- Biosciences Center, National Renewable Energy Laboratory, Golden, CO, United States
In Synechocystis sp. PCC 6803 (S. 6803), two types of phycobilisome (PBS) complexes, CpcG-PBS and CpcL-PBS, function to harvest light energy for photosynthetic reaction centers (RCs), photosystem I (PSI) and photosystem II (PSII). The compositional differences between these two forms of PBS and their specificity for RCs have led to suggestions that they may differ in function. To address this question, we examined how PBS-RC interactions, and the transfer of excitation energy from PBS to RCs, might be adjusted under conditions where electron demand and photon availability are modulated. The CpcG-PBS, CpcL-PBS, and RC complexes were isolated from a S. 6803 strain defective in expression of flavodiiron 1 (oxygen reduction reaction 1, ORR1) grown under varied light regimes. The energy transfer preference from CpcL-PBS to either PSI or PSII was investigated by in vitro crosslinking and 77 K fluorescence emission spectroscopy to assess energy transfer efficiency under photoexcitation. While the results demonstrate that the transfer of excitation energy from CpcL-PBS favors PSI over PSII in WT strains as previously shown, the preference of CpcL-PBS switches from PSI to PSII in ORR1 strains. Surprisingly, this change in preference was reproduced when ORR1 CpcL-PBS was crosslinked with WT RCs, or when WT CpcL-PBS was cross-crosslinked with ORR1 RCs, indicating there are physical modifications to both PBS and RCs that mediate the preference switch. In contrast, the analysis with ORR1 CpcG-PBS shows similar preferences to WT. Additionally, PBS populations in ORR1 shifted to a greater proportion of CpcL-PBS relative to CpcG-PBS. These results demonstrate that under conditions where electron utilization changes, there is a tuning of the excitation energy allocation from CpcL-PBS to RCs to manage the energy distribution for photosynthesis under dynamic flux conditions.
Introduction
Photosynthetic organisms utilize light energy to generate low potential reducing equivalents required for reductive chemistry. In cyanobacteria, phycobilisomes (PBS) harvest light and transfer the excitation energy to nearby reaction center (RC) complexes, Photosystem I (PSI) and Photosystem II (PSII), to drive the charge separation reaction (Bryant and Canniffe, 2018). In Synechocystis sp. PCC 6803 (S. 6803), there are two different types of PBS, CpcL-PBS and CpcG-PBS (Kaneko et al., 1996; Kondo et al., 2005; Bryant and Canniffe, 2018). The major molecular composition difference between the two types of PBS is that CpcL-PBS has a simplified structure consisting of one rod with only phycocyanin, compared to CpcG-PBS which has a set of six rods and consists of phycocyanin (PC) and allophycocyanin (APC) pigments (Kondo et al., 2005; Kondo et al., 2007; Watanabe et al., 2014). Although both types of PBS can transfer excitation energy to PSI and PSII, fluorescence studies with PBS deletion mutant strains showed that CpcL-PBS prefers PSI while CpcG-PBS prefers PSII under normal growth conditions (Kondo et al., 2007; Mullineaux, 2008). The differences in composition between CpcL-PBS and CpcG-PBS and more recent findings of increased ferredoxin-NADP+ reductase (FNR) and linker protein in CpcL-PBS compared to CpcG-PBS (Liu et al., 2019) have raised questions around how functionality may differ between the two forms of PBS (Liu et al., 2019; Niedzwiedzki et al., 2019).
The capacity to adjust excitation energy capture, transfer and dissipation by PBS and RC complexes is a major contributor to how photosynthetic organisms acclimate to changes in light quality/quantity and other growth conditions. Cyanobacteria have evolved ways to manipulate the interactions between PBS and RC complexes such as chromatic acclimation (Montgomery, 2017; Hirose et al., 2019), changes in expression of light harvesting antenna and RC proteins (Walters, 2005; Kopečná et al., 2012), dissipation of photon flux by non-photochemical quenching (Wilson et al., 2007), and redistribution of excitation energy between PSI and PSII by state transitions (Calzadilla and Kirilovsky, 2020). In addition to the capacity to fine tune photon energy allocation, cyanobacteria have various pathways to distribute reductant generated by photosynthesis. While linear electron flow (LEF) utilizes PSII, cytochrome b6f, PSI and ATP synthase to provide ATP and NADPH for downstream metabolic processes (Arnon, 1959; Allen, 2002; Munekage et al., 2004), cyclic electron flow (CEF) transfers electrons from PSI back into the plastoquinone (PQ) pool through ferredoxin to produce the additional ATP required to support carbon fixation (Takahashi et al., 2013; Shikanai and Yamamoto, 2017). Additionally, alternative electron flow (AEF) can be utilized to partition electrons into biochemical pathways that couple to substrates other than CO2 and have different energetic requirements (Demmig-Adams and Adams III, 1992; Shikanai and Yamamoto, 2017). The existence of multiple electron pathways functions to provide a suite of mechanisms for cells to manage the capture and allocation of light energy with variations in electron flow.
Alternative electron accepting pathways downstream of PSI include an O2 reduction reaction (ORR) catalyzed by flavodiiron 1 (Flv1). Analysis of a S. 6803 mutant strains deficient in this pathway (ORR1) showed evidence of changes in PSI oligomeric, spectral, and photochemical properties, which were exasperated under fluctuating light (5 minutes 30 µmol photons m-2 s-1, 30 seconds 500 µmol photons m-2 s-1) (Vicente et al., 2002; Helman et al., 2003; Allahverdiyeva et al., 2013; Brown et al., 2020; Smolinski et al., 2022). Collectively, this work indicated a flexibility in the capacity to tune the generation and supply of photoexcited electrons at PSI. It remains unclear whether there are compensating changes in the preference for transfer of excitation energy from PBS to RCs under these conditions (Figure 1). Elucidating the molecular basis of how these mechanisms are interconnected will enable a better fundamental understanding of energy transformation in photosynthetic systems.
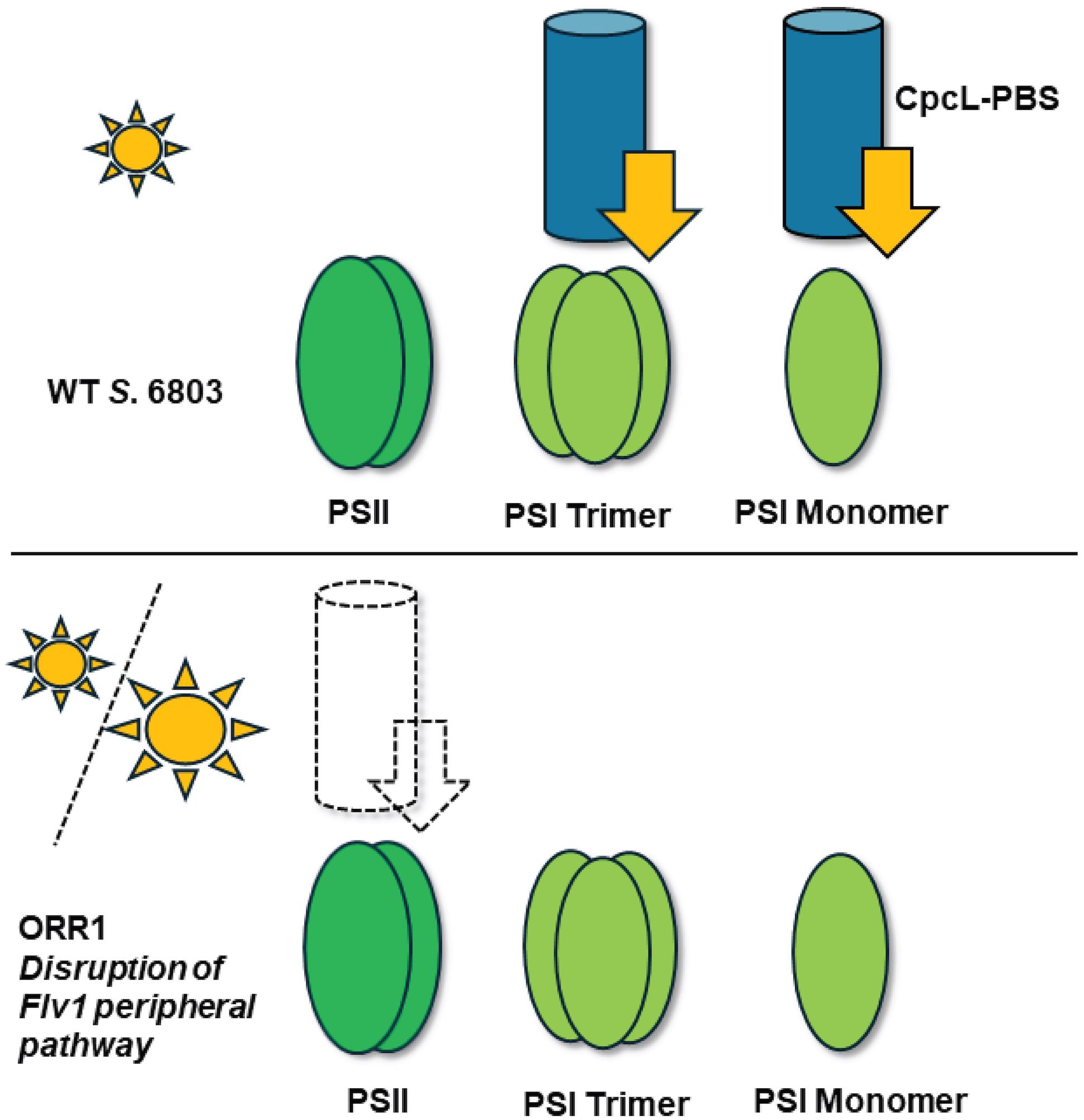
Figure 1. Model of energy transfer preferences from CpcL-PBS (blue cylinder) to RCs (green ovals) in WT S. 6803 (top) and ORR1 (bottom). Unknown energy transfer preferences occur in the ORR1 strain, where the dashed lines represent the hypothesized transfer preferences examined in this study. Energy transfer from PBS to RCs is shown as arrows. Sun size indicates light quantity where small sun is moderate and large is increased photon flux.
