- 1Umea Plant Science Centre (UPSC), Department of Forest Genetics and Plant Physiology, Swedish University of Agricultural Sciences, Umea, Sweden
- 2Umea Plant Science Centre (UPSC), Department of Plant Physiology, Umea University, Umea, Sweden
Cell-cell adhesion is a fundamental aspect of maintaining multicellular integrity while ensuring controlled cell and organ shedding, intercellular space formation and intrusive growth. Understanding of the precise mechanisms governing regulated cell separation, such as abscission, considerably progressed in recent decades. However, our comprehension of how plants maintain adhesion within tissues in which it is essential remains limited. Here we review some of the well-established knowledge along with latest discoveries that lead us to rethink the way developmentally controlled cell separation and adhesion maintenance may work. We also specifically explore the relationship between growth and adhesion, highlighting their similarities and coupling, and propose a plausible framework in which growth and adhesion are tightly co-regulated.
Introduction
Plants are multicellular organisms that exhibit clonal multicellularity and develop from a single cell through the process of cell division. Contrary to animal, plant cells are surrounded by a cell wall which mediates their adhesion (Atakhani et al., 2022). Adhesion between cells is established during cell division when a new shared wall is formed by the cell plate (Jarvis et al., 2003). Throughout normal growth and development, cell differentiation gives rise to a complex tissue organization roughly made up of the epidermis, ground tissues, vasculature, and meristems. Interestingly, different tissues appear to develop differential adhesion. While the epidermis keeps a strong adhesion to form a barrier to the environment (Galletti et al., 2016), stomata formed within it through guard cells separation allow a controlled gas exchange between the inside and outside of the leaf (Lin et al., 2022). Below the epidermis, the mesophyll and cortex parenchyma (types of ground tissue) tend to develop large intercellular spaces forming largely by cell separation at the tricellular junctions (Whitewoods, 2021). This creates a large network of air spaces in the tissues that is important for gas exchange and photosynthesis (Figure 1F). Further within the tissues, the vasculature tends to develop rigid and thickened cell walls with tight adhesion to ensure the proper transport of water and nutrients (Kuriyama and Fukuda, 2007; Lucas et al., 2013). Later on, a number of cells and organs can shed through cell separation. For instance, leaves as well as floral organs such as sepal, petal, stamen and carpels undergo abscission after pollination and seed maturation (Figure 1A; Leslie et al., 2007). The seeds can shed, and the pollen grains separate to be disseminated individually (Figure 1D; Wen et al., 2007). In the root system, root cap sloughing and border cells separation from the root tip help to protect the root and promote soil penetration (Figure 1C; Driouich et al., 2007; Wen et al., 2007). In parallel, lateral roots emerge through the cortex and epidermis thanks to controlled cell separation (Figure 1B; Banda et al., 2019). Some specific cell types such as the pollen tube and fiber cells can grow intrusively by their tip, leading to cell-cell contact rearrangement (Figure 1E; Mollet et al., 2007; Marsollier and Ingram, 2018). Finally, cells can re-adhere through grafting or post embryonic tissue fusion (Melnyk, 2017). This brief overview of cell adhesion and separation dynamics in plants highlights how important the regulation of cell adhesion is for plant growth and development (Figure 1).
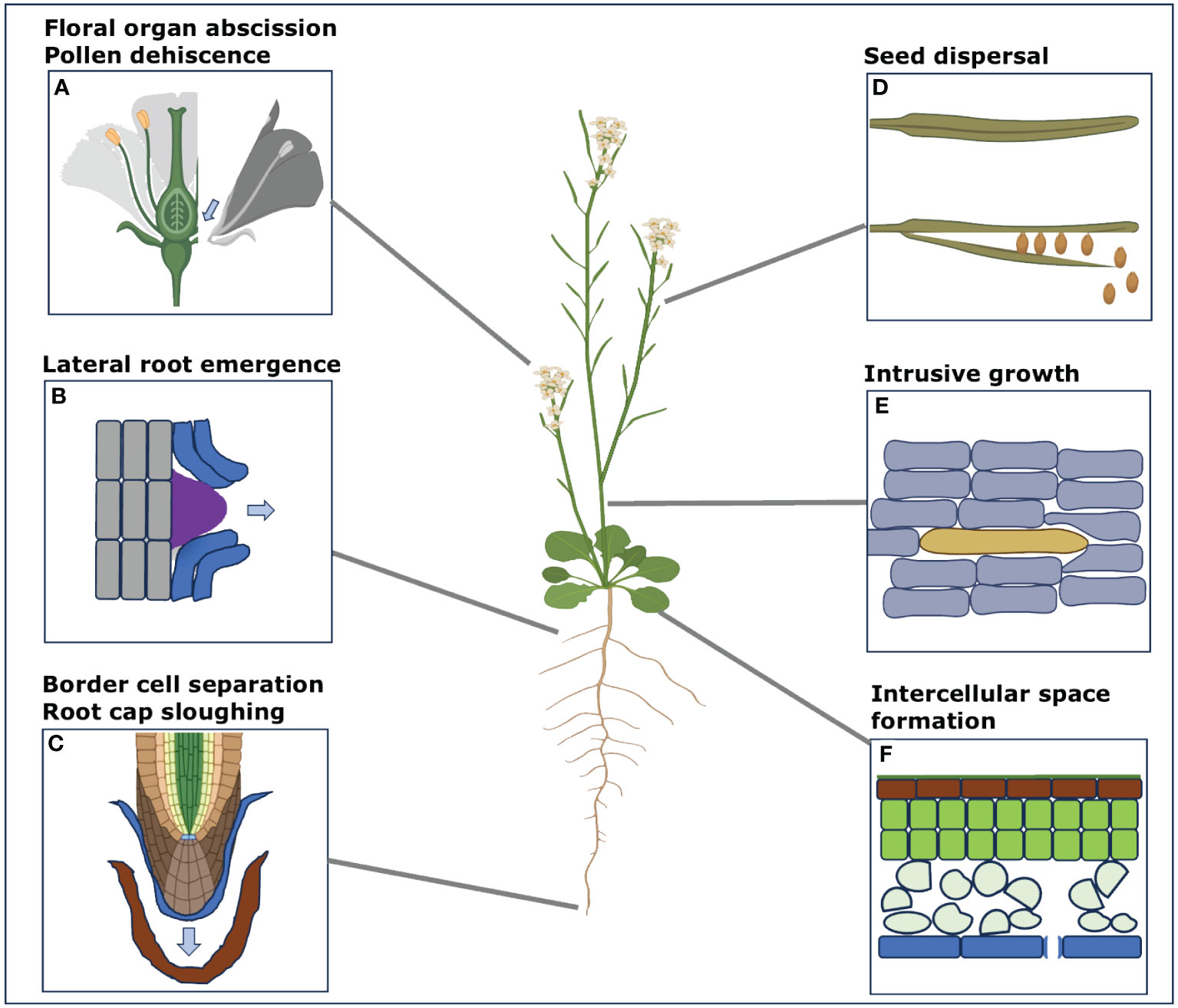
Figure 1 Different examples of the processes involving cell separation in the model plant Arabidopsis. Here, we display some of the main processes that involve cell separation during various developmental stages and during specific periodic changes in Arabidopsis, used as an example here. These processes are not just limited to this model species only but can be involved in other plant species too. These different instances of cell separation processes include (A) Floral organ abscission of sepals, petals, stamen, carpels including pollen separation (B) Lateral root emergence through the cell layers of cortex and epidermis (C) Root cap sloughing and border cell separation from the root tip (D) Seed dispersal after the maturation from the siliques (E) Intrusive growth of cambial cells in inflorescence stems (F) Intercellular space formation in the mesophyll and cortex parenchyma. (Some segments from this figure were generated with the help of Biorender (www.bioRender.com accessed on 14 December 2023).
