- Department Disease and Stress Biology, John Innes Centre, Norwich, UK
Cell-to-cell communication is fundamental to multicellular life. For this to occur effectively there must be pathways and dynamic networks for communication. These might depend upon electrical or chemical signals or the mass transfer of molecules between adjacent cells. Molecular communication occurs either via an extra-cellular pathway or through physical structures, called plasmodesmata, that connect the cytoplasm of neighboring cells. Plasmodesmata bridge the rigid physical barrier presented by the cell wall to extend the symplasm from single cells to tissue domains that have functional importance for tissue growth, development, and defense. Although recent years have seen advances in our knowledge of the physical nature of PD, the trafficked molecules, and of the wider processes they affect, our knowledge of PD structure and function is still relatively rudimentary. This article will consider the technical/experimental difficulties hindering PD research and suggest priorities in the future research effort that might advance the field at a significantly faster rate.
Cells in multicellular organisms work as social communities, sharing information and resources, and co-ordinating roles to the benefit of the collective. For this to occur effectively there must be pathways and dynamic networks for communication. These might depend upon electrical or chemical signals or the mass transfer of molecules between adjacent cells. In animals and plants, efflux, and influx carriers on the plasma membrane (PM) and the processes of exo- and endo-cytosis provide the means for molecular communication via an extra-cellular pathway. In addition, physical structures connecting adjacent cells have been identified that connect the cytoplasm of neighboring cells, forming the symplasm. In animals, gap junctions allow the symplasmic transfer of small molecules and tunneling nanotubes or cellular bridges provide the means for larger molecules, and even organelles to pass from cell-to-cell (Gerdes and Carvalho, 2008). In plants the existence of similar structures is complicated by the need to overcome the physical barrier between cells represented by the cell wall. This is achieved by structures called “Plasmodesmata” (PD). These are membrane-lined channels that provide symplasmic connectivity between adjacent cells (Figure 1).
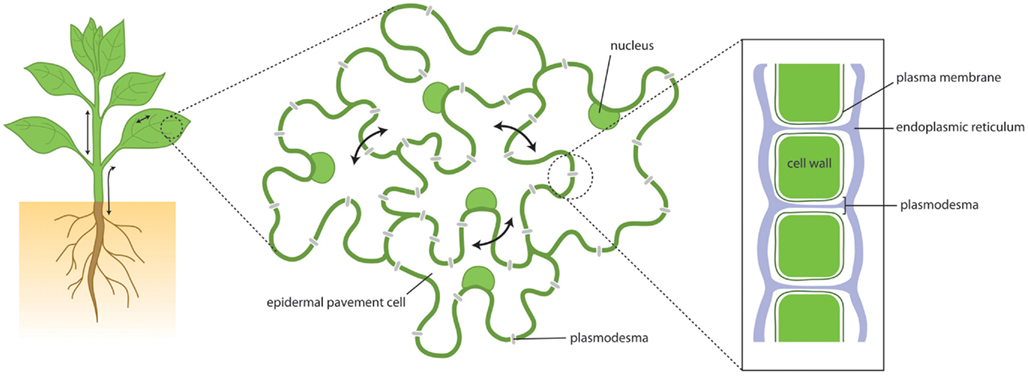
Figure 1. Plant growth and development depends upon cellular communication over short and long distances. This communication depends in part upon movement of molecules via the symplasm – the cytoplasmic continuum that is connected through cell walls by plasmodesmata. Through plasmodesmata cytoplasm, plasma membrane and endoplasmic reticulum are all continuous, providing paths for molecular flux.
It is clear now that development and patterning of the major organs of the plant depend upon the symplasmic movement of transcription factors through PD (Lucas et al., 2009), and that aspects of plant defense, especially against viruses, involve communication between cells ahead of the advancing infection (Benitez-Alfonso et al., 2010a; Harries and Ding, 2011; Lee and Lu, 2011). More recently, small RNA species have been shown to act as mobile signals and, together with non-cell autonomous transcription factors (such as SHORTROOT), generate positional information during the specification of root and shoot organs (Furuta et al., 2011). Despite this fundamental role in plant growth and responses there are major gaps in our insight into these important structures. Nevertheless, recent years have seen advances in our knowledge of the physical nature of PD, of their constituent components, of the trafficking pathways to and through the channel, of the trafficked molecules, and of the wider processes they affect. This article will consider the technical/experimental difficulties for the study of PDs and suggest priorities in the future research effort that might advance the field at a significantly faster rate.