To address if there is a change in preference of PBS for RCs to modulate excitation energy transfer under dynamic changes in electron demand, we investigated the energy transfer interactions between isolated PBS and RC complexes from the S. 6803 WT and ORR1 strains grown under moderate (30 µmol photons m-2 s-1) and fluctuating light regimes. Here we focused on assessing the preference for excitation energy transfer from CpcL-PBS to RCs, which we hypothesized adjusts to compensate in response to changes in light quality and electron flow demand. Using in vitro crosslinking to stabilize the interactions between the CpcL-PBS and RC proteins, we demonstrated that excitation energy transfer from CpcL-PBS to PSI, the preferred reaction in WT cells, was strongly attenuated in ORR1. This was accompanied by an increase in energy transfer to PSII compared with the WT strain. Crosslinking CpcL-PBS from one strain (e.g. WT) to the RC proteins from the other strain (e.g. ORR1) demonstrated that these adjustments in preference were retained, suggesting there are molecular determinants for the preference switch on both CpcL and RCs. These findings of dynamic energy transfer preferences of CpcL-PBS under conditions of modified electron utilization point to a functional role of CpcL-PBS in adjusting the management of light harvesting in response to dynamic conditions.
Materials and methods
Strains and culture conditions
Synechocystis sp. PCC 6803 WT and a strain deficient in ORR, ORR1, were used for experimentation. The ORR1 strain was generated by knocking out the flv1 gene encoding flavodiiron 1, as described in (Smolinski et al., 2022). Synechocystis sp. PCC 6803 WT and ORR1 strains were grown in 5 mM N-Tris(hydroxymethyl)methyl-2-aminoethanesulfonic acid (TES)-NaOH, pH 8.2 buffered BG-11 (Allen, 1968) media under continuous light (35 µmol photons m-2 s-1) at room temperature with 3% CO2. For the fluctuating light condition, cells were first grown under constant light of 35 µmol photons m-2 s-1 for 3 days, then shifted to cycles of 35 µmol photons m-2 s-1 for 5 min, and 500 µmol photons m-2 s-1 for 30 s, for a total of 4 days of growth.
Isolation of PSI monomer/PSII and PSI trimer fractions
Thylakoid membranes (TMs) were isolated from the cells similar to Li et al (Li et al., 1991). Briefly, all procedures were performed at 4 °C. Cells were spun down at 5,000 x g for 10 min, and the pellet was resuspended in buffer containing 20 mM 4-(2-hydroxyethyl)-1-piperazineethanesulfonic acid (HEPES)-NaOH, pH 7.5; 10 mM CaCl2; 10 mM MgCl2 and 10 mM NaCl (buffer A). Cells were lysed by three passes through a French Press cell, while kept on ice. After the cell lysate was spun down at 5,000 x g for 10 min, the supernatant was collected and then spun at 208,000 x g for 1 h (Type-70Ti rotor, Beckman Coulter). The pellet containing TMs was resuspended in buffer A to approximately 1 mg chl ml-1. TMs were incubated with 1% n-dodecyl-β-D-maltoside (DDM) (GoldBio) for 2 h in darkness, and the chlorophyll (chl) concentration was adjusted to 0.4-0.5 mg ml-1. Solubilized TMs were centrifuged at 12,000 x g for 20 min to pellet non-solubilized material. The supernatant was then loaded on to 5-30% (w/vol) linear sucrose gradient with buffer A containing 0.01% DDM. The gradient was centrifuged at 110,000 x g, overnight. Each colored fraction was carefully collected from the top of the sucrose gradient. The PSI monomer/PSII and PSI trimer fractions were further concentrated by 10 kD cutoff filter (Amicon Ultra-15, 10 kD cutoff, Sigma), subsequently flash frozen in liquid nitrogen and stored in -80°C freezer.
Isolation of CpcL-PBS and CpcG-PBS
CpcL-PBS was isolated from cells according to Hirose et al (Hirose et al., 2019). The following experiments were performed at room temperature (RT). The harvested cells were spun down at 5,000 x g for 10 min and then the pellets were resuspended in 0.85 M phosphate buffer, pH 7.0 twice. The cells were broken by passing through French press cell 3 times. The cell homogenates were centrifuged at 5,000 x g for 10 min. The supernatants were incubated with 2% (v/v) Triton X-100 and 0.5% (w/v) DDM in the dark for 45 min. After incubation, the mixtures were loaded onto 5-30% (w/vol) linear sucrose gradient with 0.85 M phosphate buffer, pH 7.0 containing 0.1% Triton and 0.1% DDM. The gradients were centrifuged at 110,000 x g at RT overnight. The colored fractions were carefully collected from the top of the sucrose gradient. The CpcL-PBS and CpcG-PBS were wrapped with foil and stored in 4°C fridge until further use.
SDS-PAGE
The PBS fractions were concentrated and exchanged into low phosphate buffer by 3 kD cutoff filter (Amicon, Ultra-0.5, 3 kD cutoff, Sigma) @ room temperature. Before denaturing the samples, the amounts of PBS were normalized to the same PC absorption peak. Proteins were incubated on ice with 20% (v/v) trichloroacetic acid (TCA) for 1 h and then spun down @ 17,300 x g for 30 min. The pellets were resuspended in 10% (v/v) TCA once then acetone twice by spinning the samples down @ 17,300 x g for 10 min. The final pellets were resuspended with 62.5 mM Tris-HCl pH 6.8, 2% (w/v) sodium lauryl sulfate, 5% (w/v) sucrose, 0.005% (w/v) bromophenol blue and 100 mM dithiothreitol and the mixtures were incubated at 65°C for 3 min. The reaction mixtures were loaded onto a pre-made gel (Any kD™ Mini-PROTEAN® TGX Stain-Free™ Protein Gels #4568124) and run for 30 min at 200 V.
The amounts of PSI were normalized to the same amount of chl concentration. Proteins were added to a 1:1 mixture with 2X SDS-PAGE sample buffer (Bio-Rad 2x Laemmli Sample Buffer #1610737) with 5% (w/v) 2-mercaptoethanol, and incubated for 10 minutes at 99°C. The sample mixtures were loaded onto a pre-made gel (Any kD™ Mini-PROTEAN® TGX Stain-Free™ Protein Gels #4568124) and run for 65 min at 120 V.
