Pubmed Full Text | CrossRef Full Text | Google Scholar
Jeong, J., and Choi, G. (2013). Phytochrome-interacting factors have both shared and distinct biological roles. Mol. Cells 35, 371–380. doi: 10.1007/s10059-013-0135-5
Pubmed Abstract | Pubmed Full Text | CrossRef Full Text | Google Scholar
Kang, J., Yu, H., Tian, C., Zhou, W., Li, C., Jiao, Y.,et al. (2014). Suppression of photosynthetic gene expression in roots is required for sustained root growth under phosphate deficiency. Plant Physiol. 165, 1156–1170. doi: 10.1104/pp.114.238725
Pubmed Abstract | Pubmed Full Text | CrossRef Full Text | Google Scholar
Kim, B. C., Tennessen, D. J., and Last, R. L. (1998). UV-B-induced photomorphogenesis in Arabidopsis thaliana. Plant J. 15, 667–674. doi: 10.1046/j.1365-313x.1998.00246.x
Pubmed Abstract | Pubmed Full Text | CrossRef Full Text | Google Scholar
Kircher, S., and Schopfer, P. (2012). Photosynthetic sucrose acts as cotyledon-derived long-distance signal to control root growth during early seedling development in Arabidopsis. Proc. Natl. Acad. Sci. U.S.A. 109, 11217–11221. doi: 10.1073/pnas.1203746109
Pubmed Abstract | Pubmed Full Text | CrossRef Full Text | Google Scholar
Kliebenstein, D. J., Lim, J. E., Landry, L. G., and Last, R. L. (2002). Arabidopsis UVR8 regulates ultraviolet-B signal transduction and tolerance and contains sequence similarity to human regulator of chromatin condensation. Plant Physiol. 130, 234–243. doi: 10.1104/pp.005041
Pubmed Abstract | Pubmed Full Text | CrossRef Full Text | Google Scholar
Kobayashi, K., Sasaki, D., Noguchi, K., Fujinuma, D., Komatsu, H., Kobayashi, M.,et al. (2013). Photosynthesis of root chloroplasts developed in Arabidopsis lines overexpressing GOLDEN2-LIKE transcription factors. Plant Cell Physiol. 54, 1365–1377. doi: 10.1093/pcp/pct086
Pubmed Abstract | Pubmed Full Text | CrossRef Full Text | Google Scholar
Koltai, H. (2011). Strigolactones are regulators of root development. New Phytol. 190, 545–549. doi: 10.1111/j.1469-8137.2011.03678.x
Koltai, H., Cohen, M., Chesin, O., Mayzlish-Gati, E., Bécard, G., Puech, V.,et al. (2011). Light is a positive regulator of strigolactone levels in tomato roots. J. Plant Physiol. 168, 1993–1996. doi: 10.1016/j.jplph.2011.05.022
Pubmed Abstract | Pubmed Full Text | CrossRef Full Text | Google Scholar
Koshiba, T., Yamauchi, K., Matsuyama, H., Miyakado, M., Sori, I., and Satô, M. (1993). Flavin-photosensitized production of indole-3-acetaldehyde from tryptophan. Tetrahedron Lett. 34, 7603–7606. doi: 10.1016/S0040-4039(00)60411-2
Kurata, T., and Yamamoto, K. T. (1997). Light stimulated root elongation in Arabidopsis thaliana. J. Plant Physiol. 151, 346–351. doi: 10.1016/S0176-1617(97)80263-5
Lai, A. G., Doherty, C. J., Mueller-Roeber, B., Kay, S. A., Schippers, J. H., and Dijkwel, P. P. (2012). CIRCADIAN CLOCK-ASSOCIATED 1 regulates ROS homeostasis and oxidative stress responses. Proc. Natl. Acad. Sci. U.S.A. 109, 17129–17134. doi: 10.1073/pnas.1209148109
Pubmed Abstract | Pubmed Full Text | CrossRef Full Text | Google Scholar
