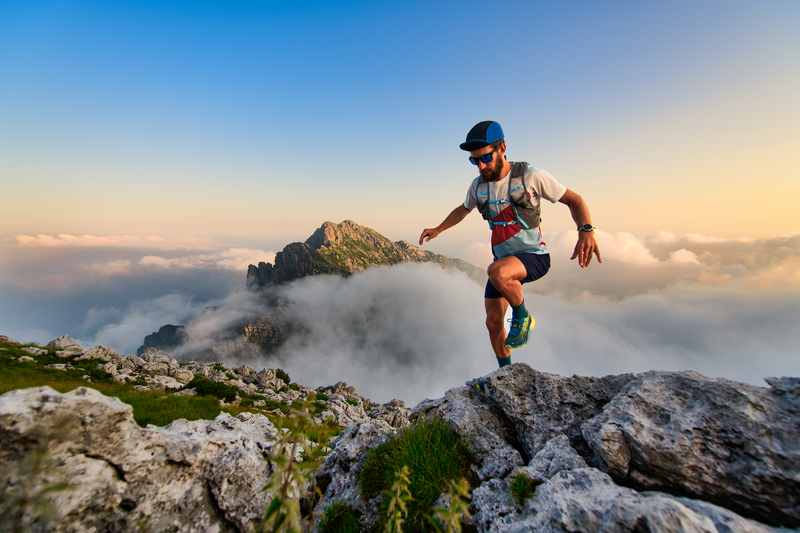
95% of researchers rate our articles as excellent or good
Learn more about the work of our research integrity team to safeguard the quality of each article we publish.
Find out more
ORIGINAL RESEARCH article
Front. Plant Sci. , 01 November 2012
Sec. Plant Physiology
Volume 3 - 2012 | https://doi.org/10.3389/fpls.2012.00234
This article is part of the Research Topic Retrograde Signaling in Plants View all 26 articles
Glycolate oxidase (GO) catalyses the oxidation of glycolate to glyoxylate, thereby consuming O2 and producing H2O2. In this work, Arabidopsis thaliana plants expressing GO in the chloroplasts (GO plants) were used to assess the expressional behavior of reactive oxygen species (ROS)-responsive genes and transcription factors (TFs) after metabolic induction of H2O2 formation in chloroplasts. In this organelle, GO uses the glycolate derived from the oxygenase activity of RubisCO. Here, to identify genes responding to an abrupt production of H2O2 in chloroplasts we used quantitative real-time PCR (qRT-PCR) to test the expression of 187 ROS-responsive genes and 1880 TFs after transferring GO and wild-type (WT) plants grown at high CO2 levels to ambient CO2 concentration. Our data revealed coordinated expression changes of genes of specific functional networks 0.5 h after metabolic induction of H2O2 production in GO plants, including the induction of indole glucosinolate and camalexin biosynthesis genes. Comparative analysis using available microarray data suggests that signals for the induction of these genes through H2O2 may originate in the chloroplast. The TF profiling indicated an up-regulation in GO plants of a group of genes involved in the regulation of proanthocyanidin and anthocyanin biosynthesis. Moreover, the upregulation of expression of TF and TF-interacting proteins affecting development (e.g., cell division, stem branching, flowering time, flower development) would impact growth and reproductive capacity, resulting in altered development under conditions that promote the formation of H2O2.
Photosynthetic organisms are confronted with reactive oxygen species (ROS), such as singlet oxygen (1O2), the superoxide anion radical (O2−), the hydroxyl radical (OH·), and hydrogen peroxide (H2O2), which may cause oxidative stress and damage to important biological molecules (Apel and Hirt, 2004; Møller et al., 2007). Plants in their natural environments are often exposed to sudden increases in light intensity, which results in the absorption of excitation energy in excess of that required for metabolism. In chloroplasts, when absorbed energy is in excess at photosystem II (PSII), O2− is produced during the Mehler reaction by Fd-NADPH oxidase at PSI and is dismutated by superoxide dismutase (SOD) to H2O2 (Ort and Baker, 2002; Asada, 2006). The photorespiratory pathway consumes photosynthetic reducing energy and produces H2O2 in the peroxisomes through the action of glycolate oxidase (GO) (Maurino and Peterhansel, 2010). H2O2 is also produced during a variety of different reactions under stress conditions, often through the detoxification of 1O2 and O2−. The generated H2O2 is scavenged by different antioxidant/enzyme reactions: the ascorbate and glutathione cycles, ascorbate peroxidase (APX), catalase, and peroxiredoxin (PRX) (Tripathi et al., 2009).