In vitro crosslinking of CpcL-PBS or CpcG-PBS and PSI monomer/PSII or PSI trimer
In vitro crosslinking experiments were performed similar to Hartmann et al (Hartmann et al., 2020). Isolated CpcL-PBS or CpcG-PBS were incubated with 500 µM 1,4-butanediol diglycidyl ether (BDDE) at 4°C for 3 h in the dark. The excess BDDE was removed by overnight dialysis (14 kD cutoff) to avoid potential crosslinking among RCs. Under this condition, only PBS was crosslinked with RCs. The concentrations of CpcL-PBS and CpcG-PBS from different strains growing under different conditions were normalized to 0.21 µM PC. The concentrations were determined by UV-Vis spectroscopy using an extinction coefficient for PC of ~3 × 105 M−1 cm−1 (Niedzwiedzki et al., 2019).
PSI monomer/PSII and PSI trimer fractions were normalized to the same estimated P700 content similar to Smolinski and Lubner et al (Smolinski et al., 2022). Fluorescence emission spectra at 77 K were measured with a Fluorolog-3 (HORIBA Scientific) using 450 nm excitation wavelength (excitation slit of 5 nm) and emission at 730 nm (emission slit of 1 nm), and isolated PSI samples at a chl concentration of 3 μg mL-1. Chl concentration was based on absorbance at 663 nm by diluting 10 μL sample in 990 μL methanol, A663 x (100/82). Maximum fluorescence emission intensity at approximately 718 nm and 685 nm were used to determine the ratio of chl in PSI and PSII. Estimated P700 was based on the amount of chl in the sample attributable to PSI. In the diluted PSI trimer samples, P700 (nmol) was determined by: (chl concentration of diluted sample ÷ 893.51) ÷ 95. P700 in the diluted PSI monomer samples (nmol) was determined by: [(chl concentration of diluted sample x (ratio for PSI: PSII x 95)) ÷ ((ratio for PSI: PSII x 95) + (ratio value for PSII, which is 1 x 35) ÷ 893.51] ÷ 95. The number of chl molecules in PSI monomeric unit: 95. The number of chl molecules in PSII: 35. The molecular weight of chl: 893.51 g mol-1. Estimated P700 in the stock PSI monomer or trimer sample (nmol) was based on the amount of chl, as determined by: [(nmol P700+ in diluted sample for fluorescence emission spectra samples) ÷ (3 μg mL-1 chl)] x (chl concentration of non-diluted PSI sample). In the samples for cross-linking analysis, the final chl concentration of PSI was 0.21 µM (i.e. estimated PSI content or chlPSI).
PBS and RC fractions were mixed in a molar ratio of PC-to-chlPSI equaling to 1:1. The mixture was incubated for more than 10 min at room temperature and used for further analysis.
Fluorescence spectra
Fluorescence excitation and emission spectra at 77 K were measured with a Fluorolog-3 (HORIBA Scientific). Whole cells (2.5 µg chl ml-1) or crosslinked PBS-RC samples (at concentrations indicated above) were transferred into a quartz EPR tube with 200 µL volume and slowly frozen in liquid nitrogen. Excitation wavelengths were 450 nm or 580 nm (slit width of 5 nm) to excite chl or PBS, respectively. The emission spectra were recorded between 600 nm and 850 nm or 750 nm (slit width of 1 nm) for whole cells or crosslinked samples, respectively.
Results
PBS composition and spectral characteristics
ORR1 strains cultured under standard photon flux (GL, 35 µmol photons m-2 s-1) conditions exhibited WT-like growth. However, under fluctuating light (FL, 35 µmol photons m-2 s-1 for 5 min with 500 µmol photons m-2 s-1 for 30 s) the growth rate of the mutant was attenuated, consistent with a mismatch between photon and electron flux (Smolinski et al., 2022). Whole cell 77 K fluorescence emission spectra suggested more energy directed toward PSII in ORR1 under either light regime (Supplementary Figure S1), indicating potential differences in PBS preferences however the reasons for this were not apparent from this data alone. A change in the composition of the PBS forms across the strains was observed by sucrose density gradients (Supplementary Figure S2), which shifted to an increased amount of CpcL-PBS in ORR1 GL and FL (Table 1). This observation is consistent with our hypothesis of CpcL-PBS having a greater role in managing photon and electron flow balance in this strain.
To measure the potential changes of PBS complexes from WT and ORR1 strains, we used sucrose gradients to isolate CpcG- and CpcL-PBS in cells cultured under GL and FL conditions (Supplementary Figure S2). The absorption spectra of CpcL-PBS showed a PC peak at around 620 nm (Figure 2A). The 77 K fluorescence emission spectra of CpcL-PBS (Figure 2B), excited at 580 nm, displayed the typical CpcL-PBS spectral signatures at 650 nm, which is the blue PC rod, and 670 nm, which is the typical terminal emitter of CpcL-PBS. Aside from intensity changes, the data exhibit no obvious changes in the spectral characteristics of CpcL-PBS in ORR1 cells cultured under either light condition. SDS-PAGE analysis of isolated CpcL-PBS did not provide evidence of substantial compositional changes in ORR1 compared to WT (Supplementary Figure S3).
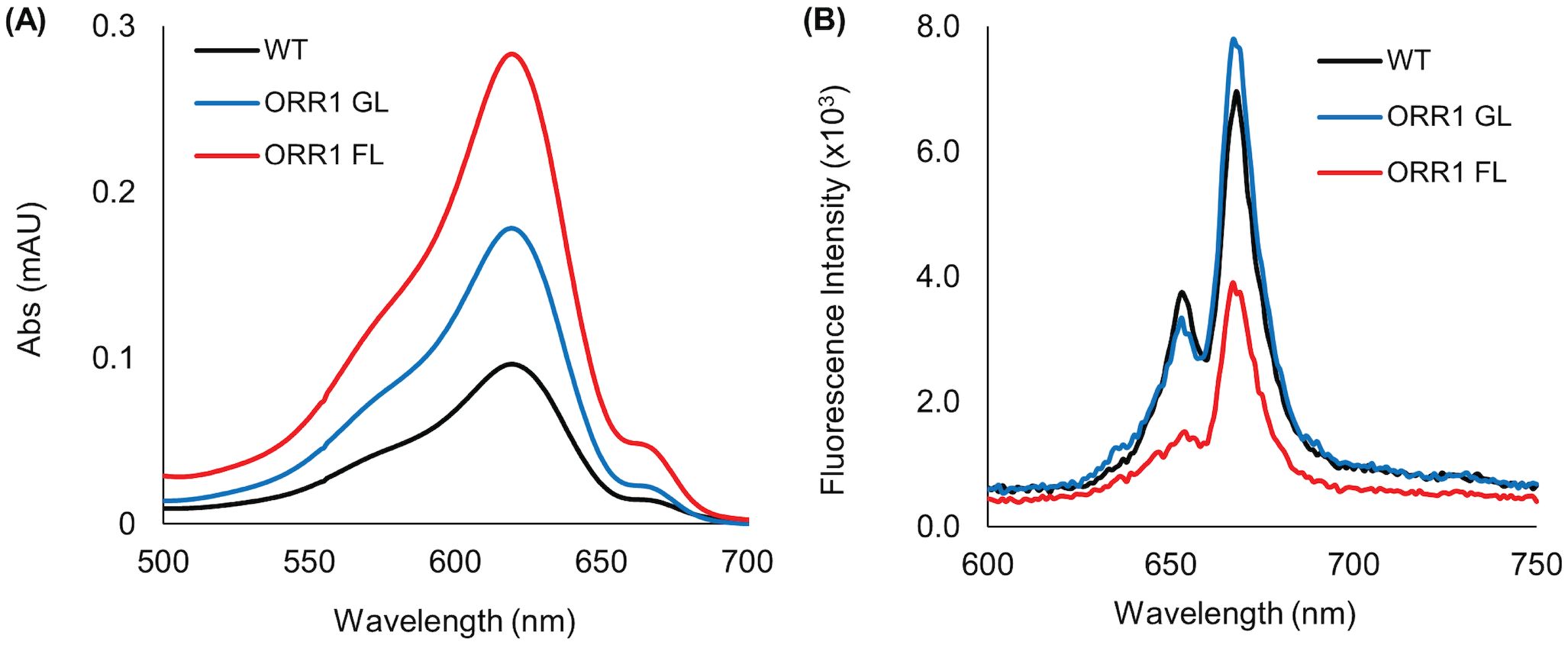
Figure 2. Optical properties of isolated CpcL-PBS from WT and ORR1 strains. (A) UV/Vis absorption spectra of CpcL-PBS isolated from S. 6803 WT GL, ORR1 GL and ORR1 FL conditions, shifted to zero at 700 nm. (B) Fluorescence emission spectra of CpcL-PBS using an excitation wavelength of 580 nm. The sample concentrations were normalized to the approximate same PC absorption at 620 nm.