Laxmi, A., Pan, J., Morsy, M., and Chen, R. (2008). Light plays an essential role in intracellular distribution of auxin efflux carrier PIN2 in Arabidopsis thaliana. PLoS ONE 3:e1510. doi: 10.1371/journal.pone.0001510
Pubmed Abstract | Pubmed Full Text | CrossRef Full Text | Google Scholar
Li, G., and Xue, H. W. (2007). Arabidopsis PLDzeta2 regulates vesicle trafficking and is required for auxin response. Plant Cell 19, 281–295. doi: 10.1105/tpc.106.041426
Pubmed Abstract | Pubmed Full Text | CrossRef Full Text | Google Scholar
Li, X., and Zhang, W. (2008). Salt-avoidance tropism in Arabidopsis thaliana. Plant Signal. Behav. 3, 351–353. doi: 10.4161/psb.3.5.5371
Pubmed Abstract | Pubmed Full Text | CrossRef Full Text | Google Scholar
Liao, H., Rubio, G., Yan, X., Cao, A., Brown, K. M., and Lynch, J. P. (2001). Effect of phosphorus availability on basal root shallowness in common bean. Plant Soil 232, 69–79. doi: 10.1023/A:1010381919003
Pubmed Abstract | Pubmed Full Text | CrossRef Full Text | Google Scholar
Liu, J., Rowe, J., and Lindsey, K. (2014). Hormonal crosstalk for root development: a combined experimental and modeling perspective. Front. Plant Sci. 5:116. doi: 10.3389/fpls.2014.00116
Pubmed Abstract | Pubmed Full Text | CrossRef Full Text | Google Scholar
López-Ráez, J. A., Kohlen, W., Charnikhova, T., Mulder, P., Undas, A. K., Sergeant, M. J.,et al. (2010). Does abscisic acid affect strigolactone biosynthesis? New Phytol. 187, 343–354. doi: 10.1111/j.1469-8137.2010.03291.x
Pubmed Abstract | Pubmed Full Text | CrossRef Full Text | Google Scholar
Maathuis, F. J. (2014). Sodium in plants: perception, signalling, and regulation of sodium fluxes. J. Exp. Bot. 65, 849–858. doi: 10.1093/jxb/ert326
Pubmed Abstract | Pubmed Full Text | CrossRef Full Text | Google Scholar
Maier, A., Schrader, A., Kokkelink, L., Falke, C., Welter, B., Iniesto, E.,et al. (2013). Light and the E3 ubiquitin ligase COP1/SPA control the protein stability of the MYB transcription factors PAP1 and PAP2 involved in anthocyanin accumulation in Arabidopsis. Plant J. 74, 638–651. doi: 10.1111/tpj.12153
Pubmed Abstract | Pubmed Full Text | CrossRef Full Text | Google Scholar
Mancuso, S., Marras, A. M., Mugnai, S., Schlicht, M., Zársky, V., Li, G.,et al. (2007). Phospholipase dzeta2 drives vesicular secretion of auxin for its polar cell-cell transport in the transition zone of the root apex. Plant Signal. Behav. 2, 240–244. doi: 10.4161/psb.2.4.4566
Pubmed Abstract | Pubmed Full Text | CrossRef Full Text | Google Scholar
Mayzlish-Gati, E., De-Cuyper, C., Goormachtig, S., Beeckman, T., Vuylsteke, M., Brewer, P. B.,et al. (2012). Strigolactones are involved in root response to low phosphate conditions in Arabidopsis. Plant Physiol. 160, 1329–1341. doi: 10.1104/pp.112.202358
Pubmed Abstract | Pubmed Full Text | CrossRef Full Text | Google Scholar
Meng, L. S. (2014). Transcription coactivator Arabidopsis ANGUSTIFOLIA3 modulates anthocyanin accumulation and light-induced root elongation through transrepression of constitutive photomorphogenic 1. Plant Cell Environ. doi: 10.1111/pce.12456 [Epub ahead of print].