Plasmodesmata form and function are integral to the structure of the cell wall. “Primary PD” are formed during cell division when new PM and cell wall deposition are added to components of the endoplasmic reticulum (ER) that have been trapped in the developing phragmoplast (Burch-Smith and Zambryski, 2012). This results in simple tunnel structures that bridge the cell wall and provide several distinct physical phases for molecular communication between cells: the cytosolic channel, the membrane phases of the ER and PM, and the lumen of the ER. These structures are surrounded by a pectin-enriched wall matrix and have insoluble glucans (β-1,3-glucan or callose) deposited in the wall matrix around the neck region. In mature tissues, PD exhibit more complex morphology consistent with PD branching and/or fusion, although the principle structural components remain the same. The more complex forms, and the occurrence of clusters of PD in pit-fields, relate to ontogenic processes influenced by the rigidity of the surrounding cell wall (Faulkner et al., 2008; Ehlers and van Bel, 2010) or the deposition of new cell wall material (Burch-Smith et al., 2011). Generally, PD formed post-cytokinesis are referred to as “secondary PD.” Simple “primary PD” and complex “secondary PD” may also have different functional properties reflected in the size of molecules able to pass through the channel (Burch-Smith et al., 2011) and the nature of their molecular constituents (Citovsky et al., 1993). These functional differences also define “symplasmic domains” within and between recognizable tissue types. Hence, for example, while the L1, L2, and L3 layers of the shoot apical meristem have distinct communication properties, so too does the central and peripheral zones of the meristem L1 layer (Rinne and Van der Schoot, 1998; Ormenese et al., 2002). Such domains both influence and are regulated by cell fate specification and patterns of tissue development (Stadler et al., 2005; Guseman et al., 2010).
This tight association between PD and the insoluble matrix of the wall and consequent difficulties for PD isolation have presented particular technical challenges for their molecular characterization and have slowed progress in this area. Nevertheless, proteomic characterizations have recently identified components of PD that presumably have roles in their structure and function. Our recent strategy adopted enzymatic methods developed by others (Levy et al., 2007) to remove the cell wall before PD purification, allowing us to report the proteome of isolated Arabidopsis PD (Fernandez-Calvino et al., 2011). This proteome has proven to be a rich source of novel PD proteins the functions of which are mostly unknown. This biochemical approach complements genetic approaches where, surprisingly, the identified genes encode proteins that affect PD function only indirectly. Hence genetic screens based upon passive diffusion of reporter molecules have identified mitochondrial and plastidial enzymes (Kobayashi et al., 2007; Benitez-Alfonso et al., 2009; Stonebloom et al., 2009) that have RNA-binding domains and/or modulate the redox status of the cells with a consequent impact on cell-to-cell trafficking. An alternative screen based upon the trafficking of a transcription factor to activate a developmental program in neighboring cells identified a component of the chaperonin complex needed for the refolding of translocated proteins; this protein was also not located in PDs (Xu et al., 2011).
Our PD proteome is comprised of approximately 1300 proteins (of which ∼30% are predicted to be contaminants); the remaining “soluble” and “membrane” proteins include some with known direct or indirect, or inferred, association with PD. This appears to be a surprisingly large number of proteins for a relatively small structure and raises the question as to how many and which can truly be defined as PD proteins. Answering this question is truly a difficult challenge. Certainly the proteome will contain contaminants, which are an inevitable consequence of improvements in the sensitivity of proteomic technologies. However, predictably when working with “purified” PD as source material the proteome will represent an enriched pool of PD proteins that can be “mined” for the rich nuggets amongst the mining waste. Our approach has been to view PD as membrane-rich environments in which membrane-associated PD proteins would be abundant.