Energy transfer preference of CpcL-PBS shifts to PSII in ORR1
Having established that there is an enrichment of CpcL-PBS in ORR1, we utilized isolated CpcL-PBS and RC proteins (PSI monomer/PSII and PSI trimer) (Supplementary Figure S4) to assess whether there are changes in the preference for energy transfer between CpcL-PBS and RCs (Wilson et al., 2007). SDS-PAGE analysis of the isolated PSI monomer/PSII and PSI trimer fractions, did not indicate substantial compositional changes between WT and ORR1 (Supplementary Figure S5). Furthermore, our previous in vitro studies of ORR1 PSI showed no evidence of PSI photoinhibition (Smolinski et al., 2022). The ability of CpcL-PBS to bind and transfer energy to RCs was tested by in vitro crosslinking of PBS and RCs from the same strains, using normalized component levels (i.e., chlPSI and PC) (Supplementary Figure S6). We used 77 K fluorescence spectroscopy with excitation at 580 nm to detect CpcL-PBS excitation energy transfer to RCs as a relative intensity difference (Wilson et al., 2007). Because fluorescence yield can vary depending on the chemical environment of the components (Niedzwiedzki et al., 2019), we conducted all experimentation in the same buffer composition to limit artifactual intensity changes. The relative intensity differences in ORR1 versus WT that are observed in the 77 K fluorescence spectra (Figure 3) are consistent with a change in the CpcL-PBS preference for excitation energy transfer from PSI to PSII in ORR1 (Figure 3, Table 2). This change is further enhanced in ORR1 cultured under FL conditions.
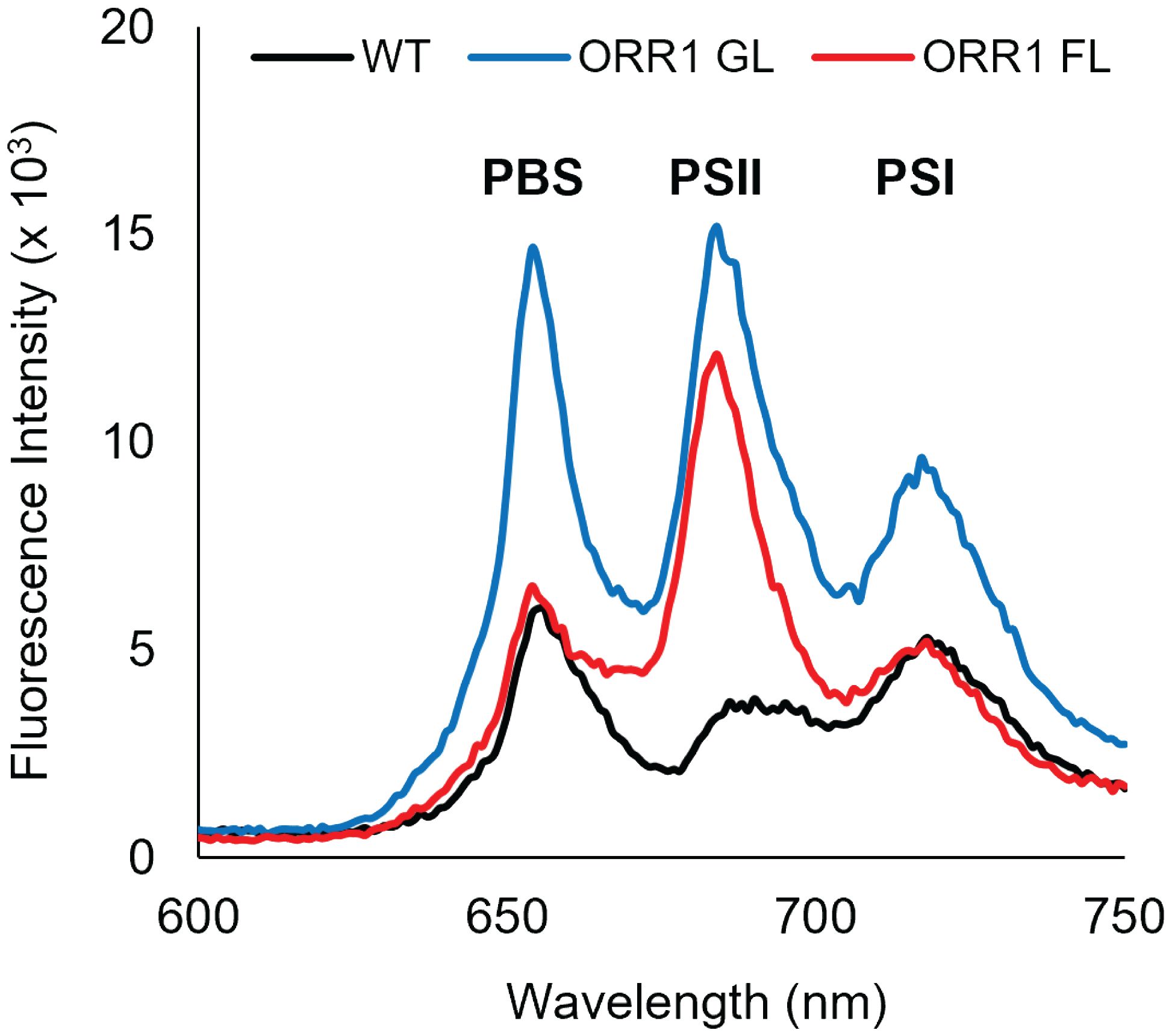
Figure 3. 77 K fluorescence emission spectra showing energy transfer from CpcL-PBS to PSI monomers and PSII, for PBS crosslinked with RCs from the same strains. WT GL CpcL-PBS crosslinked with WT GL PSI monomer/PSII (black), ORR1 GL CpcL-PBS crosslinked with ORR1 GL PSI monomer/PSII (blue), and ORR1 FL CpcL-PBS crosslinked with ORR1 FL PSI monomer/PSII (red). The excitation wavelength was 580 nm. The spectra are an average of two to three samples. Before in vitro crosslinking, the RCs were normalized to the same estimated PSI concentration, and the CpcL-PBS were normalized to the same PC concentration.

Table 2. Summary of energy transfer preferences of CpcL-PBS from WT and ORR1, crosslinked with PSII and PSI monomer isolated from the same strain.
Our previous study found that the ratio of PSI: PSII remains similar for WT and ORR1 for both light regimes (Smolinski et al., 2022), demonstrating that the relative abundance of PSI and PSII does not change in whole cells. PSI content, however, shifts from primarily trimeric in WT (14% monomeric), to slightly increased monomeric in ORR1 GL (18%), to significantly increased monomeric forms in ORR1 FL (34%). This increase in PSI monomerization observed for ORR1 FL translates to an enrichment of PSI monomers relative to PSII in the PSI monomer/PSII fraction from ORR1 FL. Despite the increase in PSI monomer amount, we still observed a shift toward more energy transfer with PSII, displaying that the preference shift is independent of the total amount of RC present in the sample and rather depends on changes specific to the CpcL-PBS, RCs, and/or their interactions. Further supporting this interpretation is that WT and ORR1 GL, which have similar ratios of PSI monomers to PSII, display opposite behaviors with WT exhibiting a preference for PSI monomers as reported previously (Kondo et al., 2005; Kondo et al., 2007) and ORR1 GL preferring PSII. Furthermore, analysis of CpcG-PBS showed the energy transfer preference for PSII that is observed for WT was maintained in ORR1, under both light regimes (Supplementary Figure S7, Supplementary Table S1). Thus, the combined effects of variation in light and electron utilization on energy transfer during photosynthesis are isolated to changes in the CpcL-PBS interactions with RCs.