ROS generated in the chloroplast have been implicated as triggers of signaling pathways that influence expression of nuclear-encoded genes, which may initiate responses such as cell death or acclimation depending on the degree of the stress (Karpinski et al., 1999; Fryer et al., 2003; Op den Camp et al., 2003; Danon et al., 2005). H2O2 can take part in signaling acting as messenger either directly (e.g., by reversibly modifying critical thiol groups in target proteins; Neill et al., 2002) or by using an oxidized product as a secondary messenger (Møller et al., 2007). The H2O2-scavenging enzymes APX and dehydroascorbate reductase (DHAR) may act as highly efficient initiators of oxidative signaling by generating transient bursts of reduced glutathione. This in consequence triggers glutaredoxin-mediated protein oxidation (Neill et al., 2002). Crosstalk between redox pools of different cellular compartments, possibly transmitted by a redox shift in cellular components, has also been suggested to be important for control of the expression of nuclear genes (Baier and Dietz, 2005; Leister, 2005). A generalized model of H2O2 signal transduction pathways suggests that H2O2 may also directly oxidize transcription factors (TFs) in either the cytosol or the nucleus. Alternatively, H2O2-mediated activation of a signaling protein such as a protein kinase may activate TFs (Mittler et al., 2004; Miao et al., 2007). TFs would interact with cognate H2O2-response elements in target gene promoters thereby modulating gene expression (Foyer and Noctor, 2005). Recently, Møller and Sweetlove (2010) put forward the hypothesis that H2O2 itself is unlikely to be the signaling molecule that selectively regulates nuclear-encoded chloroplastic genes but rather that oxidized peptides deriving from proteolysis of oxidized proteins would act as second messengers during retrograde ROS signaling. On the other hand, using spin trapping EPR spectroscopy in addition to chemical assays (employing Amplex Red reagent), Mubarakshina et al. (2010) showed that 5% of the H2O2 produced inside chloroplasts at high light intensities can actually be detected outside the organelles. This process may involve the pass of H2O2 through aquaporins (Bienert et al., 2007) and might be sufficient to trigger signaling processes outside the chloroplasts.
Desikan et al. (2001) showed that approximately 1% of the transcriptome was altered in H2O2-treated Arabidopsis thaliana (A. thaliana) cell cultures. Although H2O2-responsive promoters have been identified (Desikan et al., 2001), specific H2O2-regulatory DNA sequences and their cognate TFs have not been isolated and characterized. In more recent studies genes involved in H2O2 signal transduction have been identified or proposed, including mitogen-activated protein kinases (MAPKs), various TFs of e.g., the NAC, ZAT, and WRKY families, miRNAs and others (Van Breusegem et al., 2008; Li et al., 2011; Petrov and Van Breusegem, 2012). Moreover, using genome-wide analysis of catalase deficient A. thaliana, H2O2 was inferred to regulate the expression of genes encoding specific small heat shock proteins, several TFs and candidate regulatory proteins (Vandenabeele et al., 2004; Vanderauwera et al., 2005).
To date, it is not known to which extent the chemical specificity of the ROS and the cellular compartment of their release may contribute to the multiplicity of responses that occur in plants. A major challenge is to dissect the genetic networks that control ROS signaling and to assess specific and common responses toward different types of ROS signals. To this end, the molecular, biochemical and physiological responses of A. thaliana to elevated in planta levels of H2O2 were and are being investigated in various types of model systems including mutants altered in the ROS scavenging machinery (Maurino and Flügge, 2008). However, the analysis of dynamic physiological processes using (knock-out) mutants may not always be straightforward, especially when compensatory cellular mechanisms are induced. With respect to ROS-related mutants, changing the balance of scavenging enzymes may induce compensatory mechanisms such that signaling and oxidative damage effects may not be easily separated. Moreover, invasive experimental setups like the application of oxidative stress-causing agents may induce a non-specific oxidative stress that acts throughout the cell and triggers additional responses that may complicate the analysis of ROS signal transduction pathways (Maurino and Flügge, 2008). We have recently developed a tool to functionally dissect the action of plastid-generated H2O2, using plants overexpressing GO in plastids (GO plants; Fahnenstich et al., 2008). During photosynthesis, the oxygenase activity of ribulose 1,5-bisphosphate carboxylase/oxygenase (RubisCO) produces glycolate 2-phosphate within the chloroplasts, which is then dephosphorylated to glycolate by phosphoglycolate phosphatase (Maurino and Peterhansel, 2010). In GO plants, glycolate is oxidized to glyoxylate by the plastidic GO, with the parallel production of H2O2. When growing under moderate photon fluxes and ambient CO2 concentration (photorespiratory conditions) the GO plants remain smaller than the wild type, presenting a reduced rosette diameter and yellowish leaves due to H2O2 accumulation (Fahnenstich et al., 2008). In contrast, in non-photorespiratory conditions (e.g., at high CO2 concentration) the oxygenase activity of RubisCO is abolished and thus, the metabolic flux through GO is suppressed, allowing GO plants to grow like wild type (Fahnenstich et al., 2008). Transferring GO plants from high to ambient CO2 concentration specifically induces H2O2 formation in the chloroplasts (Fahnenstich et al., 2008). These properties permit the modulation of plastidic produced H2O2 levels by changing light intensity and/or CO2 levels (Maurino and Flügge, 2008). Moreover, H2O2 is specifically generated without a concomitant accumulation of superoxide or singlet oxygen, which are common precursors of H2O2 during ROS generation in chloroplasts. A similar experimental set-up was employed in previous studies using catalase null mutants in which the production of peroxisomal H2O2 is induced by changing the conditions of plant growth from non-photorespiratory to photorespiratory conditions (e.g., high light intensity) (Dat et al., 2000; Vandenabeele et al., 2004; Vanderauwera et al., 2005). The metabolic production of H2O2 may avoid the pleiotropic effects discussed above but it cannot be ruled out that ROS-unrelated pleiotropic reactions may occur in both approaches due to abrupt changes in CO2 level or light intensity.
In this work we attempted to identify genes strongly responding to an abrupt production of H2O2 in chloroplasts of A. thaliana. To this end we tested the expressional changes of 187 nuclear-encoded ROS-responsive genes and 1880 TFs, using quantitative real-time (qRT)-PCR (Czechowski et al., 2004; Balazadeh et al., 2008; Wu et al., 2012) upon transfer of high CO2-grown GO and wild-type (WT) plants to ambient CO2 concentration. Our data revealed a rapid and coordinated expression response of ROS-affected genes of specific functional networks in GO including an early induction of indole glucosinolate and camalexin biosynthesis genes and an up-regulation of a group of genes involved in the regulation of proanthocyanidin and anthocyanin biosynthesis. Moreover, the upregulation of expression of TF and TF-interacting proteins affecting development (e.g., cell division, stem branching, flowering time, flower development) would impact growth and reproductive capacity, resulting in altered development under conditions that promote the formation of H2O2.