Cell adhesion is mediated by the cell wall
The attachment of neighboring cells in plants is mediated through the formation of the cell wall. Approximately 90% of the total components in the cell wall are made up of polysaccharides, namely cellulose, hemicellulose, and pectin, along with structural proteins and cell wall remodeling enzymes making up the remaining 10% (Anderson and Kieber, 2020). In young expanding tissues the cell wall between two adjacent cells generally displays a layered structure: at the center the middle lamella is enriched in pectins, and on each side sit the primary cell walls of each adjacent cells. This structure is believed to be established during and following cell division (Jarvis et al., 2003). First, when the formation of the cell plate establishes the precursor of the middle lamella, and after the completion of the new cell wall when each daughter cells initiate the synthesis of their primary cell wall (Zamil and Geitmann, 2017). These newly formed walls thus sandwich the pre-existing pectin-enriched matrix derived from the cell plate that forms the middle lamella. Note that the exact genesis/origin, structure and composition of the middle lamella remains poorly understood (Zamil and Geitmann, 2017; Malek and Mouille, 2023). Yet, overall, it is generally recognized that pectins and the middle lamella play a central role as components of the cell wall for mediating cell adhesion (for extensive reviews on the topic see Knox, 1992; Jarvis et al., 2003; Daher and Braybrook, 2015; Atakhani et al., 2022). The middle lamella can then be specifically degraded through the action of pectin remodeling and degrading enzymes (e.g. Pectin Methyl Esterases (PMEs), PolyGalactoronases (PGs) and Pectate Lyases (PLs)) for cell separation (Daher and Braybrook, 2015). Interestingly, the pectin in the middle lamella is often depicted as the glue that makes the cells stick together (Zamil and Geitmann, 2017). However, except in specific cases (e.g. grafting) plant cells are not adhering to each other by being put in contact to stick but rather remain attached after cell division. In turn, it could be argued that instead of being a “gluing layer”, the middle lamella may primarily serve as a “degradable” layer for cell separation, that can specifically be degraded by a subset of enzymes (pectin degrading), without degrading the whole cell wall which would otherwise threaten cell integrity.
The primary cell wall also serves a central role in cell and tissue growth. It is a dynamic structure that is constantly remodeled during the processes of growth and development (Anderson and Kieber, 2020; Cosgrove, 2023). This is mediated by the constant synthesis and secretion of cell wall polysaccharides and proteins. This contributes to adding material to the wall while it is being expanded and to remodel it to allow changes of extensibility (Anderson and Kieber, 2020). Along with turgor pressure, the action of cell wall remodeling enzymes has been shown to be the key downstream mediator of cell expansion, overall controlling tissue growth and morphogenesis (Cosgrove, 2022; Coen and Cosgrove, 2023). Those enzymes typically include members from the XYLOGLUCAN ENDOTRANSGLUCOSYLASE/HYDROLASE (XTH), EXPANSIN (EXP), CELLULASE, PECTIN METHYLESTERASE (PME) and POLYGALATURONASE (PG) families (For a comprehensive review on the subject see Cosgrove, 2016). For growth to be properly regulated, the action of these enzymes should not lead to the overall degradation and excessive weakening of the cell wall, which could lead to cell bursting due to turgor pressure (Feng et al., 2018; Sapala et al., 2018; Malivert et al., 2021). Instead, the activity of these enzymes and overall cell wall remodeling needs to be tightly regulated by cell wall integrity signaling feedback mechanisms (Bacete and Hamann, 2020). Interestingly there are striking similarities in the set of cell wall remodeling enzymes that are involved in growth and cell separation (See sections below). This could imply that similar cell wall integrity mechanisms could be in place not only for keeping cellular integrity (preventing cell bursting) but also keeping supracellular integrity (cell adhesion) (Verger et al., 2018) during extensive growth.
Developmentally controlled cell separation in plants
As described in the introductory section, there are several events throughout plant life during which cell separation is specifically triggered (Figure 1). The process of abscission has been particularly studied for its agricultural implications. Here we provide a broad description and the common mechanisms regulating these types of developmentally regulated cell separation, but for more detailed review see (Ballester and Ferrándiz, 2017; Patharkar and Walker, 2018). Broadly, abscission processes can be categorized into four stages: 1) Initially, abscission in plants involves the formation of a defined Abscission Zone (AZ) with the specification of specialized cell layers mediated by developmental patterning transcription factors (Mao et al., 2000; Cho et al., 2008; Gubert et al., 2014). 2) The second step consists in triggering the signaling cascade that will lead to cell separation in the abscission zone. A precise balance between ethylene and auxin has been shown to be key in triggering abscission and may serve to integrate environmental stimuli to regulate the timing of abscission (Taylor and Whitelaw, 2001; Butenko et al., 2006; Meir et al., 2010). Moreover, the non-hormonal control of abscission in plants involves the short secretory peptide known as INFLORESCENCE DEFICIENT IN ABSCISSION (IDA) (Butenko et al., 2003) and its downstream receptor-like kinases (RLKs), HAESA (HAE) and HAESA-LIKE 2 (HSL2) (Cho et al., 2008; Estornell et al., 2015). Downstream, this peptide-receptor complex activates a mitogen-activated protein kinase (MAPK) cascade, which primarily consists of MAPK kinase 4 (MKK4)/MKK5 and MAPK 3 (MPK3)/MPK6 (Cho et al., 2008), and may ultimately de-repress the transcription factors KNOTTED-LIKE FROM ARABIDOPSIS THALIANA2 (KNAT2) and KNAT6 (Shi et al., 2011). 3) This signaling cascade then leads to the massive expression of cell wall remodeling enzymes. While the pectin remodeling enzyme of the PME and PG families have been shown to be central players for cell separation, it was also shown that a wider set of CWR enzyme is expressed during abscission. Those enzymes typically include members from the XTH, EXPANSIN, CELLULASE, PME and PG families (Cho and Cosgrove, 2000; Rhee et al., 2003; Francis et al., 2006; Cai and Lashbrook, 2008; Ogawa et al., 2009). Interestingly, the combined action of these enzymes, along with the presence of turgor pressure in the cells also lead to local cell expansion (Cai and Lashbrook, 2008) and overall generate forces that physically help pull cells apart to achieve abscission (Reiche, 1885; Taesakul et al., 2015). Finally, 4) after the abscission of the organ, a protective epidermal layer is formed at the abscised region in the plant (Shi et al., 2019).
Interestingly, some of the cell separation processes taking place in the root, namely lateral root emergence and root cap sloughing have also been shown to involve part of the IDA signaling cascade without apparent abscission zone specification. For lateral root emergence, auxin signaling was shown to induce the expression of the IDA peptide around the lateral root emergence site, which along with the HEA and HSL2 receptors, help the lateral root primordia to emerge from different cell layers (Kumpf et al., 2013). The IDL1 (IDA LIKE1) homologs of IDA and its receptor HSL2 have been shown to regulate root cap sloughing by controlling the frequency of sloughing events (Shi et al., 2018). Interestingly however, the progressive detachment of the border-like cells along the elongating root tip seems to happen independently from the sloughing events. Overall, this suggests that the IDA/HEA signaling module may be widely employed to specifically trigger cell separation events in plants. Note however, that there are significant differences in the way in which root cap cells detach between different species. Arabidopsis and more generally Brassicaceae represent a specific case for which border cells do not fully detach (Border-like cells; (Driouich et al., 2007; Mravec et al., 2014)) while in other species, true border cells are continuously released as individual cells (Mravec, 2017; Mravec et al., 2017) and thus may not require active root cap sloughing-off events.
Active rearrangement of cell adhesion by intrusive growth
By far most cells in plant tissues establish adhesion during cell division and grow in a diffuse symplastic manner, keeping the same neighbors throughout their life span. However, some specific cell types harbor an intrusive tip growth behavior leading to the rearrangement of cell-cell contacts. The two most studied examples of intrusive tip growth in plants are the pollen tubes (Kamel and Geitmann, 2023) and the fiber cells (Lev-Yadun, 2001; Gorshkova et al., 2012). During intrusive tip growth, cells generally elongate specifically at their tip by a combination of the addition of cell wall material localized at the tip and internal hydrostatic (turgor) pressure (Sanati Nezhad and Geitmann, 2013). Different pectin modifying enzymes are believed to be implicated in the modification of the cell wall during pollen tube growth (Bosch and Hepler, 2005; Kamel and Geitmann, 2023). Recently it was shown that pectate lyase-like (PLL) may contribute to the lubrication and loosening of the middle lamella in the intruded tissues (Chebli and Geitmann, 2023). In wood fiber cells, several studies have revealed the contribution of cell wall remodeling enzymes. Those include the XTH, EXPANSIN, PME and PL families (Im et al., 2000; Israelsson et al., 2003; Gray-Mitsumune et al., 2004; Gray-Mitsumune et al., 2008; Siedlecka et al., 2008; Kushwah et al., 2020). It is generally unclear if those enzymes primarily play a role in the cell wall remodeling at the tip for tip growth or specifically contribute to loosening the middle lamella of the intruded tissues, but it is worth considering that some of these enzymes may serve both purposes at the same time. In addition to cell wall remodeling, physical factors also play a major role. It has been shown that the turgor pressure in the growing tip of pollen tubes applies considerable forces that help penetrating between the cells (Sanati Nezhad et al., 2013). It was also proposed that high turgor pressure and radial expansion of the cells making up the tissues in which cells intrude may contribute to promote intercellular spaces formation. The intruding tips could then grow more easily within such intercellular spaces (Yanagisawa et al., 2017; Majda et al., 2021).