Excitation of crosslinked CpcL-PBS and PSI trimers isolated from ORR1 shows the peak PSI fluorescence emission intensities are similar to WT, but slightly decreased (Figure 4, Table 3). When compared to PSI monomers (Figure 3, Table 2), the lower peak intensities for PSI trimers could imply favored energy transfer from CpcL-PBS to PSI monomers than to PSI trimers.
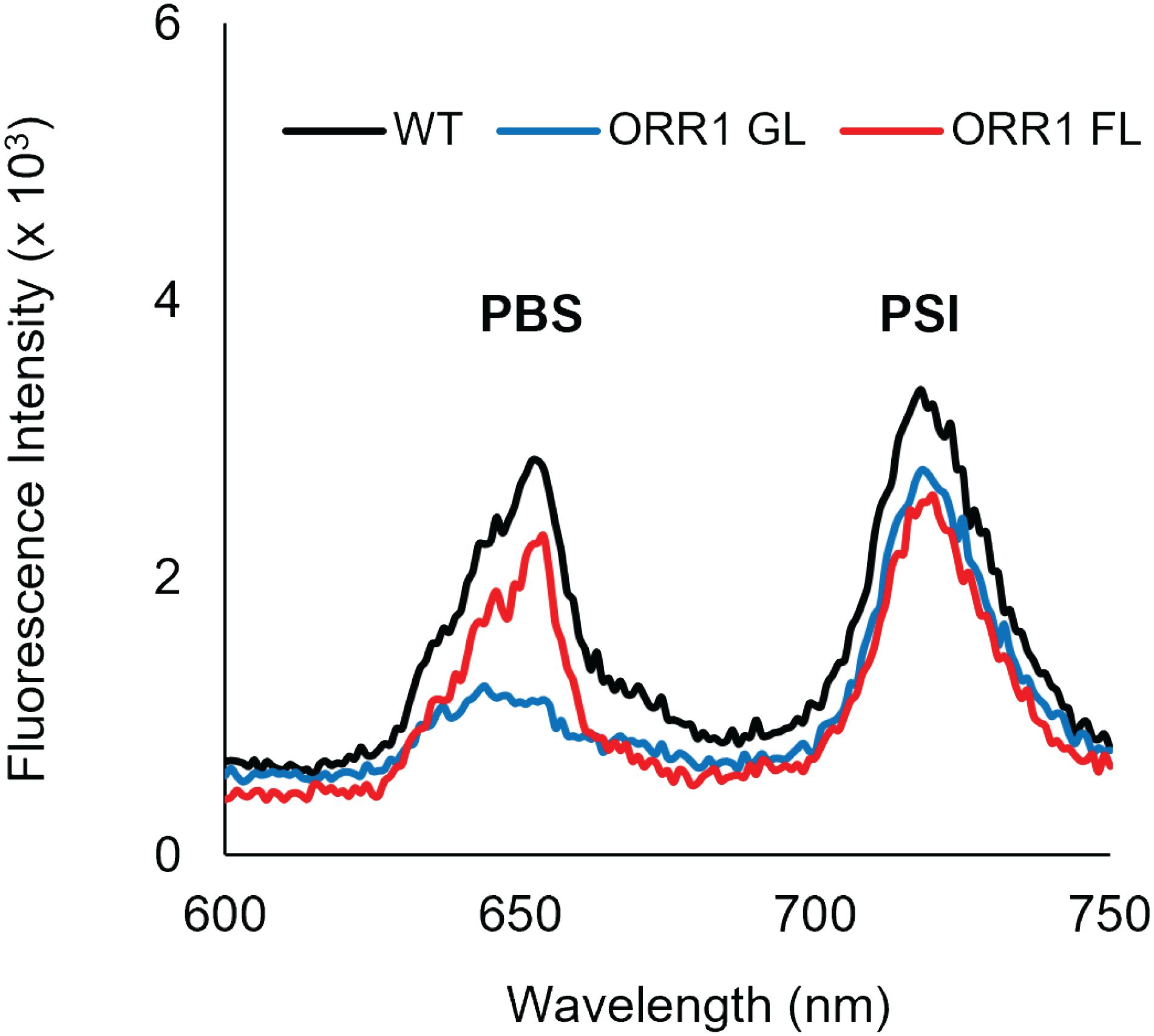
Figure 4. 77 K fluorescence emission spectra showing energy transfer from CpcL-PBS to PSI trimers, for PBS crosslinked with RCs from the same strains. WT GL CpcL-PBS crosslinked with WT GL PSI trimers (black), ORR1 GL CpcL-PBS crosslinked with ORR1 GL PSI trimers (blue), and ORR1 FL CpcL-PBS crosslinked with ORR1 FL PSI trimers (red). The excitation wavelength was 580 nm. The spectra are an average of two to three samples. Before in vitro crosslinking, the RCs were normalized to the same estimated PSI concentration, and the CpcL-PBS were normalized to the same PC concentration.
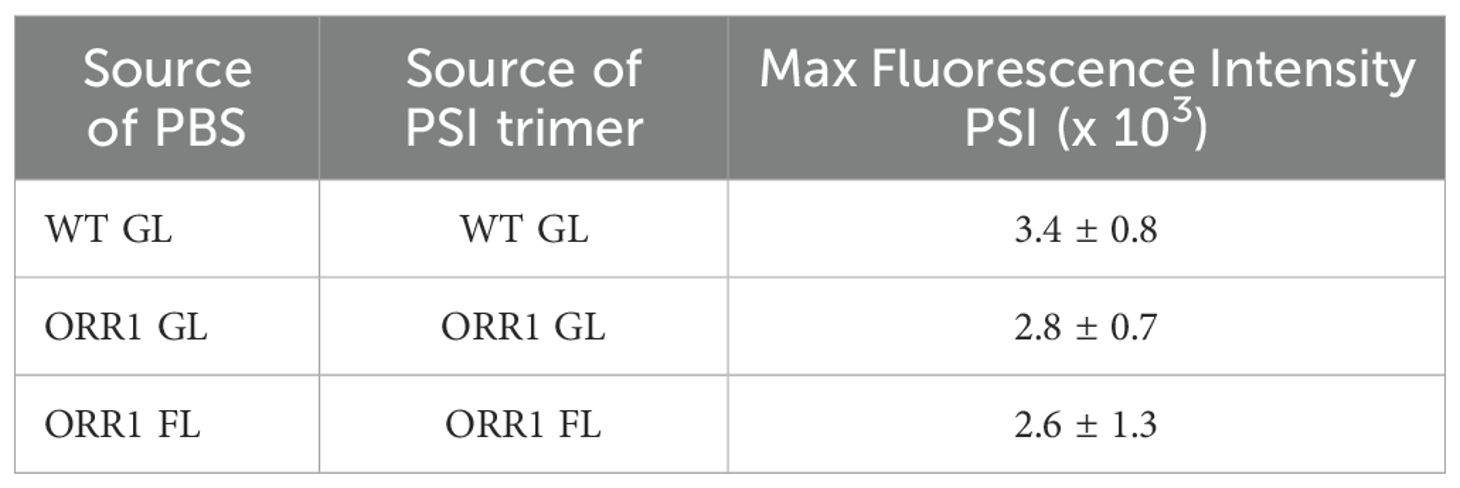
Table 3. Summary of energy transfer preferences of CpcL-PBS from WT and ORR1 crosslinked with PSI trimer isolated from the same strain.