Amongst the “membrane” class of proteins are a number of PD-located receptor-like molecules that point to PD as being a PM domain rich in receptor-like functions; there is evidence that the PM in PD has a distinctive composition with respect to the majority of the PM (Raffaele et al., 2009; Mongrand et al., 2010) Why should these receptors be located at PD and why should their activation by ligand- or partner-binding lead to altered trafficking between cells? These questions remain unanswered at present? That sensing of molecules in the apoplast could lead to symplasmic control of intercellular communication is not difficult to understand in the context of environmental influences on physiology and development; the physical location of receptors at PD might suggest very local control of this process, without the need for a nuclear or transcriptional contribution. An example of this is the potential for PD receptor-like proteins to mediate in host responses to pathogen attack (Lee et al., 2011; our unpublished data), leading to reduced symplasmic transport and/or altered host susceptibility. Other relevant proteins identified in the PD proteome include some with known intercellular mobility (e.g., HSPs; Aoki et al., 2002), some associated with callose accumulation and others affecting cellular redox status (Fernandez-Calvino et al., 2011).
The collective research effort in this area has consumed significant research time and resources but has been rewarded in part by a number of proven PD components that number in the low teens (Faulkner and Maule, 2011; Fernandez-Calvino et al., 2011). However, it has not yet shown the ways in which these proteins work together to deliver a functionally regulated channel critical for plant development and defense. Since we know that PD can control mass flow and specificity, as in the nature of the trafficked molecules and their direction of travel (Kim et al., 2002; Christensen et al., 2009), the future priority must be to dissect the mechanisms of regulation and its impact on particular biological processes. By connecting candidate PD proteins with specific genetic mutant phenotypes we have revealed roles for PD in plant–pathogen interactions, plant–insect interactions and in lateral root development, opening several new angles of research. The challenge in each of these cases is to identify the additional and unknown proteins that work together with the candidates to deliver controlled cell-to-cell communication often at tissue specific interfaces in a restricted time frame (this spatio-temporal limitation lies at the heart of the concept of symplasmic domains that is a feature of many aspects of plant development; (Kim and Zambryski, 2005; Xu and Jackson, 2010). One weakness in much of this work is the availability of only relatively crude tools for measuring cell-to-cell trafficking. Generally, these are based upon altered trafficking of reporter molecules introduced locally by microinjection (small dyes) or more commonly now by microprojectile bombardment of surface cells with DNA clones expressing fluorescent reporters, such as green fluorescent protein (GFP) or derivatives. These assays measure the movement of non-native molecules, often in non-representative cells/tissues, and provide a measure of gross changes in mass flow (Crawford and Zambryski, 2001; Rutschow et al., 2011). What is urgently required is a collection of more sophisticated tools based upon inducible and tissue specific expression of native molecules. These could be provided by the new generations of chemically inducible expression vectors (Moller and Chua, 2002) or by the generation of phenotypic boundaries around genetic tissue sectors in transgenic plants (Wachsman and Heidstra, 2010) where the movement of molecules across the boundaries can be visualized and/or monitored as a phenotypic change.
Evidence points to callose accumulation as a mechanism for both quantitative and absolute control of cell-to-cell communication (Figure 2). The latter has been shown to be important in establishing symplasmic domains during development (e.g., Ruan et al., 2004; reviewed in Chen and Kim, 2009; Zavaliev et al., 2011). Callose accumulation results from the balanced activities of β-1,3-glucanases (glycosyl hydrolases) and callose synthases (CALS or glucan synthase-like – GSL) and its variable deposition at the neck of PD serves to regulate the mass symplasmic flow by constriction of the channel. These enzymes are the products of gene families and it is clear that only specific members of the families serve in the regulation of PD-associated callose. For example, Arabidopsis GSL8 (also called CALS10) is responsible for callose synthesis at PD (Guseman et al., 2010) and CALS7 for callose accumulation at phloem sieve pores, which are modified PD (Xie et al., 2011). For β-1,3-glucanases, only members of the glycosylphosphatidylinositol (GPI)-anchored glycosyl hydrolase family 17 have been associated with PD (Levy et al., 2007; Rinne et al., 2011); signals for GPI anchorage lead to tethering of the C-terminus of the protein to the external face of the PM appropriate for modification of callose deposits in the near cell wall. GPI-anchored proteins are also commonly found associated with the PM microdomains present within PD (Mongrand et al., 2010). Significant opportunities exist in understanding how and why only particular activities are targeted to or serve to catalyze PD-associated callose.