Arabidopsis thaliana (L.) Heynh. ecotype Columbia-0 (Col-0, wild-type) constitutively expressing glycolate oxidase (GO, At3g14420) in the plastids (GO plants) under the cauliflower mosaic virus 35S promoter were generated in our previous work (Fahnenstich et al., 2008). In these plants to direct the expression of GO to the chloroplats the stromal targeting presequence from Arabidopsis thaliana phosphoglucomutase (At5g51820) was used (Fahnenstich et al., 2008). WT and GO transgenic plants were grown in pots containing 3 parts of soil (Gebr. Patzer KG, Sinntal-Jossa, Germany) and one part of vermiculite (Basalt Feuerfest, Linz, Austria) under a 16 h-light/8 h-dark regime at photosynthetically active photon flux densities (PPFD) of 75 μmol quanta m−2 s−1 at 22°C day/18°C night temperatures and a CO2 concentration of 3000 ppm. After 3 weeks of growth plants were transferred to ambient CO2 concentration (380 ppm) and the same PPFD. Whole rosettes were harvested at different time points after transfer, immediately frozen in liquid nitrogen and stored at −80°C until use for RNA isolation and H2O2 measurements.
For the large-scale qRT-PCR analysis, total RNA was extracted from 100 mg leaves (fresh weight) using RNeasy Plant Mini kit (Qiagen, Valencia, USA) according to the manufacturer's protocol. DNAse I digestion was performed on 20–30 μg of total RNA using TURBO DNase Kit (Ambion, Cambridgeshire, UK) according to manufacturer's instructions. RNA integrity was checked on 1% (w/v) agarose gels and concentration measured with a Nanodrop ND-1000 spectrophotometer before and after DNAse treatment. Absence of genomic DNA was confirmed subsequently by quantitative PCR using primers that amplify an intron sequence of the gene At5g65080 (forward 5′-TTTTTTGCCCCCTTCGAATC-3′ and reverse 5′-ATCTTCCGCCACCACATTGTAC-3′). First-strand cDNA was synthesized from 8 μg to 10 μg of total RNA using RevertAid™ First Strand cDNA Synthesis Kit (Fermentas, St. Leon-Rot, Germany) following the manufacturer's protocol. The efficiency of cDNA synthesis was estimated by qRT-PCR using two different primer sets annealing to the 5′ and 3′ ends, respectively, of a control gene (At3g26650, GAPDH, glyceraldehyde-3-phosphate dehydrogenase). Primer sequences were as follows: for GAPDH3′, forward 5′-TTGGTGACAACAGGTCAAGCA-3′ and reverse 5′-AAACTTGTCGCTCAATGCAATC-3′; for GAPDH5′, forward 5′-TCTCGATCTCAATTTCGCAAAA-3′ and reverse 5′-CGAAACCGTTGATTCCGATTC-3′. Transcript levels of each gene were normalized to ACTIN2 (At3g18780) transcript abundance (forward 5′-TCCCTCAGCACATTCCAGCAGAT-3′ and reverse 5′-AACGATTCCTGGACCTGCCTCATC-3′). A total of 187 ROS-responsive genes (Wu et al., 2012) and 1880 TFs (Czechowski et al., 2004; Balazadeh et al., 2008) were analyzed by qRT-PCR as previously described (Caldana et al., 2007; Balazadeh et al., 2008). PCR reactions were run on an ABI PRISM 7900HT sequence detection system (Applied Biosystems, Darmstadt, Germany), and amplification products were visualized using SYBR Green (Applied Biosystems).
Levels of H2O2 were determined using the Amplex® Red Technology (Life Technologies, Darmstadt, Germany) following the manufacturer's instructions. Amplex Red (N-acetyl-3,7-dihydroxyphenoxazine) reacts with H2O2 in the presence of horseradish peroxidase and forms the fluorescent product resorufin. For the determinations, 100 mg leaves (fresh weight) were ground in liquid nitrogen into a fine powder and resuspended with 0.15 mL extraction buffer prepared as indicated by the manufacturer. This suspension was centrifuged at 4°C at 13,000 rpm for 15 min. Five μL of the supernatant, 45 μL distilled water and 50 μL of Amplex® Red solution were added to a microtitre plate. After 30 min incubation in the dark fluorescence was measured by excitation at 560 nm and emission reads at 590 nm. A calibration curve was established with known H2O2 concentrations.
The two genes that were most strongly induced under photorespiratory conditions in GO plants at the 0.5 and 6 h time points (At3g02840 and At1g17180, respectively) were used as baits to identify globally coexpressed genes using the ATTED-II database (http://atted.jp), which allows evaluating genes that are coexpressed under five experimental conditions (tissue, abiotic stress, biotic stress, hormones, and light conditions) (Obayashi et al., 2009).