Both CpcL-PBS and RCs modulate energy transfer interactions
The differences in the observed excitation energy distribution in ORR1 versus WT strains might be due to modifications to the PBS or RC components in ORR1 that change preferences. To test this, we performed experiments where the PBS or RC component of ORR1 was crosslinked with the corresponding component from the WT strain and measured energy transfer as the fluorescence intensity. When WT CpcL-PBS was crosslinked with ORR1 PSI monomer/PSII (Figure 5, black dashed line), or ORR1 CpcL-PBS was crosslinked with WT PSI monomer/PSII (Figure 5, blue dashed line), there was a slight preference for PSII over PSI monomer (Table 4). The results are consistent with the preference observed for ORR1-only crosslinking experiments where CpcL-PBS preference is for PSII in ORR1 (Figure 5, blue solid line) but is reversed from the WT-only crosslinking experiment which shows preference for PSI (Figure 5, black solid line). These results demonstrate that determinants from both CpcL-PBS and PSI determine the preference change in ORR1. There was a greater amount of uncoupled CpcL-PBS (Figure 5, PBS peak at 650 nm) for cross-strain versus same-strain crosslinked reactions, further supporting that modifications to both components underpin the energy transfer activity.
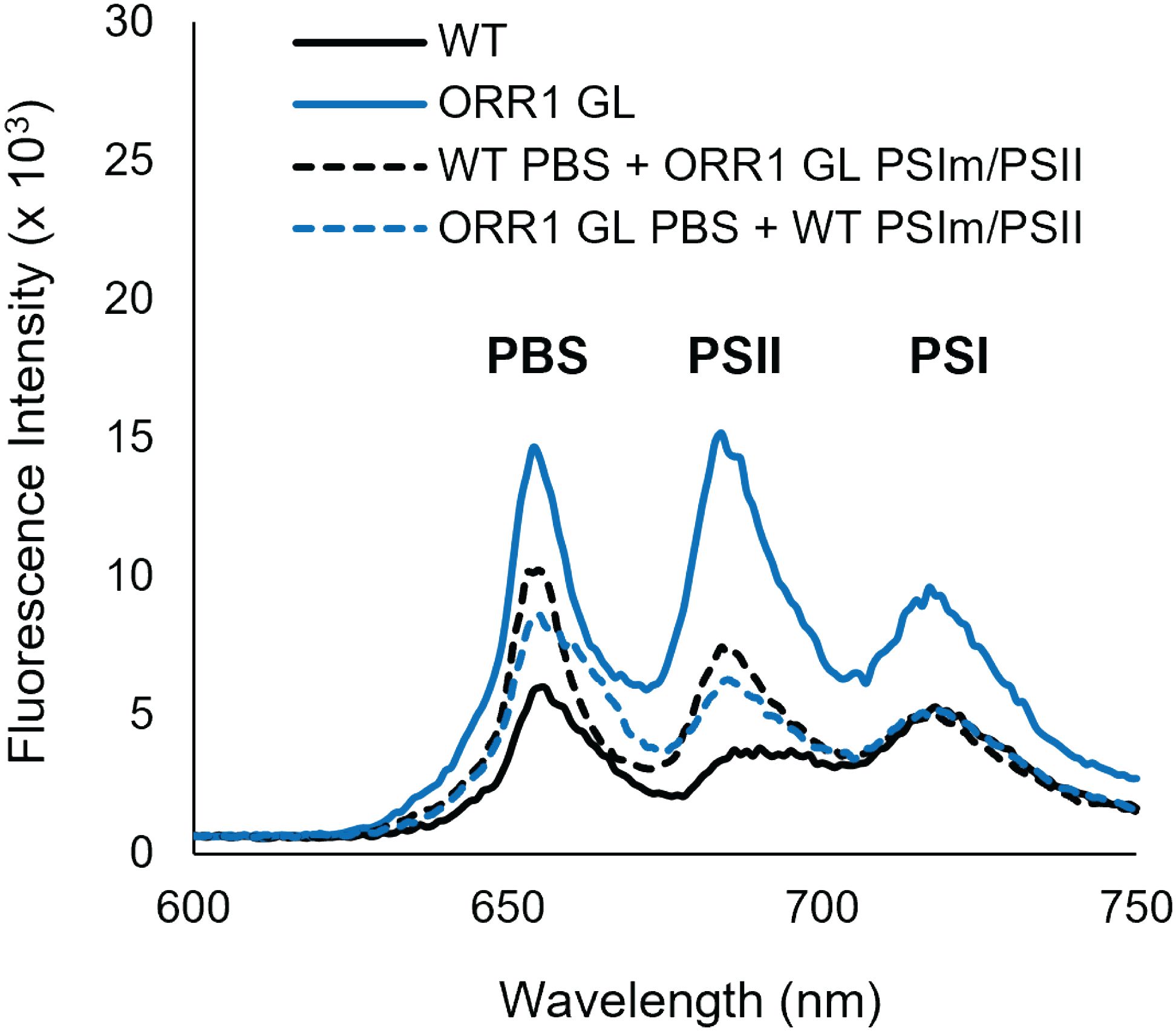
Figure 5. 77 K fluorescence emission spectra showing energy transfer from CpcL-PBS to PSI monomers and PSII for PBS crosslinked with RCs from the same strains (solid lines), and for PBS crosslinked with RCs from different strains (dashed lines). WT GL CpcL-PBS crosslinked with WT GL PSI monomers/PSII (black solid), ORR1 GL CpcL-PBS crosslinked with ORR1 GL PSI monomers/PSII (blue solid), WT GL CpcL-PBS crosslinked with ORR1 GL PSI monomers/PSII (black dashed) ORR1 GL CpcL-PBS crosslinked with WT GL PSI monomers/PSII (blue dashed). The excitation wavelength was 580 nm. The samples have the same PBS and PSI content. The spectra are an average of two to three samples.

Table 4. Summary of energy transfer preferences of CpcL-PBS from WT and ORR1 crosslinked with PSII or PSI monomer isolated from the other strain.
The preference of CpcL-PBS to transfer energy to PSII over PSI monomers in ORR1 was also consistent when ORR1 CpcL-PBS was crosslinked to ORR1 RCs from the different light regimes (GL and FL) (Supplementary Figure S8, Supplementary Table S2). The crosslinking analysis of CpcG-PBS with RCs demonstrated that the preference for PSII over PSI was maintained for all strains under all light conditions (Supplementary Figure S9, Supplementary Table S3).
Discussion
Living in aqueous environments, Cyanobacteria usually face highly dynamic conditions with mismatches between photon and electron flux frequently occurring. Previously it has been shown that cyanobacteria could regulate the abundance of PBSs to adjust for environmental changes, for example under various light intensities or nutrient limitation conditions (Chitnis et al., 1995; Akhtar et al., 2022). However, whether there are mechanisms for balancing of photon flux between the RCs under changing conditions is not fully known. Here we studied energy transfer from PBS to RCs under the loss of a peripheral electron pathway and under increased and fluctuating photon flux. The outcome of this approach has revealed new insights into the control of PBS-RC binding preference and its functional role in adjusting excitation energy transfer in response to changes in electron utilization.
A key finding of our work is that mismatches in photon and electron flux, generated through the deletion of the ORR pathway, lead to pronounced changes in PBS composition and their preferences for reaction centers. We found increased levels of CpcL-PBS in ORR1 cultured under both GL and FL conditions compared with WT, and with emissive properties consistent with functionally intact PBS. While CpcG-PBS energy transfer preferences were unchanged between WT and ORR1 GL and FL, marked changes in CpcL-PBS preferences were observed in ORR1. Consistent with previous reports (Kondo et al., 2005; Kondo et al., 2007), we observed a preference for excitation energy transfer of CpcL-PBS with PSI in the WT strain. However, in ORR1 this behavior is altered to preferentially transfer energy to PSII over PSI monomers. When ORR1 cells are grown under FL conditions, which further intensifies the energy mismatch, the preference for PSII is more pronounced. Our previous work on the ORR1 strain highlighted several important modifications to PSI reaction centers, namely an increase in the monomeric form and decreased photooxidative capacity, which both became more pronounced under FL conditions (Smolinski et al., 2022). In the ORR1 strain, the population of PSI in the monomeric form is similar to WT under GL conditions (18% vs 14% for ORR1 and WT) but shifts to an increasing population of monomers (34%) under FL conditions (Smolinski et al., 2022). The stronger interaction between CpcL-PBS and PSI monomers in ORR1 GL vs ORR1 FL may arise from the tuning of PBS preferences to maintain energy transfer capacity with both PSI and PSII. When energy mismatch is severely affected as in ORR1 under FL conditions, the shift in CpcL-PBS preference is more pronounced for directing more excitation energy away from PSI and towards PSII. Therefore, the modification to the CpcL-PBS preferences in ORR1 could result from a need to re-direct photon energy toward other dissipative mechanisms in order to protect PSI when there is decreased electron utilization demand downstream of PSI.