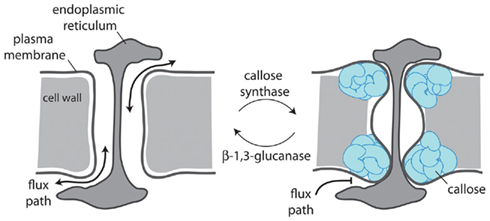
Figure 2. Plasmodesmal aperture is regulated by callose deposition in the cell wall surrounding the neck of the pore. Plasmodesmata-located callose synthases and β-1,3-glucanases control callose turnover which dictates whether a plasmodesma is open (left) or closed (right) and whether movement can occur freely between cells (left) or is obstructed (right).
Callose accumulation is also affected by alterations in cellular or local redox status for which, in turn, calcium signaling has been implicated (Benitez-Alfonso et al., 2010b). Redox status can be controlled at the cellular or subcellular levels by a suite of proteins that use thiol groups (e.g., thioredoxins; Benitez-Alfonso et al., 2009) or metal ions (e.g., cupredoxins) as electron donors/acceptors, or indirectly by proteins without obvious biochemical connection (Stonebloom et al., 2009, 2012; Burch-Smith and Zambryski, 2010). So far there is little published evidence that these proteins are active at or in PD, although antibodies to peroxidase enzymes have been shown to have reactivity in the locality of PD (Ehlers and van Bel, 2010). The importance of subcellular regulation of redox has been highlighted in recent work (Stonebloom et al., 2012) where plastidial and mitochondrial oxidation were shown to have opposing affects on molecular transport through PD.
Hence, emerging is a fragmented picture of PD and their function comprising PD-located proteins and ancillary activities connected with mechanisms of physical control of the symplasmic aperture and mechanisms of molecular translocation. Urgently needed are strategies with the potential to reveal functional networks of activity. These might come from further analysis of the PD proteome or from transcriptomic network analysis (Obayashi et al., 2007; Krouk et al., 2010) or a combination of the two; we have already identified candidate regulatory nodes arising from these combined approaches.
What we have tried to do in this article is to highlight the importance of PD for plant growth and to suggest priority areas for research interest and support. That cell-to-cell communication is a topic of keen interest to the plant community is highlighted by recent high profile publications that connect the process to aspects of plant development and RNA silencing (Molnar et al., 2010; Hyun et al., 2011). It is not yet however a topic that attracts the attention and interest that it deserves. The components of PD may seem trivial and obscure to those outside the field (Ruben, 2011), or at times within the field, but they are sophisticated and complicated structures that are absolutely fundamental to the multicellularity of plants. Without an understanding of the operational mechanics of these channels our understanding of plant development and environmental responses will, quite frankly, be limited. We are poised to make relevant and exciting advances in this field of biology that will impact on our understanding of all areas of plant growth and development.
Conflict of Interest Statement
The authors declare that the research was conducted in the absence of any commercial or financial relationships that could be construed as a potential conflict of interest.
Acknowledgments
John Innes Centre is grant-aided by the Biotechnology and Biological Sciences Research Council and supported by the John Innes Foundation.
References
Aoki, K., Kragler, F., Xoconostle-Cazares, B., and Lucas, W. J. (2002). A subclass of plant heat shock cognate 70 chaperones carries a motif that facilitates trafficking through plasmodesmata. Proc. Natl. Acad. Sci. U.S.A. 99, 16342–16347.