Pubmed Abstract | Pubmed Full Text | CrossRef Full Text | Google Scholar
Nelson, D. C., Flematti, G. R., Riseborough, J. A., Ghisalberti, E. L., Dixon, K. W., and Smith, S. M. (2010). Karrikins enhance light responses during germination and seedling development in Arabidopsis thaliana. Proc. Natl. Acad. Sci. U.S.A. 107, 7095–7100. doi: 10.1073/pnas.0911635107
Pubmed Abstract | Pubmed Full Text | CrossRef Full Text | Google Scholar
Nick, P., Han, M. J., and An, G. (2009). Auxin stimulates its own transport by shaping actin filaments. Plant Physiol. 151, 155–167. doi: 10.1104/pp.109.140111
Pubmed Abstract | Pubmed Full Text | CrossRef Full Text | Google Scholar
Oyama, T., Shimura, Y., and Okada, K. (1997). The Arabidopsis HY5 gene encodes a bZIP protein that regulates stimulus-induced development, of root and hypocotyl. Genes Dev. 11, 2983–2995. doi: 10.1101/gad.11.22.2983
Pubmed Abstract | Pubmed Full Text | CrossRef Full Text | Google Scholar
Pandya-Kumar, N., Shema, R., Kumar, M., Mayzlish-Gati, E., Levy, D., Zemach, H.,et al. (2014). Strigolactone analog GR24 triggers changes in PIN2 polarity, vesicle trafficking and actin filament architecture. New Phytol. 202, 1184–1196. doi: 10.1111/nph.12744
Pubmed Abstract | Pubmed Full Text | CrossRef Full Text | Google Scholar
Pardo, J. M. (2010). Biotechnology of water and salinity stress tolerance. Curr. Opin. Biotechnol. 21, 185–196. doi: 10.1016/j.copbio.2010.02.005
Pubmed Abstract | Pubmed Full Text | CrossRef Full Text | Google Scholar
Pierik, R., and Testerink, C. (2014). The art of being fexible: how to escape from shade, salt, and drought. Plant Physiol. 166, 5–22. doi: 10.1104/pp.114.239160
Pubmed Abstract | Pubmed Full Text | CrossRef Full Text | Google Scholar
Raghavendra, A. S., Gonugunta, V. K., Christmann, A., and Grill, E. (2010). ABA perception and signalling. Trends Plant Sci. 15, 395–401. doi: 10.1016/j.tplants.2010.04.006
Pubmed Abstract | Pubmed Full Text | CrossRef Full Text | Google Scholar
Rizzini, L., Favory, J. J., Cloix, C., Faggionato, D., O’Hara, A., Kaiserli, E.,et al. (2011). Perception of UV-B by the Arabidopsis UVR8 protein. Science 332, 103–106. doi: 10.1126/science.1200660
Pubmed Abstract | Pubmed Full Text | CrossRef Full Text | Google Scholar
Rosquete, M. R., and Kleine-Vehn, V. (2013). Halotropism: turning down the salty date. Curr. Biol. 23, R927–R929. doi: 10.1016/j.cub.2013.08.020
Pubmed Abstract | Pubmed Full Text | CrossRef Full Text | Google Scholar
Santelia, D., Henrichs, S., Vincenzetti, V., Sauer, M., Bigler, L., Klein, M.,et al. (2008). Flavonoids redirect PIN-mediated polar auxin fluxes during root gravitropic responses. J. Biol. Chem. 283, 31218–31226. doi: 10.1074/jbc.M710122200
Pubmed Abstract | Pubmed Full Text | CrossRef Full Text | Google Scholar
Sassi, M., Lu, Y., Zhang, Y., Wang, J., Dhonukshe, P., Blilou, I.,et al. (2012). COP1 mediates the coordination of root and shoot growth by light through modulation of PIN1 and PIN2-dependent auxin transport in Arabidopsis. Development 139, 3402–3412. doi: 10.1242/dev.078212
Pubmed Abstract | Pubmed Full Text | CrossRef Full Text | Google Scholar
Sharp, R. E., Poroyko, V., Hejlek, L. G., Spollen, W. G., Springer, G. K., Bohnert, H. J.,et al. (2004). Root growth maintenance during water deficits: physiology to functional genomics. J. Exp. Bot. 55, 2343–2351. doi: 10.1093/jxb/erh276
Pubmed Abstract | Pubmed Full Text | CrossRef Full Text | Google Scholar