CpcL-PBS retained the capacity to interact with PSI trimers, showing minimal differences between WT, ORR1 GL or ORR1 FL. While the fluorescence emission intensities showed overall lower emission from PSI trimers compared to PSI monomers, despite these experiments being performed at the same chlPSI concentration, the preference of CpcL-PBS for specific ORR1 PSI oligomeric forms remains unclear. On one hand, the increased emission intensity from PSI monomers (Figure 3) vs PSI trimers (Figure 4) suggests more energy transfer to PSI monomers, however the decreased emission from uncoupled PBS in the PSI trimer spectra could imply more efficient energy transfer to PSI trimers, particularly for ORR1 GL. Direct evaluation of the preference for PSI oligomeric form presents experimental challenges due to the lack of specific emission signatures from PSI monomers and trimers. In our experiments, the inclusion of PSII centers within the PSI monomer sample may obscure some of the contributions to the CpcL-PBS preference, i.e. direct comparison between PSI monomers and PSI trimers is not straightforward. Further investigation will be required to more clearly interrogate CpcL-PBS energy transfer with the various PSI forms.
Crosslinking experiments revealed that the modifications in preference were maintained when either CpcL-PBS or reaction centers from ORR1 under each light condition were used with the complementary components from WT, indicating physical alterations to the CpcL-PBS and RCs due to the lack of the ORR activity in this strain. Because the preference was maintained when the reverse pairing was utilized, this suggests that both the CpcL-PBS and the RCs are physically modified to support the altered interactions. This further suggests a dynamic adjustment in the relative abundance, structure, and interactions of multiple photosynthetic components in parallel to allow for the modulation of light harvesting and energy transfer. While further investigation is needed to explore potential structural and compositional adjustments in CpcL-PBS and PSI in ORR1, the evidence of differences in both CpcL-PBS and PSI in ORR1 provide insights into the complex dynamic relationships across components which coordinate to tune electron flux in the photosynthetic electron transport chain. Future work should include proteomic analyses of both the PBS and PSI components in ORR1 to elucidate the structural determinants underlying the observations of altered CpcL-PBS preference reported here. Specifically, post translational modifications, subunit depletions or alterations to subunit environments could be explored as possible mechanistic contributions to altered energy transfer or PBS/RC interaction properties.
The collective outcomes of this work demonstrate that there is a shift in the preference of excitation energy transfer from CpcL-PBS to PSII that functions as a mechanism to modulate the amount of excitation energy provided to PSI when there is a decreased demand for electrons downstream from PSI. In this way, CpcL-PBS can tune energy transfer in response to dynamic changes in electron flow, contributing to the photosynthetic energy management mechanisms available to the cell. As more knowledge is gathered regarding CpcL-PBS structural and functional properties (Liu et al., 2019; Niedzwiedzki et al., 2019; Liu, 2023; Zheng et al., 2023), together with the understanding of how the relative abundance and preferred energy transfer from PBS forms can be adjusted, advances in defining the molecular mechanisms that allow for the adaptive management of photosynthetic energy flux will be realized. In turn, this fundamental knowledge can inform the design of applied photosynthetic systems for renewable energy approaches (Nikkanen et al., 2020; Santos-Merino et al., 2021). Understanding the molecular mechanisms enabling cells to coordinate photon flux with electron flux is essential for optimizing cyanobacterial-based solar fuel conversion systems.
Data availability statement
The original contributions presented in the study are included in the article/Supplementary Material. Further inquiries can be directed to the corresponding author.
Author contributions
ZG: Conceptualization, Data curation, Formal analysis, Investigation, Methodology, Writing – original draft, Writing – review & editing. SS: Data curation, Formal analysis, Investigation, Writing – original draft, Writing – review & editing. PK: Conceptualization, Funding acquisition, Project administration, Supervision, Writing – original draft, Writing – review & editing. CL: Conceptualization, Funding acquisition, Investigation, Methodology, Resources, Supervision, Writing – original draft, Writing – review & editing.
Funding
The author(s) declare financial support was received for the research, authorship, and/or publication of this article. This work was authored by the National Renewable Laboratory, operated by Alliance for Sustainable Energy, LLC, for the U.S. Department of Energy (DOE) under Contract No. DE-AC36-08GO28308. Funding provided by the U.S. Department of Energy Office of Basic Energy Sciences, Division of Chemical Sciences, Geosciences, and Biosciences, Photosynthetic Systems Program. The views expressed in the article do not necessarily represent the views of the DOE or the U.S. Government. The U.S. Government retains and the publisher, by accepting the article for publication, acknowledges that the U.S. Government retains a nonexclusive, paid-up, irrevocable, worldwide license to publish or reproduce the published form of this work, or allow others to do so, for U.S. Government purposes.
Acknowledgments
The authors acknowledge the contribution of Nick Sweeney for the use of custom NREL bioreactors, U.S. patent application no. 16/998,045, for the growth of strains. The authors acknowledge Melissa Cano for contributing to the generation of ORR deficient strains.
Conflict of interest
The authors declare that this study received funding from the U.S. Department of Energy, Basic Energy Sciences, Chemical Sciences, Geosciences and Biosciences Division, Photosynthetic Systems Program. The funder was not involved in the study or the decision to submit it for publication.
Publisher’s note
All claims expressed in this article are solely those of the authors and do not necessarily represent those of their affiliated organizations, or those of the publisher, the editors and the reviewers. Any product that may be evaluated in this article, or claim that may be made by its manufacturer, is not guaranteed or endorsed by the publisher.