Benitez-Alfonso, Y., Cilia, M., San, R. A., Thomas, C., Maule, A., Hearn, S., and Jackson, D. (2009). Control of Arabidopsis meristem development by thioredoxin-dependent regulation of intercellular transport. Proc. Natl. Acad. Sci. U.S.A. 106, 3615–3620.
Benitez-Alfonso, Y., Faulkner, C., Ritzenthaler, C., and Maule, A. J. (2010a). Plasmodesmata: gateways to local and systemic virus infection. Mol. Plant Microbe Interact. 23, 1403–1412.
Benitez-Alfonso, Y., Jackson, D., and Maule, A. (2010b). Redox regulation of intercellular transport. Protoplasma 248, 131–140.
Burch-Smith, T. M., Stonebloom, S., Xu, M., and Zambryski, P. C. (2011). Plasmodesmata during development: re-examination of the importance of primary, secondary, and branched plasmodesmata structure versus function. Protoplasma 248, 61–74.
Burch-Smith, T. M., and Zambryski, P. C. (2010). Loss of INCREASED SIZE EXCLUSION LIMIT (ISE)1 or ISE2 increases the formation of secondary plasmodesmata. Curr. Biol. 20, 989–993.
Burch-Smith, T. M., and Zambryski, P. C. (2012). Plasmodesmata paradigm shift: regulation from without versus within. Annu. Rev. Plant Biol. 63, 2.1–2.22.
Chen, X. Y., and Kim, J. Y. (2009). Callose synthesis in higher plants. Plant Signal. Behav. 4, 489–492.
Christensen, N. M., Faulkner, C., and Oparka, K. (2009). Evidence for unidirectional flow through plasmodesmata. Plant Physiol. 150, 96–104.
Citovsky, V., McLean, B. G., Zupan, J. R., and Zambryski, P. (1993). Phosphorylation of tobacco mosaic virus cell-to-cell movement protein by a developmentally regulated plant cell wall-associated protein kinase. Genes Dev. 7, 904–910.
Crawford, K. M., and Zambryski, P. C. (2001). Non-targeted and targeted protein movement through plasmodesmata in leaves in different developmental and physiological states. Plant Physiol. 125, 1802–1812.
Ehlers, K., and van Bel, A. J. (2010). Dynamics of plasmodesmal connectivity in successive interfaces of the cambial zone. Planta 231, 371–385.
Faulkner, C., Akman, O. E., Bell, K., Jeffree, C., and Oparka, K. (2008). Peeking into pit fields: a multiple twinning model of secondary plasmodesmata formation in tobacco. Plant Cell 20, 1504–1518.
Faulkner, C., and Maule, A. (2011). Opportunities and successes in the search for plasmodesmal proteins. Protoplasma 248, 27–38.
Fernandez-Calvino, L., Faulkner, C., Walshaw, J., Saalbach, G., Bayer, E., Benitez-Alfonso, Y., and Maule, A. (2011). Arabidopsis plasmodesmal proteome. PLoS ONE 6, e18880. doi:10.1371/journal.pone.0018880
Furuta, K., Lichtenberger, R., and Helariutta, Y. (2011). The role of mobile small RNA species during root growth and development. Curr. Opin. Cell Biol. 24, 1–6.
Gerdes, H. H., and Carvalho, R. N. (2008). Intercellular transfer mediated by tunneling nanotubes. Curr. Opin. Cell Biol. 20, 470–475.
Guseman, J. M., Lee, J. S., Bogenschutz, N. L., Peterson, K. M., Virata, R. E., Xie, B., Kanaoka, M. M., Hong, Z., and Torii, K. U. (2010). Dysregulation of cell-to-cell connectivity and stomatal patterning by loss-of-function mutation in Arabidopsis chorus (glucan synthase-like 8). Development 137, 1731–1741.
Harries, P., and Ding, B. (2011). Cellular factors in plant virus movement: at the leading edge of macromolecular trafficking in plants. Virology 411, 237–243.