Shen, H., Luong, P., and Huq, E. (2007). The F-box protein MAX2 functions as a positive regulator of photomorphogenesis in Arabidopsis. Plant Physiol. 145, 1471–1483. doi: 10.1104/pp.107.107227
Pubmed Abstract | Pubmed Full Text | CrossRef Full Text | Google Scholar
Shen, H., Zhu, L., Bu, Q. Y., and Huq, E. (2012). MAX2 affects multiple hormones to promote photomorphogenesis. Mol. Plant 5, 750–762. doi: 10.1093/mp/sss029
Pubmed Abstract | Pubmed Full Text | CrossRef Full Text | Google Scholar
Sibout, R., Sukumar, P., Hettiarachchi, C., Holm, M., Muday, G. K., and Hardtke, C. S. (2006). Opposite root growth phenotypes of hy5 versus hy5 hyh mutants correlate with increased constitutive auxin signaling. PLoS Genet. 2:e202. doi: 10.1371/journal.pgen.0020202
Pubmed Abstract | Pubmed Full Text | CrossRef Full Text | Google Scholar
Stangherlin, A., and Reddy, A. B. (2013). Regulation of circadian clocks by redox homeostasis. J. Biol. Chem. 288, 26505–26511. doi: 10.1074/jbc.R113.457564
Pubmed Abstract | Pubmed Full Text | CrossRef Full Text | Google Scholar
Stirnberg, P., Furner, I. J., and Leyser, H. M. O. (2007). MAX2 participates in an SCF complex which acts locally at the node to suppress shoot branching. Plant J. 50, 80–94. doi: 10.1111/j.1365-313X.2007.03032.x
Pubmed Abstract | Pubmed Full Text | CrossRef Full Text | Google Scholar
Sun, F., Zhang, W., Hu, H., Li, B., Wang, Y., Zhao, Y.,et al. (2008). Salt modulates gravity signaling pathway to regulate growth direction of primary roots in Arabidopsis. Plant Physiol. 146, 178–188. doi: 10.1104/pp.107.109413
Pubmed Abstract | Pubmed Full Text | CrossRef Full Text | Google Scholar
Sun, X. D., and Ni, M. (2011). HYPOSENSITIVE TO LIGHT, an alpha/beta fold protein, acts downstream of ELONGATED HYPOCOTYL 5 to regulate seedling de-etiolation. Mol. Plant 4, 116–126. doi: 10.1093/mp/ssq055
Pubmed Abstract | Pubmed Full Text | CrossRef Full Text | Google Scholar
Taniguchi, Y. Y., Taniguchi, M., Tsuge, T., Oka, A., and Aoyama, T. (2010). Involvement of Arabidopsis thaliana phospholipase Dzeta2 in root hydrotropism through the suppression of root gravitropism. Planta 231, 491–497. doi: 10.1007/s00425-009-1052-x
Pubmed Abstract | Pubmed Full Text | CrossRef Full Text | Google Scholar
Toh, S., McCourt, P., and Tsuchiya, Y. (2012). HY5 is involved in strigolactone-dependent seed germination in Arabidopsis. Plant Signal. Behav. 7, 556–558. doi: 10.4161/psb.19839
Pubmed Abstract | Pubmed Full Text | CrossRef Full Text | Google Scholar
Tong, H., Leasure, C. D., Hou, X., Yuen, G., Briggs, W., and He, Z.-H. (2008). Role of root UV-B sensing in Arabidopsis early seedling development. Proc. Natl. Acad. Sci. U.S.A. 105, 21039–21044. doi: 10.1073/pnas.0809942106
Pubmed Abstract | Pubmed Full Text | CrossRef Full Text | Google Scholar
Trewavas, A. (2005). Plant intelligence. Naturwissenschaften 92, 401–413. doi: 10.1007/s00114-005-0014-9
Pubmed Abstract | Pubmed Full Text | CrossRef Full Text | Google Scholar
Trewavas, A. (2009). What is plant behaviour? Plant Cell Environ. 32, 606–616. doi: 10.1111/j.1365-3040.2009.01929.x
Pubmed Abstract | Pubmed Full Text | CrossRef Full Text | Google Scholar
Trewavas, A. (2014). Plant Behaviour and Intelligence. Oxford: Oxford University Press. doi: 10.1093/acprof:oso/9780199539543.001.0001
Trewavas, A., and Baluška, F. (2011). The ubiquity of consciousness. The ubiquity of consciousness, cognition and intelligence in life. EMBO Rep. 12, 1221–1225. doi: 10.1038/embor.2011.218