Intercellular spaces formation
Plant internal tissues can develop air spaces which are notably important for gas exchange and photosynthesis (Earles et al., 2018; Whitewoods, 2021). In most cases they initially form by cell separation at the tricellular junctions. Air spaces can further expand by continued cell separation (schizogeny), through the expansion of the already separated wall regions (expansigeny) or a combination of both (Zhang et al., 2021), which leads to an increase in airspace size (Whitewoods, 2021). Interestingly this process is tightly associated with the intense expansion of the tissues in which it takes place such as during leaf and stem expansion. In this case it is still unknown which cell wall remodeling enzymes are involved in the initial cell separation process. However, the high turgor pressure in those ground tissues that is required to drive tissue growth (Kutschera and Niklas, 2007) should play a key role. At tricellular junction, cell edges often display sharp angles. Because the cortical (cell wall) tension derived from turgor pressure would tend to round up those edges, in fully adhering cells, those tricellular junction are mechanical stress hotspots that contribute to separate the cells if adhesion is weakened (Jarvis, 1998; Treado et al., 2022). In turn, it is possible that growth-promoting cell wall remodeling enzymes activity in combination with high separation forces at those mechanical hotspots would be sufficient to weaken the middle lamella to a point that would allow cell separation at tricellular junctions. Interestingly, in this case cell separation would be a passive process, collateral to the process of growth.
Cell adhesion maintenance: the case of cell adhesion defective mutants
As described above, while many cells in ground tissues develop intercellular spaces through partial cell separation, other surrounding tissues like the epidermis and vasculature need to maintain tight adhesion. However, the mechanisms regulating these processes are still very unclear. Within the last three decades, genetic screens have led to the isolation of cell adhesion defective mutants which are thus unable to properly maintain adhesion and offer some clues to start investigating this process. Such mutants could either be unable to produce the cell wall polysaccharides that keep cells attached to each other or be involved in sensing and feedback mechanisms ensuring the maintenance of adhesion. One of the most characteristic examples are the quasimodo1 (qua1) and qua2 mutants (Bouton et al., 2002; Krupková et al., 2007; Mouille et al., 2007), for which the disrupted loci encode a putative galacturonosyltransferase and a pectin methyltransferase (Du et al., 2020), respectively. These mutants are deficient in HomoGalacturonans synthesis (HG), the main form of pectin in the cell wall and middle lamella. This led to the conclusion that the adhesion phenotype of these mutants was a direct consequence of decreased pectin content in the cell wall, likely disrupting the middle lamella. However, a genetic suppressor screen carried out on both qua1 and qua2 mutants identified alleles of ESMERALDA1 (ESMD1), a putative o-fucosyltransferase (Verger et al., 2016). The suppressor lines display a rescued adhesion phenotype without restoring the HG fraction that is deficient in the quasimodo mutants. ESMD1 was hypothesized to play a role in cell wall integrity sensing, via the pectin-binding receptor WALL-ASSOCIATED KINASES (WAKs) by potentially mediating the o-fucosylation of the epidermal growth factor (EGF) domains of the WAKs (Verger et al., 2016). Another putative o-fucosyltransferase mutant, friable1 (frb1) was shown to have cell adhesion defects without significant pectin levels deficiency except for a perturbed HG methylesterification level (Neumetzler et al., 2012). Altogether this suggests that bulk pectin content in the cell wall may not be the primary determinant of cell adhesion maintenance, but that more complex regulations of the pectin and overall cell wall structure are at play. However, it was later shown that enzymes of the putative o-fucosyltransferase family, including FRB1 are instead acting as Rhamnose RamnosylTransferases (Takenaka et al., 2018; Wachananawat et al., 2020) and thus involved in the synthesis of RGI pectins rather than signaling. It was also shown that WAKs are likely not o-fucosylated on their EGF domains, that esmd1 does not seem to impact the function of the WAKs, and that knocking out WAKs did not rescue adhesion defects in the qua2 mutant (Kohorn et al., 2021). Nevertheless, further work confirmed that the QUA2 knockout does lead to massive secondary responses which can largely account for its phenotypes and that most are absent in the qua2 esmd1 suppressor line. These includes over expression of PGs, miss regulation of PMEs as well as deficiency in cellulose synthesis and a disrupted cuticle (Du et al., 2020; Lorrai et al., 2021; Barnes et al., 2022; Grandjean et al., 2023). It was also recently suggested that endogenous oligogalacturonides (small pectin fragments derived from the degradation of HG) may play a signaling role in this context (Grandjean et al., 2023). Furthermore, an esmd1 mutant allele was also recently isolated as a suppressor of the cellulose deficient mutant korrigan1 (kor1). In this case it appears to act by suppressing elevated jasmonate levels in the kor1 mutant (Mielke et al., 2021). While it is still unclear what the function of ESMD1 is, these observations suggest that ESMD1 may still play a role directly or indirectly in cell wall integrity signaling which ultimately influences cell adhesion maintenance.
It is also interesting to note that the quasimodo adhesion phenotype can be partially rescued by decreasing the overall growth or the tissue tensions derived from differential growth between the inner tissues and the epidermis (Verger et al., 2018) and that it can be enhanced by increasing the growth differential between the inner tissues and epidermis (Kelly-Bellow et al., 2023). This shows that in quasimodo, in addition to a reduced adhesion mediated by extensive cell wall remodeling, the adhesion phenotype depends on the overall extent of growth and tension pulling cells apart in the epidermis.
In addition to QUA1, QUA2, ESMD1, and FRB1, two new gene families have recently been identified to act in the same pathway. Mutation in the genes coding for Golgi membrane localized protein ELMO1, ELMO2 and ELMO4/NKS1 (Kohorn et al., 2021; Lathe et al., 2021) as well as Golgi-localized putative S-adenosyl methionine transporters (GoSAMT1 and GoSAMT2) (Temple et al., 2022) also lead to a strong defect in cell-cell adhesion which can be rescued by esmd1. ELMO family proteins are suspected to act as scaffold for QUA1 and QUA2 in the Golgi (Lathe et al., 2021; Kohorn et al., 2023) and GoSAMT transporter should be necessary to provide the S-adenosyl methionine for HG methyl esterification by QUA2 in the Golgi.
Beyond direct cell wall regulators, other mutants with clear cell adhesion defects include mutants defective in actin filament nucleators from the ACTIN-RELATED PROTEINS 2 and 3 (ARP2/3) complex (Le et al., 2003; Mathur et al., 2003) as well as upstream regulators SCAR/WAVE (WASP family Verprolin homolog — also known as SCAR for suppressor of cAMP receptor) complex (Basu et al., 2004; El-Assal et al., 2004) and SPIKE1 (Qiu et al., 2002). While these mutants do not appear to have clear cell wall defects in growing tissues (Dyachok et al., 2008; Pratap Sahi et al., 2017), they suggest a more localized role of actin-mediated cell wall polysaccharides secretion at the subcellular level for the proper maintenance of cell adhesion. Furthermore, the putative mechanosensitive calcium channel DEFECTIVE KERNEL1 (DEK1; (Galletti et al., 2015; Amanda et al., 2016) and epidermal identity transcription factors MERISTEM LAYER1 (ATML1) and PROTODERMAL FACTOR2 (PDF2) mutants (Abe et al., 2003) show epidermis specific adhesion defects. While DEK1 phenotype suggests a role for mechanical signals feedback in regulating cell adhesion maintenance, it was also shown to contribute to epidermal cell identity (Johnson et al., 2005) and epidermal identity was shown to be regulated by mechanical signals (Iida et al., 2023). In dek1 and pdf2 atml1 mutants, cells that should belong to the epidermal cell layer, instead resemble mesophyll cell. This suggests that in these mutants the outer layer of cell has either lost the capacity to specifically maintain cell adhesion as the epidermis normally does, or that it activates a putative signaling pathway leading to intercellular space formation. Overall, while they remain to be better characterized for their cell adhesion phenotype, these mutants further hint at a complex regulation of cell adhesion maintenance in plants through cytoskeleton dynamics, cell wall integrity, mechanosensing and cell identity specification.