Hyun, T. K., Uddin, M. N., Rim, Y., and Kim, J. Y. (2011). Cell-to-cell trafficking of RNA and RNA silencing through plasmodesmata. Protoplasma 248, 101–116.
Kim, I., and Zambryski, P. C. (2005). Cell-to-cell communication via plasmodesmata during Arabidopsis embryogenesis. Curr. Opin. Plant Biol. 8, 593–599.
Kim, J. Y., Yuan, Z., Cilia, M., Khalfan-Jagani, Z., and Jackson, D. (2002). Intercellular trafficking of a KNOTTED1 green fluorescent protein fusion in the leaf and shoot meristem of Arabidopsis. Proc. Natl. Acad. Sci. U.S.A. 99, 4103–4108.
Kobayashi, K., Otegui, M. S., Krishnakumar, S., Mindrinos, M., and Zambryski, P. (2007). INCREASED SIZE EXCLUSION LIMIT 2 encodes a putative DEVH box RNA helicase involved in plasmodesmata function during Arabidopsis embryogenesis. Plant Cell 19, 1885–1897.
Krouk, G., Mirowski, P., Lecun, Y., Shasha, D. E., and Coruzzi, G. M. (2010). Predictive network modeling of the high-resolution dynamic plant transcriptome in response to nitrate. Genome Biol. 11, R123.
Lee, J. Y., and Lu, H. (2011). Plasmodesmata: the battleground against intruders. Trends Plant Sci. 16, 201–210.
Lee, J. Y., Wang, X., Cui, W., Sager, R., Modla, S., Czymmek, K., Zybaliov, B., van Wijk, K., Zhang, C., Lu, H., and Lakshmanan, V. (2011). A plasmodesmata-localized protein mediates crosstalk between cell-to-cell communication and innate immunity in Arabidopsis. Plant Cell 23, 3353–3373.
Levy, A., Erlanger, M., Rosenthal, M., and Epel, B. L. (2007). A plasmodesmata-associated beta-1,3-glucanase in Arabidopsis. Plant J. 49, 669–682.
Lucas, W. J., Ham, B. K., and Kim, J. Y. (2009). Plasmodesmata – bridging the gap between neighboring plant cells. Trends Cell Biol. 19, 495–503.
Moller, S. G., and Chua, N. H. (2002). Chemical regulated production of cDNAs from genomic DNA fragments in plants. Plant J. 32, 615–622.
Molnar, A., Melnyk, C. W., Bassett, A., Hardcastle, T. J., Dunn, R., and Baulcombe, D. C. (2010). Small silencing RNAs in plants are mobile and direct epigenetic modification in recipient cells. Science 328, 872–875.
Mongrand, S., Stanislas, T., Bayer, E. M., Lherminier, J., and Simon-Plas, F. (2010). Membrane rafts in plant cells. Trends Plant Sci. 15, 656–663.
Obayashi, T., Kinoshita, K., Nakai, K., Shibaoka, M., Hayashi, S., Saeki, M., Shibata, D., Saito, K., and Ohta, H. (2007). ATTED-II: a database of co-expressed genes and cis elements for identifying co-regulated gene groups in Arabidopsis. Nucleic Acids Res. 35, D863–D869.
Ormenese, S., Havelange, A., Bernier, G., and van der Schoot, C. (2002). The shoot apical meristem of Sinapis alba L. expands its central symplasmic field during the floral transition. Planta 215, 67–78.
Raffaele, S., Bayer, E., Lafarge, D., Cluzet, S., German Retana, S., Boubekeur, T., Leborgne-Castel, N., Carde, J. P., Lherminier, J., Noirot, E., Satiat-Jeunemaître, B., Laroche-Traineau, J., Moreau, P., Ott, T., Maule, A. J., Reymond, P., Simon-Plas, F., Farmer, E. E., Bessoule, J. J., and Mongrand, S. (2009). Remorin, a solanaceae protein resident in membrane rafts and plasmodesmata, impairs potato virus X movement. Plant Cell 21, 1541–1555.
Rinne, P. L., and Van der Schoot, C. (1998). Symplasmic fields in the tunica of the shoot apical meristem coordinate morphogenetic events. Development 125, 1477–1485.