The production of H2O2 in leaves of plants overexpressing GO in the plastids (Fahnenstich et al., 2008) was analyzed after activation of photorespiration by transferring high CO2-grown plants to ambient-CO2 conditions. As shown in Table 1, higher levels of H2O2 were determined in GO than in WT plants at 0.5 and 4 h after transfer while GO and WT plants maintained under non-photorespiratory conditions (3000 ppm CO2) showed similar H2O2 levels at both time points (Table 1). Note, that as the measurements were performed using whole-leaf extracts the expected differences in chloroplastic H2O2 levels between GO and WT plants under photorespiratory condition may be higher than determined here.
Table 1. Levels of H2O2 measured in whole rosettes (μmol/g FW) after shifting high CO2-grown wild-type and GO plants to ambient CO2 concentration for 0.5 and 4 h.
To study the impact of an abrupt production of H2O2 in chloroplasts on nuclear gene expression, we analyzed transcript level changes of 187 ROS-responsive genes using a previously established qRT-PCR platform (detailed in Wu et al., 2012). The genes included in the platform were chosen from published reports and our own experiments and represent four different groups that were already shown to be rapidly induced by (1) superoxide radical (O2−; 18 genes), (2) singlet oxygen (1O2; 22 genes), (3) H2O2 (53 genes), or (4) different types of ROS (general ROS-responsive genes; 94 in total).
Gene expression was analyzed in whole rosettes of 3-week-old WT and GO plants at 0.5, 4, 6, and 12 h after shifting high-CO2-grown plants (non-photorespiratory condition) to ambient CO2 concentration (photorespiratory condition). Expression profiling was performed in two biological replicates and log-fold change (log2 FC) ratios of expression changes were calculated for GO and WT plants by comparing gene expression levels before and after the CO2 concentration shift. A total of 131 genes were expressed in all examined samples (Table A1 in Appendix). The remaining 56 genes did not yield detectable PCR amplicons, indicating no or marginal expression under our experimental conditions.
Considering a 3-fold expression difference cut-off, 120 genes displayed differential expression in GO and/or WT plants upon transfer from high to ambient CO2 concentration; the vast majority of the affected genes (116 in total) were up-regulated, and only four genes were down-regulated (Figure 1, Table A1 in Appendix). Most noticeably, expression of 58 genes was induced in GO plants already within 0.5 h after the transfer to ambient CO2 condition, whilst only a single gene was induced in the wild type at the same time point (Figure 1). Importantly, however, many genes showed also high expression in the wild type at later time points after the CO2 concentration shift, but the expressional changes were in most cases more pronounced in GO than WT plants (Figure 1, and section “Early Induction of Indole Glucosinolate and Camalexin Biosynthesis Genes in GO Plants”). Thus, our data indicate that similar sets of ROS-responsive genes responded to the CO2 shift in GO and WT plants; however, the dynamics of the transcriptional responses were clearly different in the two types of plants, being faster and more prominent in the GO plants.
Figure 1. Venn diagram of the number of ROS-responsive genes differentially expressed in wild-type and GO plants at different time points (0.5, 4, 6, and 12 h) after the transfer of plants grown at high CO2 concentration (3000 ppm) to ambient CO2 concentration (380 ppm).
To identify transcripts responsive to metabolically produced H2O2 we focused our analysis on the 0.5- and 6-h time points. Genes were considered differentially expressed when the fold change was more than 3-fold (log2 ≥ 1.56).
At 0.5 h after shifting plants to ambient CO2 concentration, 58 of the 131 expressed genes were induced in GO plants by more than 3-fold, whilst in the wild type the expression change was less than 3-fold, suggesting that these genes participate in early signaling steps triggered by the production of H2O2 under photorespiratory conditions (Table 2). After 6 h, seven of these genes showed WT levels of expression (below 3-fold), while 29 were further overexpressed only in GO (Table 2). Although at 6 h after transfer to ambient CO2 the expression fold-change (FC) of the remaining 22 genes was higher than 3 in both, GO and WT plants, the expression change between GO and WT (FCGO/FCWT) was higher than 2 for 16 of these genes (Table 2), indicating that their higher expression in GO plants was triggered by the elevated levels of H2O2.
Table 2. ROS-responsive genes (58 in total) the expression of which was enhanced by more than 3-fold in GO plants 0.5 h after shifting plants grown at high CO2 concentration (3000 ppm) to ambient CO2 concentration (380 ppm).
Later responding genes, which were affected only after 6 h under photorespiratory conditions, were also identified. From the 23 genes that showed an expression change of above 3-fold in GO, 13 were only induced in GO, while 10 genes were induced in both, GO and WT. The FC ratio in GO and WT (FCGO/FCWT) was above 2 for the 10 genes (Table 3), indicating that their expression in GO plants is controlled by the higher levels of H2O2, similar to the early-responsive genes.
Table 3. ROS-responsive genes (23 in total) the expression of which was enhanced more than 3-fold in GO plants 6 h after shifting plants grown at high CO2 concentration to ambient CO2 concentration.
The most highly up-regulated gene in GO plants at 0.5 h after induction of H2O2 production was At3g02840 (encoding a putative U-box-type E3 ubiquitin ligase, known to respond immediately-early to fungal elicitation) (Table 2). We used the ATTED-II database (http://atted.jp; Obayashi et al., 2009) to discover genes coexpressed with At3g02840 and observed that 45 of the 58 genes induced at 0.5 h after induction of H2O2 production cluster together (Table 2), indicating that metabolically produced H2O2 in GO plants induces the coordinate expression of functionally related genes. A similar analysis using the most highly expressed gene at 6 h after induction of H2O2 production (At1g17180, encoding glutathione S-transferase Tau 25) indicated that another group of eight genes are coordinately expressed in GO plants at this later time point (Table 3).