Growth and adhesion
Based on the above review of the field it appears that there are likely at least two separate mechanisms regulating cell adhesion in plants. One specifically to promote cell separation and one specifically to maintain cell adhesion. It is well established that for active cell separation events such as in the case of abscission, upstream control by hormones and peptides lead to the specific and active digestion of the cell wall (see section Developmentally controlled cell separation in plants). One could argue that in this context the extensive cell wall remodeling that is taking place is aimed at cell separation but with the collateral action of promoting local expansion that in turn helps to pull cells apart. This tends to support the idea that adhesion between cells is a passive default state initially established during cell division, and that an active mechanism needs to be triggered to induce separation. However, it is important to note that these events take place in tissues which are not actively growing anymore. It is thus necessary for the plant to specifically re-activate cell wall remodeling that can lead to the digestion of the middle lamella, but also differential growth between different parts of the abscission zone to help physically pull the cells apart. In other words, cell separation needs a combination of cell wall remodeling, growth, and tensions to take place. On the other hand, similar conditions are already present by default in actively growing tissues. The process of growth is driven by turgor pressure which, along with cell wall remodeling, generates cell expansion (Cosgrove, 2016). It is also well established that the layered tissue organization leads to tissue tensions: high tension in the growth restricting epidermis, and relative compression in the inner tissues (Kutschera and Niklas, 2007). Work on cell adhesion defective mutants has further revealed that cell separation in those mutants is largely correlated with the extent of growth-derived tissue stresses (Verger et al., 2018; Kelly-Bellow et al., 2023). In turn, this supports the idea that in growing tissues, adhesion is not a default state. Growth associated mechanisms actively remodel the cell wall and tend to pull epidermal cells apart. Thus, plants may need to put specific mechanisms in place to prevent cell separation in cell types where adhesion is threatened and needs to be maintained. In this framework, plants would need a cell separation pathway in non-growing tissues (e.g. abscission) and a cell type specific adhesion maintenance pathway in growing tissues (Figure 2).
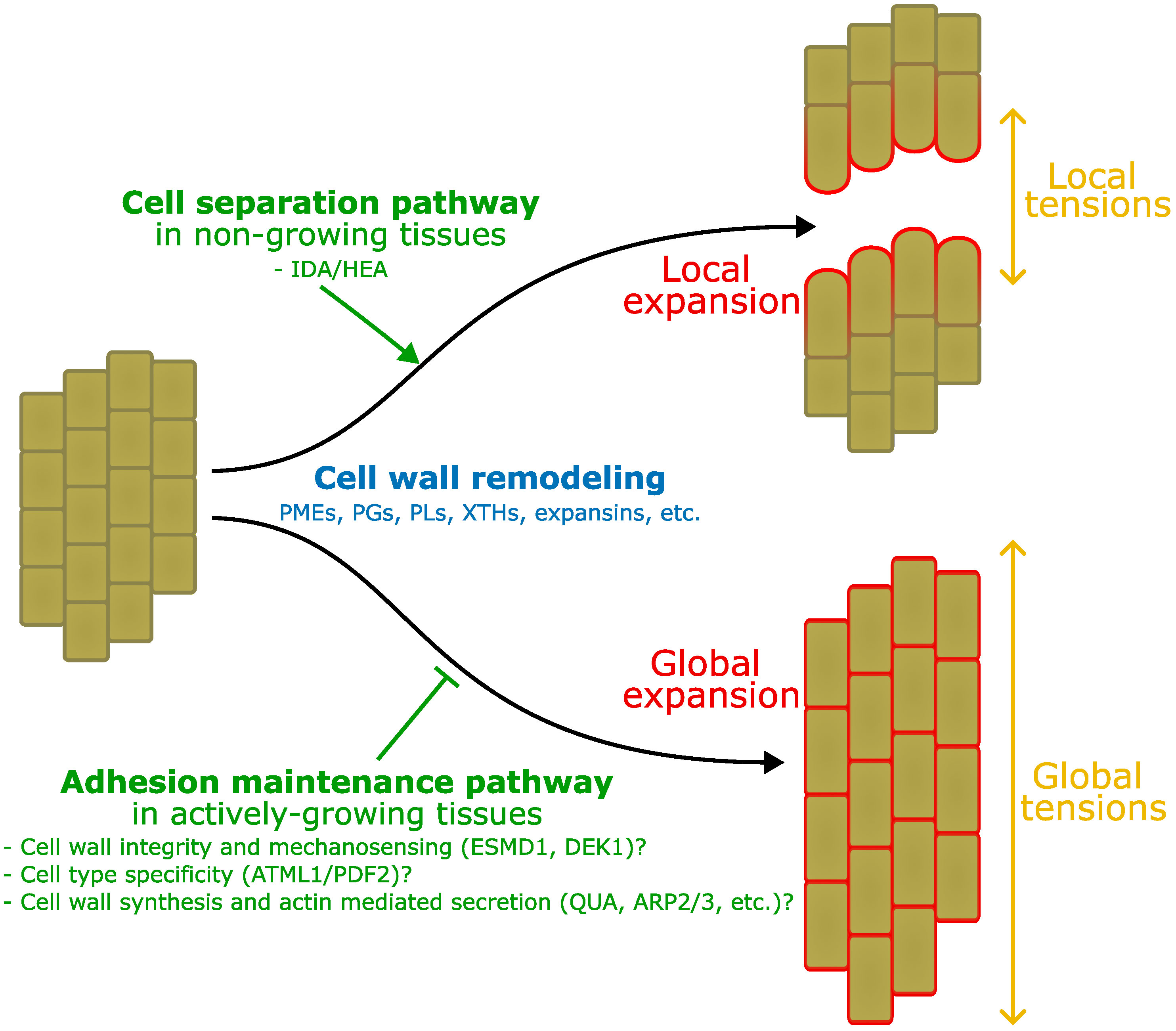
Figure 2 Interplay between adhesion, growth and tension. Here we take as an example the comparison between the process of abscission and growth in elongating tissues. In this schematic representation, both start from a group of fully adhering short cells (left). On top, IDA/HEA mediated abscission mechanisms induce local cell wall remodeling in the abscission zone (not represented), expansion and tensions, that lead to local cell separation. Below, growth induction also involves global cell wall remodeling, expansion, and tissue tensions, however, adhesion maintenance mechanisms may prevent cell separation that would otherwise takes place as suggested by mutants’ studies. Those mechanisms may involve a combination of molecular players and processes: ESMD1 and DEK1 may be involved in cell wall integrity and mechanosensing to monitor the state of the cell wall in relation to adhesion during growth. ATML1 and PDF2 epidermal identity specification may limit the activity of the adhesion maintenance pathway to the epidermis only. Wall integrity, mechanosensing and cell identity may contribute to regulate cell wall synthesis (QUA, FRB, GoSAMT, ELMO), and actin mediated secretion (ARP2/3, SCAR/WAVE, SPIKE), to ensure cell adhesion in the epidermis during growth. Similar mechanisms may for instance be at play in the vasculature, while ground tissues can develop intercellular spaces.
Revisiting the relationship between adhesion and separation in the context of growth
With this in mind we can revisit some of the cases mentioned in the sections above where adhesion is dynamically regulated including those for which we still know very little regarding their regulation mechanisms. Classical abscission and dehiscence events (leaves, floral organs, seed shedding, etc.) are already well described and take place in non-growing tissues, thus are less ambiguous in this regard. On the other hand, the case of intercellular space formation takes place in actively growing tissues and the mechanisms regulating it are still unknown. To our knowledge, none of the abscission deficient mutants are affected in intercellular space formation suggesting that it may not require such pathway. The initiation of intercellular spaces may instead be largely collateral to growth-associated cell wall remodeling and tensions at the tricellular junctions. Because adhesion remains tight in the epidermis, keeping all inner tissues cells within a sort of epidermal envelop, partially loosing adhesion between ground tissue cells doesn’t threaten the supracellular integrity of the organism. Then, the only control of cell adhesion needed would be for the maintenance in the epidermis and vasculature. While the molecular bases of such an adhesion maintenance pathway are still unclear, it could rely on cell wall integrity, mechanosensing mechanisms and be influenced by cell identity as suggested by the study of cell adhesion defective mutants (see section Cell adhesion maintenance: The case of cell adhesion defective mutants). The case of intrusive tip growth is quite interesting since it is by itself directly coupled with growth. It also takes place in tissues which are still growing and that develop intercellular spaces for the intrusive tip to grow in between (Majda et al., 2021). It is thus possible that the simple process of tip growth and associated cell wall remodeling enzyme secretion (Chebli and Geitmann, 2023) along with formation of growth associated intercellular spaces (Majda et al., 2021), is enough to explain the phenomenon of intrusive tip growth without the need for specific cell adhesion regulation mechanism. On the other hand, lateral root emergence can sometimes be considered as a case of multicellular intrusive growth. However, while the lateral root tip does grow, the emergence has to take place through the mature root cortex and epidermis which are not growing anymore. The tip of the lateral root has also been shown to be covered with a thin cuticle layer (Berhin et al., 2019). While it appears to help lubricating the tip for the root emergence, it would also prevent the secretion of cell wall remodeling enzymes by the growing root tip contrary to what has been shown for the pollen tube intrusive growth. In turn, cells of the cortex and epidermis within which the root will emerge, have to activate an abscission-like pathway that specifically triggers their separations. Finally, the case of the root cap cells is also particularly interesting. In the Arabidopsis growing root tip, the continuous detachment of the so-called border-like cells and the sloughing off of the root cap involves the separation of cells, but these appear to be two separate mechanisms at play. The border-like cells along the growing root tip appear to progressively detach as the root grows, and in parallel there is a periodic sloughing off of entire cell layers. While it has been shown that the periodic sloughing off events are regulated by a specific abscission pathway, it is likely that the progressive border-like cell detachment is a collateral effect of the root tip growth associated with the fact these cells enter a programmed cell death (Feng et al., 2018) and may not be able to actively maintain their adhesion anymore. However, as mentioned previously, border-like cells represent a particular case that appears to be specific to Brassicaceae (Driouich et al., 2007). In other species, the border cells continuously detach as individual cells, remain alive and play a key role in the root-soil interaction (Hawes et al., 2000). Border cell detachment was shown to involve extensive cell wall remodeling notably by PMEs but without noticeable pectin degradation by PGs or PLs (Mravec, 2017; Mravec et al., 2017). It was proposed that this remodeling would still contribute to loosen the middle lamella as well as drive differential growth, along with the asymmetric distribution of xyloglucans and extensins, between the inner and outer faces of border cells (respective to the root surface). This differential growth leads to the bending of individual cells which physically promotes their detachment from the root surface (Mravec, 2017; Mravec et al., 2017). Interestingly this supports the idea that growth associated cell wall remodeling and tensions can be sufficient to alter cell adhesion even in the absence of extensive pectin degradation. This also suggest that contrary to border-like cells, border cell detachment does not require active abscission-like cell separation. In turn, simply lacking a specific adhesion maintenance mechanism for the root cap cells, in the context of root tip growth, could largely explain their detachment dynamics. It was also shown that inhibiting PME activity during border cell release led to an incomplete detachment of the cells similar to what is observed with border-like cells (Mravec et al., 2017). Brassicaceae may have lost the ability to fully detach border cells and instead co-opted abscission and programmed cell death pathways to balance their root cap proliferation.