Rinne, P. L. H., Welling, A., Vahala, J., Ripel, L., Ruonala, R., Kangasjärvi, J., and van der Schoot, C. (2011). Chilling of dormant buds hyperinduces FLOWERING LOCUS T and recruits GA-inducible 1,3-beta-glucanases to reopen signal conduits and release dormancy in Populus. Plant Cell 23, 130–146.
Ruan, Y. L., Xu, S. M., White, R., and Furbank, R. T. (2004). Genotypic and developmental evidence for the role of plasmodesmatal regulation in cotton fiber elongation mediated by callose turnover. Plant Physiol. 136, 4104–4113.
Ruben, A. (2011). Experimental error: fetus don’t fail me now. Sci. Career. doi: 10.1126/science.caredit.a1100046
Rutschow, H. L., Baskin, T. I., and Kramer, E. M. (2011). Regulation of solute flux through plasmodesmata in the root meristem. Plant Physiol. 155, 1817–1826.
Stadler, R., Wright, K. M., Lauterbach, C., Amon, G., Gahrtz, M., Feuerstein, A., Oparka, K. J., and Sauer, N. (2005). Expression of GFP-fusions in Arabidopsis companion cells reveals non-specific protein trafficking into sieve elements and identifies a novel post-phloem domain in roots. Plant J. 41, 319–331.
Stonebloom, S., Brunkard, J. O., Cheung, A. C., Jiang, K., Feldman, L. J., and Zambryski, P. C. (2012). Redox states of plastids and mitochondria differentially regulate intercellular transport via plasmodesmata. Plant Physiol. 158, 190–199.
Stonebloom, S., Burch-Smith, T., Kim, I., Meinke, D., Mindrinos, M., and Zambryski, P. (2009). Loss of the plant DEAD-box protein ISE1 leads to defective mitochondria and increased cell-to-cell transport via plasmodesmata. Proc. Natl. Acad. Sci. U.S.A. 106, 17229–17234.
Wachsman, G., and Heidstra, R. (2010). The CRE/lox system as a tool for developmental studies at the cell and tissue level. Methods Mol. Biol. 655, 47–64.
Xie, B., Wang, X., Zhu, M., Zhang, Z., and Hong, Z. (2011). CalS7 encodes a callose synthase responsible for callose deposition in the phloem. Plant J. 65, 1–14.
Xu, X. M., and Jackson, D. (2010). Lights at the end of the tunnel: new views of plasmodesmal structure and function. Curr. Opin. Plant Biol. 13, 684–692.
Xu, X. M., Wang, J., Xuan, Z., Goldshmidt, A., Borrill, P. G., Hariharan, N., Kim, J. Y., and Jackson, D. (2011). Chaperonins facilitate KNOTTED1 cell-to-cell trafficking and stem cell function. Science 333, 1141–1144.
Keywords: cell-to-cell communication, plasmodesmata, callose, molecular flux
Citation: Maule A, Faulkner C and Benitez-Alfonso Y (2012) Plasmodesmata “in communicado”. Front. Plant Sci. 3:30. doi: 10.3389/fpls.2012.00030
Received: 24 November 2011;
Accepted: 26 January 2012;
Published online: 10 February 2012.
Edited by:
Jose Manuel Estevez, University of Buenos Aires and CONICET, ArgentinaReviewed by:
Kian Hématy, Institut National de la Recherche Agronomique, FranceKen Kobayashi, University of Buenos Aires, Argentina
Copyright: © 2012 Maule, Faulkner and Benitez-Alfonso. This is an open-access article distributed under the terms of the Creative Commons Attribution Non Commercial License, which permits non-commercial use, distribution, and reproduction in other forums, provided the original authors and source are credited.
*Correspondence: Andy Maule, Department of Disease and Stress Biology, John Innes Centre, Norwich Research Park, Colney Lane, Norwich NR4 7UH, UK. e-mail:YW5keS5tYXVsZUBqaWMuYWMudWs=