Recently, Inzé et al. (2012) listed the 85 most strongly H2O2-responsive genes in catalase loss-of-function mutants shifted from low- to high-light conditions, where H2O2 is produced in peroxisomes by the action of photorespiratory GOs. Interestingly, 23 of the 81 genes, which changed their expression in the GO plants were also differentially expressed in catalase loss-of-function mutants (Tables 2 and 3), indicating that they respond to enhanced levels of H2O2 independent of the site of its generation; the remaining genes may then represent candidates preferentially responsive to H2O2 produced in chloroplasts. Many of the genes up-regulated in GO plants encode proteins or TFs of currently unknown specific functions. Interestingly, however, several of the early-responsive genes are involved in tryptophan-derived biosynthesis of the phytoanticipins camalexin and indole glucosinolates, i.e., secondary metabolites that have antifungal and insect-deterring functions (Kliebenstein et al., 2001; Bednarek et al., 2009). These genes encode (1) the transcription factor WRKY33 (At2g38470), which is involved in controlling camalexin biosynthesis (Birkenbihl et al., 2012); (2) the Myb-type transcription factor HIG1/MYB51 (At1g18570) involved in the positive regulation of indole glucosinolate biosynthesis by activating several target genes (Gigolashvili et al., 2007); (3) the O-methyltransferases IGMT1 (At1g21100) and IGMT2 (At1g21120), which catalyze the transfer of a methyl group to the hydroxy indole glucosinolate hydroxyindol-3-ylmethylglucosinolate (4 and 1OH-I3M, respectively) to form methoxyindol-3-ylmethylglucosinolate (4 and 1MO-I3M, respectively) (Pfalz et al., 2011); and (4) cytochrome P450 monooxygenase CYP81F2 (At5g57220), that is essential for the pathogen-induced accumulation of 4-methoxyindol-3-ylmethylglucosinolate (4MI3G) (Bednarek et al., 2009). Our data thus show the early induction of indole glucosinolate and camalexin biosynthesis genes in GO plants after metabolic formation of H2O2 through the activation of genes encoding enzymes involved in intermediate metabolite conversions and of TFs that act on several target genes of these biosynthetic pathways.
To understand the potential effects of overexpression of GO in chloroplasts on the nuclear transcriptional program, we next broadened our analysis by testing the expression of 1880 TFs using a highly sensitive quantitative real-time PCR (qRT-PCR) platform (Czechowski et al., 2004; Balazadeh et al., 2008). Considering the data obtained from the profiling of the ROS-responsive genes, we analyzed the expression at 0.5 h after induction of H2O2 production to capture the early-responsive TFs. Expression profiling was performed in two biological replicates and log-fold change (log2 FC) ratios of expression changes were calculated for GO and WT plants by comparing gene expression levels before and after the transfer of plants grown at high CO2 to ambient CO2.
TFs most strongly responding to H2O2 were identified by comparing their expression FC in GO and WT plants. A TF was considered differentially expressed when the FC in GO was more than 3-fold (log2 ≥ 1.56) and less than 2-fold in the wild type (log2 ≥ 1.0) (Table 4). Analysis of transcript profiles revealed that the expression of 1449 genes, representing 77% of all TF genes tested, could be detected (Table A2 in Appendix). The remaining 23% (431 of the 1880 TFs) did not yield detectable PCR amplicons, indicating no or very weak expression in the tested material.
Table 4. Transcription factors the expression of which was enhanced by more than 3-fold in GO plants, but less than 2-fold in wild-type plants 0.5 h after shifting plants grown at high CO2 concentration to ambient CO2 concentration.
At 0.5 h after shifting plants to ambient CO2 concentration, 78 of the 1449 genes were induced by more than 3-fold in GO plants, whereas in WT plants the expression changes of the same genes were less than 2-fold (Table 4). Using published data the involvement/participation of the TFs in specific biological processes (Table 4) could be assessed, which allowed the classification of the TFs into five functional groups (FG) enriched with specific gene ontology categories (Figure 2). FG1 contains TFs involved in the regulation of proanthocyanidin and anthocyanin biosynthesis (Table 4 and Figure 2). The TFs TT8 and MYB75 affecting the gene expression of dihydroflavonol 4-reductase (Debeaujon et al., 2003) are included in this FG. FG2 contains TFs affecting developmental processes like lateral root formation (GATA23), flowering (FD1, ANAC089, TEM2 and SNZ), shoot branching (MYB2 and BRC2), senescence (ANAC092/ORE1) and cell division (ANAC068 and HAT4) (Table 4 and Figure 2). The activation of these TFs in GO plants would result in altered growth and flowering (see below and Fahnenstich et al., 2008). FG3 includes TFs and TF-interacting proteins negatively regulating jasmonate (JA) signaling (JAZ7, JAZ8, JAZ9, JAZ10, WRKY50, and WRKY51; Chico et al., 2008; Staswick, 2009; Gao et al., 2011) (Table 4 and Figure 2). JAZ proteins bind directly to the key transcription factor MYC2 and thereby prevent JA-dependent gene transcription (Chini et al., 2007; Pauwels et al., 2010). At the same time JAZ genes are rapidly induced by JA and some are MYC2-regulated. This feedback loop regulation would provide a rapid on and off switch of the pathway involving JA. Transcriptional activation of JAZ genes was found to occur in response to several biotic and abiotic challenges (Yan et al., 2007). JAZ proteins would also exert their effects on post-wound inhibition of vegetative growth in A. thaliana (Yan et al., 2007) and as repressors of necrosis and/or programmed cell death during development in tobacco (Oh et al., 2012). In GO plants, the action of JAZ genes together with those of FG2 would impact growth and reproductive capacity, resulting in altered development under conditions that promote the formation of H2O2. FG4 includes TFs with diverse functions in plant defense and signaling, e.g., activators of tryptophan-derived biosynthesis of camalexin (JUB1/ANAC042) and indole glucosinolates (MYB122), as well as regulators of photomorphogenesis (STH2) (Table 4 and Figure 2). The early activation of camalexin and indole glucosinolate biosynthesis was also observed in the analysis performed with the ROS-responsive gene platform (Table 1). Finally, FG5 includes TFs with currently unknown functions (Table 4 and Figure 2).