Supplementary material
The Supplementary Material for this article can be found online at: https://www.frontiersin.org/articles/10.3389/fphgy.2024.1467480/full#supplementary-material
References
Akhtar P., Biswas A., Balog-Vig F., Domonkos I., Kovács L., Lambrev P. H. (2022). Trimeric photosystem I facilitates energy transfer from phycobilisomes in Synechocystis sp. PCC 6803. Plant Physiol. 189, 827–838. doi: 10.1093/plphys/kiac130
Allahverdiyeva Y., Mustila H., Ermakova M., Bersanini L., Richaud P., Ajlani G., et al. (2013). Flavodiiron proteins Flv1 and Flv3 enable cyanobacterial growth and photosynthesis under fluctuating light. Proc. Natl. Acad. Sci. 110, 4111–4116. doi: 10.1073/pnas.1221194110
Allen J. F. (2002). Photosynthesis of ATP—electrons, proton pumps, rotors, and poise. Cell 110, 273–276. doi: 10.1016/S0092-8674(02)00870-X
Allen M. M. (1968). Simple conditions for growth of unicellular blue-green algae on plates. J. Phycol. 4, 1–4. doi: 10.1111/j.1529-8817.1968.tb04667.x
Arnon D. I. (1959). Conversion of light into chemical energy in photosynthesis. Nature 184, 10–21. doi: 10.1038/184010a0
Brown K. A., Guo Z., Tokmina-Lukaszewska M., Scott L. W., Lubner C. E., Smolinski S., King P., et al. (2020). Correction: the oxygen reduction reaction catalyzed by Synechocystis sp. PCC 6803 flavodiiron proteins. Sustain. Energy Fuels 4, 417–417. doi: 10.1039/C9SE90057H
Bryant D. A., Canniffe D. P. (2018). How nature designs light-harvesting antenna systems: design principles and functional realization in chlorophototrophic prokaryotes. J. Phys. B: Atomic Mol. Optical Phys. 51. doi: 10.1088/1361-6455/aa9c3c
Calzadilla P. I., Kirilovsky D. (2020). Revisiting cyanobacterial state transitions. Photochemical Photobiological Sci. 19, 585–603. doi: 10.1039/c9pp00451c
Chitnis P. R., Xu Q., Chitnis V. P., Nechushtai R. (1995). Function and organization of Photosystem I polypeptides. Photosynthesis Res. 44, 23–40. doi: 10.1007/BF00018294
Demmig-Adams B., Adams III W. (1992). Photoprotection and other responses of plants to high light stress. Annu. Rev. Plant Biol. 43, 599–626. doi: 10.1146/annurev.pp.43.060192.003123
Hartmann V., Harris D., Bobrowski T., Ruff A., Frank A., Günther Pomorski T., et al. (2020). Improved quantum efficiency in an engineered light harvesting/photosystem II super-complex for high current density biophotoanodes. J. Materials Chem. A 8, 14463–14471. doi: 10.1039/D0TA03444D
Helman Y., Tchernov D., Reinhold L., Shibata M., Ogawa T., Schwarz R., et al. (2003). Genes encoding A-type flavoproteins are essential for photoreduction of O2 in cyanobacteria. Curr. Biol. 13, 230–235. doi: 10.1016/S0960-9822(03)00046-0
Hirose Y., Chihong S., Watanabe M., Yonekawa C., Murata K., Ikeuchi M., et al. (2019). Diverse chromatic acclimation processes regulating phycoerythrocyanin and rod-Shaped phycobilisome in cyanobacteria. Mol. Plant 12, 715–725. doi: 10.1016/j.molp.2019.02.010
Kaneko T., Sato S., Kotani H., Tanaka A., Asamizu E., Nakamura Y., et al. (1996). Sequence analysis of the genome of the unicellular cyanobacterium synechocystis sp. Strain PCC6803. II. Sequence determination of the entire genome and assignment of potential protein-coding regions. DNA Res. 3, 109–136. doi: 10.1093/dnares/3.3.109
Kondo K., Geng X. X., Katayama M., Ikeuchi M. (2005). Distinct roles of CpcG1 and CpcG2 in phycobilisome assembly in the cyanobacterium Synechocystis sp. PCC 6803. Photosynthesis Res. 84, 269–273. doi: 10.1007/s11120-004-7762-9
Kondo K., Ochiai Y., Katayama M., Ikeuchi M. (2007). The membrane-Associated cpcG2-Phycobilisome in synechocystis: A new photosystem I antenna. Plant Physiol. 144, 1200–1210. doi: 10.1104/pp.107.099267
Kopečná J., Komenda J., Bučinská L., Sobotka R. (2012). Long-Term acclimation of the cyanobacterium synechocystis sp. PCC 6803 to high light is accompanied by an enhanced production of chlorophyll that is preferentially channeled to trimeric photosystem I. Plant Physiol. 160, 2239–2250. doi: 10.1104/pp.112.207274
Li N., Warren P. V., Golbeck J. H., Frank G., Zuber H., Bryant D. A. (1991). Polypeptide composition of the Photosystem I complex and the Photosystem I core protein from Synechococcus sp. PCC 6301. Biochim. Biophys. Acta (BBA) - Bioenergetics 1059, 215–225. doi: 10.1016/S0005-2728(05)80206-3
Liu H. (2023). Cyanobacterial phycobilisome allostery as revealed by quantitative mass spectrometry. Biochemistry 62, 1307–1320. doi: 10.1021/acs.biochem.3c00047
Liu H., Weisz D. A., Zhang M. M., Cheng M., Zhang B., Zhang H., et al. (2019). Phycobilisomes harbor FNRL in cyanobacteria. mBio 10:e00669-19. doi: 10.1128/mbio.00669-00619
Montgomery B. L. (2017). Seeing new light: recent insights into the occurrence and regulation of chromatic acclimation in cyanobacteria. Curr. Opin. Plant Biol. 37, 18–23. doi: 10.1016/j.pbi.2017.03.009
Mullineaux C. W. (2008). Phycobilisome-reaction centre interaction in cyanobacteria. Photosynthesis Res. 95, 175–182. doi: 10.1007/s11120-007-9249-y
Munekage Y., Hashimoto M., Miyake C., Tomizawa K.-I., Endo T., Tasaka M., et al. (2004). Cyclic electron flow around photosystem I is essential for photosynthesis. Nature 429, 579–582. doi: 10.1038/nature02598
Niedzwiedzki D. M., Liu H., Blankenship R. E. (2019). Excitation energy transfer in intact cpcL-Phycobilisomes from synechocystis sp. PCC 6803. J. Phys. Chem. B 123, 4695–4704. doi: 10.1021/acs.jpcb.9b02696
Nikkanen L., Santana Sánchez A., Ermakova M., Rögner M., Cournac L., Allahverdiyeva Y. (2020). Functional redundancy between flavodiiron proteins and NDH-1 in Synechocystis sp. PCC 6803. Plant J. 103, 1460–1476. doi: 10.1111/tpj.14812
Santos-Merino M., Torrado A., Davis G. A., Röttig A., Bibby T. S., Kramer D. M., et al. (2021). Improved photosynthetic capacity and photosystem I oxidation via heterologous metabolism engineering in cyanobacteria. Proc. Natl. Acad. Sci. 118, e202152311. doi: 10.1073/pnas.2021523118
Shikanai T., Yamamoto H. (2017). Contribution of cyclic and pseudo-cyclic electron transport to the formation of proton motive force in chloroplasts. Mol. Plant 10, 20–29. doi: 10.1016/j.molp.2016.08.004
Smolinski S. L., Lubner C. E., Guo Z., Artz J. H., Brown K. A., Mulder D. W., et al. (2022). The influence of electron utilization pathways on photosystem I photochemistry in Synechocystis sp. PCC 6803. RSC Adv. 12, 14655–14664. doi: 10.1039/D2RA01295B
Takahashi H., Clowez S., Wollman F.-A., Vallon O., Rappaport F. (2013). Cyclic electron flow is redox-controlled but independent of state transition. Nat. Commun. 4, 1954. doi: 10.1038/ncomms2954
Vicente J. B., Gomes C. M., Wasserfallen A., Teixeira M. (2002). Module fusion in an A-type flavoprotein from the cyanobacterium Synechocystis condenses a multiple-component pathway in a single polypeptide chain. Biochem. Biophys. Res. Commun. 294, 82–87. doi: 10.1016/S0006-291X(02)00434-5
Walters R. G. (2005). Towards an understanding of photosynthetic acclimation. J. Exp. Bot. 56, 435–447. doi: 10.1093/jxb/eri060
Watanabe M., Semchonok D. A., Webber-Birungi M. T., Ehira S., Kondo K., Narikawa R., et al. (2014). Attachment of phycobilisomes in an antenna–photosystem I supercomplex of cyanobacteria. Proc. Natl. Acad. Sci. 111, 2512–2517. doi: 10.1073/pnas.1320599111
Wilson A., Boulay C., Wilde A., Kerfeld C. A., Kirilovsky D. (2007). Light-Induced energy dissipation in iron-Starved cyanobacteria: roles of OCP and isiA proteins. Plant Cell 19, 656–672. doi: 10.1105/tpc.106.045351
Keywords: photosynthesis, light harvesting, phycobilisome (PBS), photosystem I (PSI), excitation energy transfer (EET)
Citation: Guo Z, Smolinski SL, King PW and Lubner CE (2024) Alteration of phycobilisome excitation energy transfer properties in response to attenuations in peripheral electron flow. Front. Plant Physiol. 2:1467480. doi: 10.3389/fphgy.2024.1467480
Received: 19 July 2024; Accepted: 31 October 2024;
Published: 27 November 2024.
Edited by:
Wu Xu, University of Louisiana at Lafayette, United StatesReviewed by:
Qingfang He, University of Arkansas at Little Rock, United StatesPetar H. Lambrev, HUN-REN Biological Research Centre, Hungary
Copyright © 2024 Guo, Smolinski, King and Lubner. This is an open-access article distributed under the terms of the Creative Commons Attribution License (CC BY). The use, distribution or reproduction in other forums is permitted, provided the original author(s) and the copyright owner(s) are credited and that the original publication in this journal is cited, in accordance with accepted academic practice. No use, distribution or reproduction is permitted which does not comply with these terms.
*Correspondence: Carolyn E. Lubner, Y2FyYS5sdWJuZXJAbnJlbC5nb3Y=
†Present address: Zhanjun Guo, School of Materials Science & Engineering, Beijing Institute of Technology, Beijing, China