Tsuchiya, Y., Vidaurre, D., Toh, S., Hanada, A., Nambara, E., Kamiya, Y.,et al. (2010). A small-molecule screen identifies new functions for the plant hormone strigolactone. Nat. Chem. Biol. 6, 741–749. doi: 10.1038/nchembio.435
Pubmed Abstract | Pubmed Full Text | CrossRef Full Text | Google Scholar
Umehara, M., Hanada, A., Yoshida, S., Akiyama, K., Arite, T., Takeda-Kamiya, N.,et al. (2008). Inhibition of shoot branching by new terpenoid plant hormones. Nature 455, 195–200. doi: 10.1038/nature07272
Pubmed Abstract | Pubmed Full Text | CrossRef Full Text | Google Scholar
Waller, F., and Nick, P. (1997). Response of actin microfilaments during phytochrome-controlled growth of maize seedlings. Protoplasma 200, 154–162. doi: 10.1007/BF01283291
Wan, Y., Jasik, J., Wang, L., Hao, H., Volkmann, D., Menzel, D.,et al. (2012). The signal transducer NPH3 integrates the phototropin1 photosensor with PIN2-based polar auxin transport in Arabidopsis root phototropism. Plant Cell 24, 551–565. doi: 10.1105/tpc.111.094284
Pubmed Abstract | Pubmed Full Text | CrossRef Full Text | Google Scholar
Warnasooriya, S. N., and Montgomery, B. L. (2011). Spatial-specific regulation of root development by phytochromes in Arabidopsis thaliana. Plant Signal. Behav. 6, 2047–2050. doi: 10.4161/psb.6.12.18267
Pubmed Abstract | Pubmed Full Text | CrossRef Full Text | Google Scholar
Waters, M. T., Nelson, D. C., Scaffidi, A., Flematti, G. R., Sun, Y. K., Dixon, K. W.,et al. (2012). Specialisation within the DWARF14 protein family confers distinct responses to karrikins and strigolactones in Arabidopsis. Development 139, 1285–1295. doi: 10.1242/dev.074567
Pubmed Abstract | Pubmed Full Text | CrossRef Full Text | Google Scholar
Waters, M. T., Wang, P., Korkaric, M., Capper, R. G., Saunders, N. J., and Langdale, J. A. (2009). GLK transcription factors coordinate expression of the photosynthetic apparatus in Arabidopsis. Plant Cell 21, 1109–1128. doi: 10.1105/tpc.108.065250
Pubmed Abstract | Pubmed Full Text | CrossRef Full Text | Google Scholar
Werner, T., Nehnevajova, E., Köllmer, I., Novák, O., Strnad, M., Krämer, U.,et al. (2010). Root-specific reduction of cytokinin causes enhanced root growth, drought tolerance, and leaf mineral enrichment in Arabidopsis and tobacco. Plant Cell 22, 3905–3920. doi: 10.1105/tpc.109.072694
Pubmed Abstract | Pubmed Full Text | CrossRef Full Text | Google Scholar
Woolley, J. T., and Stoller, E. W. (1978). Light penetration and light-induced seed germination in soil. Plant Physiol. 61, 597–600. doi: 10.1104/pp.61.4.597
Pubmed Abstract | Pubmed Full Text | CrossRef Full Text | Google Scholar
Xu, W., Ding, G., Yokawa, K., Baluška, F., Li, Q. F., Liu, Y.,et al. (2013). An improved agar-plate method for studying root growth and response of Arabidopsis thaliana. Sci. Rep. 3:1273. doi: 10.1038/srep01273
Pubmed Abstract | Pubmed Full Text | CrossRef Full Text | Google Scholar
Yazdanbakhsh, N., Sulpice, R., Graf, A., Stitt, M., and Fisahn, J. (2011). Circadian control of root elongation and C partitioning in Arabidopsis thaliana. Plant Cell Environ. 34, 877–894. doi: 10.1111/j.1365-3040.2011.02286.x
Pubmed Abstract | Pubmed Full Text | CrossRef Full Text | Google Scholar
Yokawa, K., and Baluška, F. (2014). Pectins, ROS homeostasis and UV-B responses in plant roots. Phytochemistry doi: 10.1016/j.phytochem.2014.08.016 [Epub ahead of print].