Are growth and cell separation mechanisms really similar?
Overall, here we highlight that the mechanisms of growth and cell separation appear to be highly similar in terms of cell wall remodeling and mechanics. Pushing this logic further, one could propose that abscission is only a precise targeted growth event in which adhesion maintenance feedback mechanisms are not active. There is, however, no clear evidence for that yet. Adhesion defective mutants appear to be a promising tool to study what could be the cell adhesion maintenance mechanisms. However, while here they are somehow depicted as potentially passively unable to maintain adhesion, the pectin deficient quasimodo mutant were for instance shown to not only have less pectin, but also an increase of pectin degradation by PMEs and PG and other cell wall and cuticle defects (Du et al., 2020; Lorrai et al., 2021; Barnes et al., 2022; Grandjean et al., 2023) reminiscent to what takes place during abscission event. It is thus difficult to know if this relative increase in cell wall remodeling is in fact a sign of abscission-like event triggered throughout the plant in this mutant background or only a sign that such cell wall remodeling is normally down regulated by a functioning adhesion maintenance feedback mechanism. We also pointed out several times the similarities in terms of enzymes involved in growth and cell separation associated cell wall remodeling, but it is important to note that these enzymes are part of large families and that their activities can vary and lead to radically different effects. It is for instance proposed that some endogenous PGs have an effect specifically for expansion and other more specifically for cell separation (Ogawa et al., 2009; Xiao et al., 2017; Safran et al., 2023). Furthermore, in the long term, sustained growth also involves additional cell wall synthesis and secretion, that would compensate for some of the cell wall loosening and adhesion weakening effect of cell wall remodeling enzymes. Overall, such a difference in activities within enzyme families and presence or absence of cell wall synthesis could indicate that, although they appear similar, growth and cell separation mechanisms are really distinct processes. Nevertheless, these processes remain tightly linked, at least in terms of mechanics.
Conclusion
While much progress has been achieved in the past to characterize the processes of abscission, recent work opened the door to study how adhesion maintenance may work. However, much remains to be done to better characterize what really is adhesion and adhesion maintenance in the case of plants. What sets apart adhesion deficiency from abscission? What is the contribution of the cell wall in the interplay between adhesion, growth, and development? Future work will need to integrate not only the molecular and chemical aspects of adhesion regulation but also the mechanics and in particular the coupling with growth.
Author contributions
AB: Visualization, Writing – original draft, Writing – review & editing. SV: Conceptualization, Funding acquisition, Visualization, Writing – original draft, Writing – review & editing.
Funding
The author(s) declare financial support was received for the research, authorship, and/or publication of this article. The author(s) would like to acknowledge the financial support from the Knut and Alice Wallenberg Foundation (KAW 2016.0341 and KAW 2016.0352), the Swedish Governmental Agency for Innovation Systems (VINNOVA 2016-00504) as well as a grant from the Novo Nordisk Foundation (NNF21OC0067282) to SV. We also thank Bio4Energy, a Strategic Research Environment supported through the Swedish Government’s Strategic Research Area initiative, for supporting this work.
Acknowledgments
We are grateful to Adrien Heyman, Asal Atakhani and Ioannis Theodorou for their helpful comments on the manuscript.
Conflict of interest
The authors declare that the research was conducted in the absence of any commercial or financial relationships that could be construed as a potential conflict of interest.
The author(s) declared that they were an editorial board member of Frontiers, at the time of submission. This had no impact on the peer review process and the final decision.
Publisher’s note
All claims expressed in this article are solely those of the authors and do not necessarily represent those of their affiliated organizations, or those of the publisher, the editors and the reviewers. Any product that may be evaluated in this article, or claim that may be made by its manufacturer, is not guaranteed or endorsed by the publisher.
References
Abe M., Katsumata H., Komeda Y., Takahashi T. (2003). Regulation of shoot epidermal cell differentiation by a pair of homeodomain proteins in Arabidopsis. Development 130, 635–643. doi: 10.1242/dev.00292
Amanda D., Doblin M. S., Galletti R., Bacic A., Ingram G. C., Johnson K. L. (2016). DEFECTIVE KERNEL1 (DEK1) regulates cell walls in the leaf epidermis. Plant Physiol. 172, 2204–2218. doi: 10.1104/pp.16.01401
Anderson C. T., Kieber J. J. (2020). Dynamic construction, perception, and remodeling of plant cell walls. Annu. Rev. Plant Biol. 71, 39–69. doi: 10.1146/annurev-arplant-081519-035846
Atakhani A., Bogdziewiez L., Verger S. (2022). Characterising the mechanics of cell–cell adhesion in plants. Quant. Plant Biol. 3, e2. doi: 10.1017/qpb.2021.16
Bacete L., Hamann T. (2020). The role of mechanoperception in plant cell wall integrity maintenance. Plants 9, 574. doi: 10.3390/plants9050574
Ballester P., Ferrándiz C. (2017). Shattering fruits: variations on a dehiscent theme. Curr. Opin. Plant Biol. 35, 68–75. doi: 10.1016/j.pbi.2016.11.008
Banda J., Bellande K., Von Wangenheim D., Goh T., Guyomarc’h S., Laplaze L., et al. (2019). Lateral root formation in Arabidopsis: A well-ordered LRexit. Trends Plant Sci. 24, 826–839. doi: 10.1016/j.tplants.2019.06.015
Barnes W. J., Zelinsky E., Anderson C. T. (2022). Polygalacturonase activity promotes aberrant cell separation in the quasimodo2 mutant of Arabidopsis thaliana. Cell Surf. 8, 100069. doi: 10.1016/j.tcsw.2021.100069
Basu D., El-Assal S. E.-D., Le J., Mallery E. L., Szymanski D. B. (2004). Interchangeable functions of Arabidopsis PIROGI and the human WAVE complex subunit SRA1 during leaf epidermal development. Development 131, 4345–4355. doi: 10.1242/dev.01307
Berhin A., De Bellis D., Franke R. B., Buono R. A., Nowack M. K., Nawrath C. (2019). The root cap cuticle: A cell wall structure for seedling establishment and lateral root formation. Cell 176, 1367–1378.e8. doi: 10.1016/j.cell.2019.01.005
Bosch M., Hepler P. K. (2005). Pectin methylesterases and pectin dynamics in pollen tubes. Plant Cell 17, 3219–3226. doi: 10.1105/tpc.105.037473
Bouton S., Leboeuf E., Mouille G., Leydecker M.-T., Talbotec J., Granier F., et al. (2002). QUASIMODO1 encodes a putative membrane-bound glycosyltransferase required for normal pectin synthesis and cell adhesion in Arabidopsis. Plant Cell 14, 2577–2590. doi: 10.1105/tpc.004259
Butenko M. A., Patterson S. E., Grini P. E., Stenvik G.-E., Amundsen S. S., Mandal A., et al. (2003). Inflorescence deficient in abscission controls floral organ abscission in Arabidopsis and identifies a novel family of putative ligands in plants. Plant Cell 15, 2296–2307. doi: 10.1105/tpc.014365