Figure 2. Pie chart representation of the five functional groups (FG) of early H2O2-responsive TFs in GO plants. FG5 includes genes for which a distinct biological function has not been reported yet.
The analysis of the transcript profiles at 0.5 h after induction of H2O2 production in GO plants (Table A2 in Appendix) also revealed a group of 13 genes that are down-regulated in GO relative to WT plants (Table 5). The function of five of these genes is currently unknown, but interestingly, the remaining eight genes positively control developmental processes. The down-regulation of expression of these TFs in GO plants together with the up-regulation of expression of TFs negatively affecting development (see FG2, Table 3) would act in concert to arrest growth and especially to delay the transition from the vegetative to the reproductive phase. Consistently, our previous results showed that GO plants growing under photorespiratory conditions are smaller than WT plants, presenting a reduced rosette diameter and a delay in flowering time (Fahnenstich et al., 2008).
Table 5. Transcription factors the expression of which was reduced by more than 3-fold in GO plants 0.5 h after shifting plants grown at high CO2 concentration to ambient CO2 concentration.
The metabolic induction of H2O2 formation in chloroplasts of GO plants under photorespiratory conditions triggered a faster and more prominent transcriptional response of ROS-responsive genes in these plants than in wild type. The changes of the transcriptional activities observed in GO plants early after induction of H2O2 production in chloroplasts suggest the establishment of responses that resemble those occurring at early times after wounding or herbivore attack, where H2O2 is also produced (Orozco-Cardenas and Ryan, 1999). These responses include (1) the retardation of development, which in part would be linked to JA signaling, and (2) the production of the phytoanticipins indole glucosinolates and camalexin. As in the case of herbivore attack, the retardation of development such as reductions in growth and reproduction observed in GO plants could be regarded as a strategy to allow more resource allocation to support defense and tolerance responses (Zavala and Baldwin, 2006). The data also suggest that signals for the early induction of indole glucosinolate and camalexin biosynthesis genes in GO plants through H2O2 may originate in chloroplasts as these genes showed no modified expression in catalase loss-of-function mutants (Inzé et al., 2012).
The authors declare that the research was conducted in the absence of any commercial or financial relationships that could be construed as a potential conflict of interest.
Financial support was provided by the Deutsche Forschungsgemeinschaft (DFG) through grant MA 2379/11-1 to Veronica G. Maurino, and through grant MU 1199/14-1 (FOR 948) to Bernd Mueller-Roeber.
Apel, K., and Hirt, H. (2004). Reactive oxygen species: metabolism, oxidative stress, and signal transduction. Annu. Rev. Plant Biol. 55, 373–399.
Asada, K. (2006). Production and scavenging of reactive oxygen species in chloroplasts and their fuction. Plant Physiol. 141, 391–396.
Baier, M., and Dietz, K.-J. (2005). Chloroplasts as source and target of cellular redox regulation: a discussion on chloroplast redox signals in context of plant physiology. J. Exp. Bot. 56, 1449–1462.
Balazadeh, S., Riaño-Pachón, D. M., and Mueller-Roeber, B. (2008). Transcription factors regulating leaf senescence in Arabidopsis thaliana. Plant Biol. 1, 63–75.
Bednarek, P., Pislewska-Bednarek, M., Svatos, A., Schneider, B., Doubsky, J., Mansurova, M., et al. (2009). A glucosinolate metabolism pathway in living plant cells mediates broad-spectrum antifungal defense. Science 323, 101–106.
Bienert, G. P., Møller, A. L., Kristiansen, K. A., Schulz, A., Møller, I. M., Schjoerring, J. K., et al. (2007). Specific aquaporins facilitate the diffusion of hydrogen peroxide across membranes. J. Biol. Chem. 282, 1183–1192.
Birkenbihl, R. P., Diezel, C., and Somssich, I. E. (2012). Arabidopsis WRKY33 is a key transcriptional regulator of hormonal and metabolic responses toward Botrytis cinerea infection. Plant Physiol. 159, 266–285.
Caldana, C., Scheible, W. R., Mueller-Roeber, B., and Ruzicic, S. (2007). A quantitative RT-PCR platform for high-throughput expression profiling of 2500 rice transcription factors. Plant Meth. 3, 7.
Chico, J. M., Chini, A., Fonseca, S., and Solano, R. (2008). JAZ repressors set the rhythm in jasmonate signaling. Curr. Opin. Plant Biol. 11, 486–494.
Chini, A., Fonseca, S., Fernandez, G., Adie, B., Chico, J. M., Lorenzo, O., et al. (2007). The JAZ family of repressors is the missing link in jasmonate signalling. Nature 448, 666–671.
Czechowski, T., Bari, R. P., Stitt, M., Scheible, W. R., and Udvardi, M. K. (2004). Real-time RT-PCR profiling of over 1400 Arabidopsis transcription factors: unprecedented sensitivity reveals novel root- and shoot-specific genes. Plant J. 38, 366–379.