Pubmed Abstract | Pubmed Full Text | CrossRef Full Text | Google Scholar
Yokawa, K., Kagenishi, T., and Baluška, F. (2013). Root photomorphogenesis in laboratory-maintained Arabidopsis seedlings. Trends Plant Sci. 18, 117–119. doi: 10.1016/j.tplants.2013.01.002
Pubmed Abstract | Pubmed Full Text | CrossRef Full Text | Google Scholar
Yokawa, K., Kagenishi, T., Kawano, T., Mancuso, S., and Baluška, F. (2011). Illumination of Arabidopsis roots induces immediate burst of ROS production. Plant Signal. Behav. 6, 1460–1464. doi: 10.4161/psb.6.10.18165
Pubmed Abstract | Pubmed Full Text | CrossRef Full Text | Google Scholar
Yokawa, K., Koshiba, T., and Baluška, F. (2014). Light-dependent control of redox balance and auxin biosynthesis in plants. Plant Signal. Behav. 9:e29522. doi: 10.4161/psb.29522
Pubmed Abstract | Pubmed Full Text | CrossRef Full Text | Google Scholar
Yoneyama, K., Yoneyama, K., Takeuchi, Y., and Sekimoto, H. (2007). Phosphorus deficiency in red clover promotes exudation of orobanchol, the signal for mycorrhizal symbionts and germination stimulant for root parasites. Planta 225, 1031–1038. doi: 10.1007/s00425-006-0410-1
Pubmed Abstract | Pubmed Full Text | CrossRef Full Text | Google Scholar
Zhao, Y. (2010). Auxin biosynthesis and its role in plant development. Annu. Rev. Plant Biol. 61, 49–64. doi: 10.1146/annurev-arplant-042809-112308
Pubmed Abstract | Pubmed Full Text | CrossRef Full Text | Google Scholar
Keywords: root, light response, plant hormones, reactive oxygen species, root tropism
Citation: Yokawa K, Fasano R, Kagenishi T and Baluška F (2014) Light as stress factor to plant roots – case of root halotropism. Front. Plant Sci. 5:718. doi: 10.3389/fpls.2014.00718
Received: 27 October 2014; Accepted: 28 November 2014;
Published online: 12 December 2014.
Edited by:
Vadim Volkov, London Metropolitan University, UKReviewed by:
Francois Bouteau, Université Paris Diderot, FranceZheng-Hui He, San Francisco State University, USA
Copyright © 2014 Yokawa, Fasano, Kagenishi and Baluška. This is an open-access article distributed under the terms of the Creative Commons Attribution License (CC BY). The use, distribution or reproduction in other forums is permitted, provided the original author(s) or licensor are credited and that the original publication in this journal is cited, in accordance with accepted academic practice. No use, distribution or reproduction is permitted which does not comply with these terms.
*Correspondence: František Baluška, Department of Plant Cell Biology, Institute of Cellular and Molecular Botany, University of Bonn, Kirschallee 1, 53115 Bonn, Germany e-mail:YmFsdXNrYUB1bmktYm9ubi5kZQ==