Butenko M. A., Stenvik G.-E., Alm V., Sæther B., Patterson S. E., Aalen R. B. (2006). Ethylene-dependent and -independent pathways controlling floral abscission are revealed to converge using promoter::reporter gene constructs in the ida abscission mutant. J. Exp. Bot. 57, 3627–3637. doi: 10.1093/jxb/erl130
Cai S., Lashbrook C. C. (2008). Stamen abscission zone transcriptome profiling reveals new candidates for abscission control: enhanced retention of floral organs in transgenic plants overexpressing Arabidopsis ZINC FINGER PROTEIN2. Plant Physiol. 146, 1305–1321. doi: 10.1104/pp.107.110908
Chebli Y., Geitmann A. (2023). Pectate lyase-like lubricates the male gametophyte’s path toward its mating partner. Plant Physiol., 194, 124–136. doi: 10.1093/plphys/kiad481
Cho H.-T., Cosgrove D. J. (2000). Altered expression of expansin modulates leaf growth and pedicel abscission in Arabidopsis thaliana. Proc. Natl. Acad. Sci. 97, 9783–9788. doi: 10.1073/pnas.160276997
Cho S. K., Larue C. T., Chevalier D., Wang H., Jinn T.-L., Zhang S., et al. (2008). Regulation of floral organ abscission in Arabidopsis thaliana. Proc. Natl. Acad. Sci. 105, 15629–15634. doi: 10.1073/pnas.0805539105
Coen E., Cosgrove D. J. (2023). The mechanics of plant morphogenesis. Science 379, eade8055. doi: 10.1126/science.ade8055
Cosgrove D. J. (2016). Plant cell wall extensibility: connecting plant cell growth with cell wall structure, mechanics, and the action of wall-modifying enzymes. J. Exp. Bot. 67, 463–476. doi: 10.1093/jxb/erv511
Cosgrove D. J. (2022). Building an extensible cell wall. Plant Physiol. 189, 1246–1277. doi: 10.1093/plphys/kiac184
Cosgrove D. J. (2023). Structure and growth of plant cell walls. Nat. Rev. Mol. Cell Biol. doi: 10.1038/s41580-023-00691-y
Daher F. B., Braybrook S. A. (2015). How to let go: pectin and plant cell adhesion. Front. Plant Sci. 6. doi: 10.3389/fpls.2015.00523
Driouich A., Durand C., Vicré-Gibouin M. (2007). Formation and separation of root border cells. Trends Plant Sci. 12, 14–19. doi: 10.1016/j.tplants.2006.11.003
Du J., Kirui A., Huang S., Wang L., Barnes W. J., Kiemle S. N., et al. (2020). Mutations in the pectin methyltransferase QUASIMODO2 influence cellulose biosynthesis and wall integrity in Arabidopsis. Plant Cell 32, 3576–3597. doi: 10.1105/tpc.20.00252
Dyachok J., Shao M.-R., Vaughn K., Bowling A., Facette M., Djakovic S., et al. (2008). Plasma membrane-associated SCAR complex subunits promote cortical F-actin accumulation and normal growth characteristics in Arabidopsis roots. Mol. Plant 1, 990–1006. doi: 10.1093/mp/ssn059
Earles J. M., Theroux-Rancourt G., Roddy A. B., Gilbert M. E., McElrone A. J., Brodersen C. R. (2018). Beyond porosity: 3D leaf intercellular airspace traits that impact mesophyll conductance. Plant Physiol. 178, 148–162. doi: 10.1104/pp.18.00550
El-Assal S. E.-D., Le J., Basu D., Mallery E. L., Szymanski D. B. (2004). Arabidopsis GNARLED encodes a NAP125 homolog that positively regulates ARP2/3. Curr. Biol. 14, 1405–1409. doi: 10.1016/j.cub.2004.06.062
Estornell L. H., Wildhagen M., Pérez-Amador M. A., Talón M., Tadeo F. R., Butenko M. A. (2015). The IDA peptide controls abscission in Arabidopsis and citrus. Front. Plant Sci. 6. doi: 10.3389/fpls.2015.01003
Feng W., Kita D., Peaucelle A., Cartwright H. N., Doan V., Duan Q., et al. (2018). The FERONIA Receptor Kinase Maintains Cell-Wall Integrity during Salt Stress through Ca2+ Signaling. Curr. Biol. 28, 666–675.e5. doi: 10.1016/j.cub.2018.01.023
Francis K. E., Lam S. Y., Copenhaver G. P. (2006). Separation of Arabidopsis pollen tetrads is regulated by QUARTET1, a pectin methylesterase gene. Plant Physiol. 142, 1004–1013. doi: 10.1104/pp.106.085274
Galletti R., Johnson K. L., Scofield S., San-Bento R., Watt A. M., Murray J. A. H., et al. (2015). DEFECTIVE KERNEL 1 promotes and maintains plant epidermal differentiation. Development 142, 1978–1983. doi: 10.1242/dev.122325
Galletti R., Verger S., Hamant O., Ingram G. C. (2016). Developing a ‘thick skin’: a paradoxical role for mechanical tension in maintaining epidermal integrity? Development 143, 3249–3258. doi: 10.1242/dev.132837
Gorshkova T., Brutch N., Chabbert B., Deyholos M., Hayashi T., Lev-Yadun S., et al. (2012). Plant fiber formation: state of the art, recent and expected progress, and open questions. Crit. Rev. Plant Sci. 31, 201–228. doi: 10.1080/07352689.2011.616096
Grandjean C., Voxeur A., Chabout S., Jobert F., Gutierrez L., Pelloux J., et al. (2023). Fine-tuning and remodelling of pectins play a key role in the maintenance of cell adhesion. bioRxiv. doi: 10.1101/2023.10.27.564335
Gray-Mitsumune M., Blomquist K., McQueen-Mason S., Teeri T. T., Sundberg B., Mellerowicz E. J. (2008). Ectopic expression of a wood-abundant expansin PttEXPA1 promotes cell expansion in primary and secondary tissues in aspen. Plant Biotechnol. J. 6, 62–72. doi: 10.1111/j.1467-7652.2007.00295.x
Gray-Mitsumune M., Mellerowicz E. J., Abe H., Schrader J., Winzéll A., Sterky F., et al. (2004). Expansins abundant in secondary xylem belong to subgroup A of the α -expansin gene family. Plant Physiol. 135, 1552–1564. doi: 10.1104/pp.104.039321
Gubert C. M., Christy M. E., Ward D. L., Groner W. D., Liljegren S. J. (2014). ASYMMETRIC LEAVES1 regulates abscission zone placement in Arabidopsis flowers. BMC Plant Biol. 14, 195. doi: 10.1186/s12870-014-0195-5
Hawes M. C., Gunawardena U., Miyasaka S., Zhao X. (2000). The role of root border cells in plant defense. Trends Plant Sci. 5, 128–133. doi: 10.1016/S1360-1385(00)01556-9
Iida H., Mähönen A. P., Jürgens G., Takada S. (2023). Epidermal injury-induced derepression of key regulator ATML1 in newly exposed cells elicits epidermis regeneration. Nat. Commun. 14, 1031. doi: 10.1038/s41467-023-36731-6
Im K.-H., Cosgrove D. J., Jones A. M. (2000). Subcellular localization of expansin mRNA in xylem cells. Plant Physiol. 123, 463–470. doi: 10.1104/pp.123.2.463
Israelsson M., Eriksson M. E., Hertzberg M., Aspeborg H., Nilsson P., Moritz T. (2003). Changes in gene expression in the wood-forming tissue of transgenic hybrid aspen with increased secondary growth. Plant Mol. Biol. 52, 893–903. doi: 10.1023/A:1025097410445
Jarvis M. C. (1998). Intercellular separation forces generated by intracellular pressure. Plant Cell Environ. 21, 1307–1310. doi: 10.1046/j.1365-3040.1998.00363.x
Jarvis M. C., Briggs S. P. H., Knox J. P. (2003). Intercellular adhesion and cell separation in plants. Plant Cell Environ. 26, 977–989. doi: 10.1046/j.1365-3040.2003.01034.x
Johnson K. L., Degnan K. A., Ross Walker J., Ingram G. C. (2005). AtDEK1 is essential for specification of embryonic epidermal cell fate. Plant J. 44, 114–127. doi: 10.1111/j.1365-313X.2005.02514.x
Kamel H., Geitmann A. (2023). Strength in numbers: An isoform variety of homogalacturonan modifying enzymes may contribute to pollen tube fitness. Plant Physiol., 194, 67–80. doi: 10.1093/plphys/kiad544
Kelly-Bellow R., Lee K., Kennaway R., Barclay J. E., Whibley A., Bushell C., et al. (2023). Brassinosteroid coordinates cell layer interactions in plants via cell wall and tissue mechanics. Science 380, 1275–1281. doi: 10.1126/science.adf0752
Knox J. P. (1992). Cell adhesion, cell separation and plant morphogenesis. Plant J. 2, 137–141. doi: 10.1111/j.1365-313X.1992.00137.x
Kohorn B. D., Greed B. E., Mouille G., Verger S., Kohorn S. L. (2021). Effects of Arabidopsis wall associated kinase mutations on ESMERALDA1 and elicitor induced ROS. PloS One 16, e0251922. doi: 10.1371/journal.pone.0251922