Dat, J., Vandenabeele, S., Vranová, E., Van Montagu, M., Inzé, D., and Van Breusegem, F. (2000). Dual action of the active oxygen species during plant stress responses. Cell Mol. Life Sci. 57, 779–795.
Danon, A., Miersch, O., Felix, G., and Camp, R. G. Apel, K. (2005). Concurrent activation of cell death-regulating signalling pathways by singlet oxygen in Arabidopsis thaliana. Plant J. 41, 68–80.
Debeaujon, I., Nesi, N., Perez, P., Devic, M., Grandjean, O., Caboche, M., et al. (2003). Proanthocyanidin-accumulating cells in Arabidopsis testa: regulation of differentiation and role in seed development. Plant Cell 15, 2514–2531.
Desikan, R., Mackerness, S., Hancock, and Neill, S. J. (2001). Regulation of the Arabidopsis transcriptome by oxidative stress. Plant Physiol. 127, 159–172.
Fahnenstich, H., Scarpeci, T. E., Valle, E. M., Flügge, U. I., and Maurino, V. G. (2008). Generation of H2O2 in chloroplasts of Arabidopsis thaliana overexpressing glycolate oxidase as an inducible system to study oxidative stress. Plant Physiol. 148, 719–729.
Foyer, C. H., and Noctor, G. (2005). Redox homeostasis and antioxidant signaling: a metabolic interface between stress perception and physiological responses. Plant Cell 17, 1866–1875.
Fryer, M. J., Ball, L., Oxborough, K., Karpinski, S., Mullineaux, P. M., and Baker, N. R. (2003). Control of ascorbate peroxidase 2 expression by hydrogen peroxide and leaf water status during excess light stress reveals a functional organisation of Arabidopsis leaves. Plant J. 33, 691–705.
Gao, Z., Chung, E. H., Eitas, T. K., and Dangl, J. L. (2011). Plant intracellular innate immune receptor Resistance to Pseudomonas syringae pv. maculicola 1 (RPM1) is activated at, and functions on, the plasma membrane. Proc. Natl. Acad. Sci. U.S.A. 108, 8915.
Gigolashvili, T., Yatusevich, R., Berger, B., Müller, C., and Flügge, U. I. (2007). The R2R3-MYB transcription factor HAG1/MYB28 is a regulator of methionine-derived glucosinolate biosynthesis in Arabidopsis thaliana. Plant J. 51, 247–261.
Inzé, A., Vanderauwera, S., Hoeberichts, F. A., Vandorpe, M., Van Gaever, T., and Van Breusegem, F. (2012). A subcellular localization compendium of hydrogen peroxide-induced proteins. Plant Cell Environ. 35, 308–320.
Karpinski, S., Reynoldsm, H., Karpinska, B., Wingsle, G., Creissen, G., and Mullineaux, P. (1999). Systemic signalling and acclimation in response to excess excitation energy in Arabidopsis. Science 23, 654–657.
Kliebenstein, D. J., Kroymann, J., Brown, P., Figuth, A., Pedersen, D., Gershenzon, J., et al. (2001). Genetic control of natural variation in Arabidopsis glucosinolate accumulation. Plant Physiol. 126, 811–825.
Leister, D. (2005). Genomics-based dissection of the cross-talk of chloropalsts with the nucleus and mitochondria in Arabidopsis. Gene 354, 110–116.
Li, T., Li, H., Zhang, Y. X., and Liu, J. Y. (2011). Identification and analysis of seven H2O2-responsive miRNAs and 32 new miRNAs in the seedlings of rice (Oryza sativa L. ssp. indica). Nucleic Acids Res. 39, 2821–2833.
Maurino, V. G., and Flügge, U. I. (2008). Experimental systems to assess the effects of reactive oxygen species in plant tissues. Plant Signal. Behav. 3, 919–924.
Maurino, V. G., and Peterhansel, C. (2010). Photorespiration: current status and approaches for metabolic engineering. Curr. Opin. Plant Biol. 13, 248–255.
Miao, Y., Laun, T. M., Smykowski, A., and Zentgraf, U. (2007). Arabidopsis MEKK1 can take a short cut: it can directly interact with senescence-related WRKY53 transcription factor on the protein level and can bind to its promoter. Plant Mol. Biol. 65, 63–76.
Mittler, R., Vandeerauwera, S., Gollery, M., and Van Breusegem, F. (2004). Reactive oxygen gene network of plants. Trends Plant Sci. 9, 490–498.
Møller, I. M., Jensen, P. E., and Hansson, A. (2007). Oxidative modifications to cellular components in plants. Ann. Rev. Plant Biol. 58, 459–481.
Møller, I. M., and Sweetlove, L. J. (2010). ROS signalling-specificity is required. Trends Plant Sci. 15, 370–374.
Mubarakshina, M. M., Ivanov, B. N., Naydov, I. A., Hillier, W., Badger, M. R., and Krieger-Liszkay, A. (2010). Production and diffusion of chloroplastic H2O2 and its implication to signalling. J. Exp. Bot. 61, 3577–3587.
Neill, S., Desikan, R., and Hancock, J. (2002). Hydrogen peroxide signaling. Curr. Opin. Plant Biol. 5, 388–395.
Obayashi, T., Hayashi, S., Saeki, M., Ohta, H., and Kinoshita, K. (2009). ATTED-II provides coexpressed gene networks for Arabidopsis. Nucleic Acids Res. 37, 987–991.