Kohorn B. D., Yang N., Weinstock M., Asper G., Ball I., Rajiv D. (2023). Golgi ELMO1 binds QUA1, QUA2, GAUT9, and ELMO4 and is required for pectin accumulation in Arabidopsis. PloS One 18, e0293961. doi: 10.1371/journal.pone.0293961
Krupková E., Immerzeel P., Pauly M., Schmülling T. (2007). The TUMOROUS SHOOT DEVELOPMENT2 gene of Arabidopsis encoding a putative methyltransferase is required for cell adhesion and co-ordinated plant development. Plant J. 50, 735–750. doi: 10.1111/j.1365-313X.2007.03123.x
Kumpf R. P., Shi C.-L., Larrieu A., Stø I. M., Butenko Melinka A., Péret B., et al. (2013). Floral organ abscission peptide IDA and its HAE/HSL2 receptors control cell separation during lateral root emergence. Proc. Natl. Acad. Sci. 110, 5235–5240. doi: 10.1073/pnas.1210835110
Kuriyama H., Fukuda H. (2007). “Vascular cell differentiation,” in Plant Cell Separation and Adhesion. Eds. Roberts J. A., Gonzalez-Carranza Z. (Blackwell Publishing Ltd, Oxford, UK), 40–68. doi: 10.1002/9780470988824.ch3
Kushwah S., Banasiak A., Nishikubo N., Derba-Maceluch M., Majda M., Endo S., et al. (2020). Arabidopsis XTH4 and XTH9 contribute to wood cell expansion and secondary wall formation. Plant Physiol. 182, 1946–1965. doi: 10.1104/pp.19.01529
Kutschera U., Niklas K. J. (2007). The epidermal-growth-control theory of stem elongation: An old and a new perspective. J. Plant Physiol. 164, 1395–1409. doi: 10.1016/j.jplph.2007.08.002
Lathe R. S., McFarlane H. E., Khan G. A., Ebert B., Ramírez-Rodríguez E. A., Noord N., et al. (2021). A DUF1068 protein acts as a pectin biosynthesis scaffold and maintains Golgi morphology and cell adhesion in Arabidopsis. bioRxiv. doi: 10.1101/2021.05.03.442108. 2021.05.03.442108.
Le J., El-Assal S. E.-D., Basu D., Saad M. E., Szymanski D. B. (2003). Requirements for Arabidopsis ATARP2 and ATARP3 during epidermal development. Curr. Biol. 13, 1341–1347. doi: 10.1016/S0960-9822(03)00493-7
Leslie M. E., Lewis M. W., Liljegren S. J. (2007). “Organ abscission,” in Plant Cell Separation and Adhesion. Eds. Roberts J. A., Gonzalez-Carranza Z. (Blackwell Publishing Ltd, Oxford, UK), 106–136. doi: 10.1002/9780470988824.ch6
Lev-Yadun S. (2001). Intrusive growth – the plant analog of dendrite and axon growth in animals. New Phytol. 150, 508–512. doi: 10.1046/j.1469-8137.2001.00143.x
Lin P.-A., Chen Y., Ponce G., Acevedo F. E., Lynch J. P., Anderson C. T., et al. (2022). Stomata-mediated interactions between plants, herbivores, and the environment. Trends Plant Sci. 27, 287–300. doi: 10.1016/j.tplants.2021.08.017
Lorrai R., Francocci F., Gully K., Martens H. J., De Lorenzo G., Nawrath C., et al. (2021). Impaired Cuticle Functionality and Robust Resistance to Botrytis cinerea in Arabidopsis thaliana Plants With Altered Homogalacturonan Integrity Are Dependent on the Class III Peroxidase AtPRX71. Front. Plant Sci. 12. doi: 10.3389/fpls.2021.696955
Lucas W. J., Groover A., Lichtenberger R., Furuta K., Yadav S., Helariutta Y., et al. (2013). The plant vascular system: evolution, development and functions F. J. Integr. Plant Biol. 55, 294–388. doi: 10.1111/jipb.12041
Majda M., Kozlova L., Banasiak A., Derba-Maceluch M., Iashchishyn I. A., Morozova-Roche L. A., et al. (2021). Elongation of wood fibers combines features of diffuse and tip growth. New Phytol. 232, 673–691. doi: 10.1111/nph.17468
Malek B. R., Mouille G. (2023). “The middle lamella between anthropic and evolutionary contexts,” in Plant Cell Walls: Research Milestones and Conceptual Insights (1st ed.) (CRC Press, Boca Raton). doi: 10.1201/9781003178309
Malivert A., Erguvan Ö., Chevallier A., Dehem A., Friaud R., Liu M., et al. (2021). FERONIA and microtubules independently contribute to mechanical integrity in the Arabidopsis shoot. PloS Biol. 19, e3001454. doi: 10.1371/journal.pbio.3001454
Mao L., Begum D., Chuang H., Budiman M. A., Szymkowiak E. J., Irish E. E., et al. (2000). JOINTLESS is a MADS-box gene controlling tomato flower abscissionzone development. Nature 406, 910–913. doi: 10.1038/35022611
Marsollier A.-C., Ingram G. (2018). Getting physical: invasive growth events during plant development. Curr. Opin. Plant Biol. 46, 8–17. doi: 10.1016/j.pbi.2018.06.002
Mathur J., Mathur N., Kernebeck B., Hülskamp M. (2003). Mutations in actin-related proteins 2 and 3 affect cell shape development in Arabidopsis. Plant Cell 15, 1632–1645. doi: 10.1105/tpc.011676
Meir S., Philosoph-Hadas S., Sundaresan S., Selvaraj K. S. V., Burd S., Ophir R., et al. (2010). Microarray analysis of the abscission-related transcriptome in the tomato flower abscission zone in response to auxin depletion. Plant Physiol. 154, 1929–1956. doi: 10.1104/pp.110.160697
Melnyk C. W. (2017). Plant grafting: insights into tissue regeneration. Regeneration 4, 3–14. doi: 10.1002/reg2.2017.4.issue-1
Mielke S., Zimmer M., Meena M. K., Dreos R., Stellmach H., Hause B., et al. (2021). Jasmonate biosynthesis arising from altered cell walls is prompted by turgor-driven mechanical compression. Sci. Adv. 7, eabf0356. doi: 10.1126/sciadv.abf0356
Mollet J.-C., Faugeron C., Morvan H. (2007). “Cell adhesion, separation and guidance in compatible plant reproduction,” in Plant Cell Separation and Adhesion. Eds. Roberts J. A., Gonzalez-Carranza Z. (Blackwell Publishing Ltd), 69–90, Oxford, UK). doi: 10.1002/9780470988824.ch4
Mouille G., Ralet M.-C., Cavelier C., Eland C., Effroy D., Hématy K., et al. (2007). Homogalacturonan synthesis in Arabidopsis thaliana requires a Golgi-localized protein with a putative methyltransferase domain. Plant J. 50, 605–614. doi: 10.1111/j.1365-313X.2007.03086.x
Mravec J. (2017). Border cell release: Cell separation without cell wall degradation? Plant Signal. Behav. 12, e1343778. doi: 10.1080/15592324.2017.1343778
Mravec J., Guo X., Hansen A. R., Schückel J., Kračun S. K., Mikkelsen M. D., et al. (2017). Pea border cell maturation and release involve complex cell wall structural dynamics. Plant Physiol. 174, 1051–1066. doi: 10.1104/pp.16.00097
Mravec J., Kračun S. K., Rydahl M. G., Westereng B., Miart F., Clausen M. H., et al. (2014). Tracking developmentally regulated post-synthetic processing of homogalacturonan and chitin using reciprocal oligosaccharide probes. Development 141, 4841–4850. doi: 10.1242/dev.113365
Neumetzler L., Humphrey T., Lumba S., Snyder S., Yeats T. H., Usadel B., et al. (2012). The FRIABLE1 gene product affects cell adhesion in Arabidopsis. PloS One 7, e42914. doi: 10.1371/journal.pone.0042914
Ogawa M., Kay P., Wilson S., Swain S. M. (2009). ARABIDOPSIS DEHISCENCE ZONE POLYGALACTURONASE1 (ADPG1), ADPG2, and QUARTET2 are polygalacturonases required for cell separation during reproductive development in Arabidopsis. Plant Cell 21, 216–233. doi: 10.1105/tpc.108.063768
Patharkar O. R., Walker J. C. (2018). Advances in abscission signaling. J. Exp. Bot. 69, 733–740. doi: 10.1093/jxb/erx256
Pratap Sahi V., Cifrová P., García-González J., Kotannal Baby I., Mouillé G., Gineau E., et al. (2017). Arabidopsis thaliana plants lacking the ARP2/3 complex show defects in cell wall assembly and auxin distribution. Ann. Bot 122, 777–789. doi: 10.1093/aob/mcx178
Qiu J.-L., Jilk R., Marks M. D., Szymanski D. B. (2002). The Arabidopsis SPIKE1 gene is required for normal cell shape control and tissue development. Plant Cell 14, 101–118. doi: 10.1105/tpc.010346
Reiche C. (1885). Über anatomische Veränderungen, welche in den Perianthkreisen der Blüten wahrend der Entwickelung der Frucht vor sich gehen. Jahrb. Wissensch. Bot. 16, 638–687.
Rhee S. Y., Osborne E., Poindexter P. D., Somerville C. R. (2003). Microspore separation in the quartet 3 mutants of Arabidopsis is impaired by a defect in a developmentally regulated polygalacturonase required for pollen mother cell wall degradation. Plant Physiol. 133, 1170–1180. doi: 10.1104/pp.103.028266
Safran J., Tabi W., Ung V., Lemaire A., Habrylo O., Bouckaert J., et al. (2023). Plant polygalacturonase structures specify enzyme dynamics and processivities to fine-tune cell wall pectins. Plant Cell 35, 3073–3091. doi: 10.1093/plcell/koad134
Sanati Nezhad A., Geitmann A. (2013). The cellular mechanics of an invasive lifestyle. J. Exp. Bot. 64, 4709–4728. doi: 10.1093/jxb/ert254
Sanati Nezhad A., Naghavi M., Packirisamy M., Bhat R., Geitmann A. (2013). Quantification of cellular penetrative forces using lab-on-a-chip technology and finite element modeling. Proc. Natl. Acad. Sci. 110, 8093–8098. doi: 10.1073/pnas.1221677110
Sapala A., Runions A., Routier-Kierzkowska A.-L., Das Gupta M., Hong L., Hofhuis H., et al. (2018). Why plants make puzzle cells, and how their shape emerges. eLife 7, e32794. doi: 10.7554/eLife.32794.035
Shi C.-L., Alling R. M., Hammerstad M., Aalen R. B. (2019). Control of organ abscission and other cell separation processes by evolutionary conserved peptide signaling. Plants 8, 225. doi: 10.3390/plants8070225
Shi C.-L., Stenvik G.-E., Vie A. K., Bones A. M., Pautot V., Proveniers M., et al. (2011). Arabidopsis class I KNOTTED-like homeobox proteins act downstream in the IDA-HAE/HSL2 floral abscission signaling pathway. Plant Cell 23, 2553–2567. doi: 10.1105/tpc.111.084608
Shi C.-L., Von Wangenheim D., Herrmann U., Wildhagen M., Kulik I., Kopf A., et al. (2018). The dynamics of root cap sloughing in Arabidopsis is regulated by peptide signalling. Nat. Plants 4, 596–604. doi: 10.1038/s41477-018-0212-z
Siedlecka A., Wiklund S., Péronne M.-A., Micheli F., Lesniewska J., Sethson I., et al. (2008). Pectin methyl esterase inhibits intrusive and symplastic cell growth in developing wood cells of Populus. Plant Physiol. 146, 554–565. doi: 10.1104/pp.107.111963
Taesakul P., Siriphanich J., Van Doorn W. G. (2015). Two abscission zones proximal to Lansium domesticum fruit: one more sensitive to exogenous ethylene than the other. Front. Plant Sci. 6. doi: 10.3389/fpls.2015.00264
Takenaka Y., Kato K., Ogawa-Ohnishi M., Tsuruhama K., Kajiura H., Yagyu K., et al. (2018). Pectin RG-I rhamnosyltransferases represent a novel plant-specific glycosyltransferase family. Nat. Plants 4, 669–676. doi: 10.1038/s41477-018-0217-7
Taylor J. E., Whitelaw C. A. (2001). Signals in abscission. New Phytol. 151, 323–340. doi: 10.1046/j.0028-646x.2001.00194.x
Temple H., Phyo P., Yang W., Lyczakowski J. J., Echevarría-Poza A., Yakunin I., et al. (2022). Golgi-localized putative S-adenosyl methionine transporters required for plant cell wall polysaccharide methylation. Nat. Plants 8, 656–669. doi: 10.1038/s41477-022-01156-4
Treado J. D., Roddy A. B., Théroux-Rancourt G., Zhang L., Ambrose C., Brodersen C. R., et al. (2022). Localized growth and remodelling drives spongy mesophyll morphogenesis. J. R. Soc Interface 19, 20220602. doi: 10.1098/rsif.2022.0602
Verger S., Chabout S., Gineau E., Mouille G. (2016). Cell adhesion in plants is under the control of putative O-fucosyltransferases. Development 143, 2536–2540. doi: 10.1242/dev.132308
Verger S., Long Y., Boudaoud A., Hamant O. (2018). A tension-adhesion feedback loop in plant epidermis. eLife 7, e34460. doi: 10.7554/eLife.34460
Wachananawat B., Kuroha T., Takenaka Y., Kajiura H., Naramoto S., Yokoyama R., et al. (2020). Diversity of pectin rhamnogalacturonan I rhamnosyltransferases in glycosyltransferase family 106. Front. Plant Sci. 11. doi: 10.3389/fpls.2020.00997
Wen F., Laskowski M., Hawes M. (2007). “Cell separation in roots,” in Plant Cell Separation and Adhesion. Eds. Roberts J. A., Gonzalez-Carranza Z. (Blackwell Publishing Ltd, Oxford, UK), 91–105. doi: 10.1002/9780470988824.ch5
Whitewoods C. D. (2021). Riddled with holes: Understanding air space formation in plant leaves. PloS Biol. 19, e3001475. doi: 10.1371/journal.pbio.3001475
Xiao C., Barnes W. J., Zamil M. S., Yi H., Puri V. M., Anderson C. T. (2017). Activation tagging of Arabidopsis POLYGALACTURONASE INVOLVED IN EXPANSION 2 promotes hypocotyl elongation, leaf expansion, stem lignification, mechanical stiffening, and lodging. Plant J. 89, 1159–1173. doi: 10.1111/tpj.13453
Yanagisawa N., Sugimoto N., Arata H., Higashiyama T., Sato Y. (2017). Capability of tip-growing plant cells to penetrate into extremely narrow gaps. Sci. Rep. 7, 1403. doi: 10.1038/s41598-017-01610-w
Zamil M. S., Geitmann A. (2017). The middle lamella—more than a glue. Phys. Biol. 14, 015004. doi: 10.1088/1478-3975/aa5ba5
Keywords: adhesion, cell wall, abscission, separation, growth
Citation: Baba AI and Verger S (2024) Cell adhesion maintenance and controlled separation in plants. Front. Plant Physiol. 2:1369575. doi: 10.3389/fphgy.2024.1369575
Received: 12 January 2024; Accepted: 08 February 2024;
Published: 26 February 2024.
Edited by:
Yohann Boutté, UMR5200 Laboratoire de biogenèse membranaire (LBM), FranceReviewed by:
Jozef Mravec, Institute of Plant Genetics and Biotechnology (SAS), SlovakiaGrégory Mouille, l’alimentation et l’environnement (INRAE), France
Copyright © 2024 Baba and Verger. This is an open-access article distributed under the terms of the Creative Commons Attribution License (CC BY). The use, distribution or reproduction in other forums is permitted, provided the original author(s) and the copyright owner(s) are credited and that the original publication in this journal is cited, in accordance with accepted academic practice. No use, distribution or reproduction is permitted which does not comply with these terms.
*Correspondence: Stéphane Verger, U3RlcGhhbmUudmVyZ2VyQHVtdS5zZQ==