Oh, Y., Baldwin, I. T., and Gális, I. (2012). NaJAZh regulates a subset of defense responses against herbivores and spontaneous leaf necrosis in Nicotiana attenuata plants. Plant Physiol. 159, 769–788.
Op den Camp, R. G. L., Przybyla, D., Ochsenbein, C., Laloi, C., Kim, C., Danon, A., et al. (2003). Rapid induction of distinct stress responses after the release of singlet oxygen in Arabidopsis. Plant Cell 15, 2320–2332.
Orozco-Cardenas, M., and Ryan, C. A. (1999). Hydrogen peroxide is generated systemically in plant leaves by wounding and systemin via the octadecanoid pathway. Proc. Natl. Acad. Sci. U.S.A. 96, 6553–6557.
Ort, D. R., and Baker, N. R. (2002). A photoprotective role for O2 as an alternative electron sink in photosynthesis? Curr. Opin. Plant Biol. 5, 193–198.
Pauwels, L., Barbero, G. F., Geerinck, J., Tilleman, S., Grunewald, W., Perez, A. C., et al. (2010). NINJA connects the co-repressor TOPLESS to jasmonate signalling. Nature 464, 788–791.
Petrov, V. D., and Van Breusegem, F. (2012). Hydrogen peroxide—a central hub for information flow in plant cells. AoB Plants. 2012, pls014. doi: 10.1093/aobpla/pls014
Pfalz, M., Mikkelsen, M. D., Bednarek, P., Olsen, C. E., Halkier, B. A., and Kroymann, J. (2011). Metabolic engineering in Nicotiana benthamiana reveals key enzyme functions in Arabidopsis indole glucosinolate modification. Plant Cell 23, 716–729.
Staswick, P. E. (2009). The tryptophan conjugates of jasmonic and indole-3-acetic acids are endogenous auxin inhibitors. Plant Physiol. 150, 1310–1321.
Tripathi, B. N., Bhatt, I., and Dietz, K. J. (2009). Peroxiredoxins: a less studied component of hydrogen peroxide detoxification in photosynthetic organisms. Protoplasma 235, 3–15.
Van Breusegem, F., Bailey-Serres, J., and Mittler, R. (2008). Unraveling the tapestry of networks involving reactive oxygen species in plants. Plant Physiol. 147, 978–984.
Vandenabeele, S., Vanderauwera, S., Vuylsteke, M., Rombauts, S., Langebartels, C., Seidlitz, H. K., et al. (2004). Catalase deficiency drastically affects gene expression induced by high light in Arabidopsis thaliana. Plant J. 39, 45–58.
Vanderauwera, S., Zimmermann, P., Rombauts, S., Vandenabeele, S., Langebartels, C., Gruissem, W., et al. (2005). Genome-wide analysis of hydrogen peroxide-regulated gene expression in Arabidopsis reveals a high light-induced transcriptional cluster involved in anthocyanin biosynthesis. Plant Physiol. 139, 806–821.
Wu, A., Allu, A. D., Garapati, P., Siddiqui, H., Dortay, H., Zanor, M.-I., et al. (2012). JUNGBRUNNEN1, a reactive oxygen species-responsive NAC transcription factor, regulates longevity in Arabidopsis. Plant Cell 24, 482–506.
Yan, Y., Stolz, S., Chetelat, A., Reymond, P., Pagni, M., Dubugnon, L., et al. (2007). A downstream mediator in the growth repression limb of the jasmonate pathway. Plant Cell 19, 2470–2483.
Zavala, J. A., and Baldwin, I. T. (2006). Jasmonic acid signalling and herbivore resistance traits constrain regrowth after herbivore attack in Nicotiana attenuata. Plant Cell Environ. 29, 1751–1760.
Table A1. Expression profile of 131 ROS-responsive genes in wild-type (WT) and GO plants after transferring seedlings to ambient CO2 concentration.
Keywords: glycolate oxidase, H2O2, ROS-responsive genes, transcription factors
Citation: Balazadeh S, Jaspert N, Arif M, Mueller-Roeber B and Maurino VG (2012) Expression of ROS-responsive genes and transcription factors after metabolic formation of H2O2 in chloroplasts. Front. Plant Sci. 3:234. doi: 10.3389/fpls.2012.00234
Received: 17 August 2012; Accepted: 01 October 2012;
Published online: 01 November 2012.
Edited by:
Dario Leister, Ludwig-Maximilians-University Munich, GermanyReviewed by:
Ian M. Møller, Aarhus University, DenmarkCopyright © 2012 Balazadeh, Jaspert, Arif, Mueller-Roeber and Maurino. This is an open-access article distributed under the terms of the Creative Commons Attribution License, which permits use, distribution and reproduction in other forums, provided the original authors and source are credited and subject to any copyright notices concerning any third-party graphics etc.
*Correspondence: Veronica G. Maurino, Entwicklungs- und Molekularbiologie der Pflanzen, Heinrich-Heine-Universität, Universitätsstraße 1, 40225 Düsseldorf, Germany. e-mail:dmVyb25pY2EubWF1cmlub0B1bmktZHVlc3NlbGRvcmYuZGU=
† These authors equally contributed to this work.
Disclaimer: All claims expressed in this article are solely those of the authors and do not necessarily represent those of their affiliated organizations, or those of the publisher, the editors and the reviewers. Any product that may be evaluated in this article or claim that may be made by its manufacturer is not guaranteed or endorsed by the publisher.
Research integrity at Frontiers
Learn more about the work of our research integrity team to safeguard the quality of each article we publish.