- 1Chemical Institute, Umeå University, Umeå, Sweden
- 2Laboratories of Plant Physiology and Photobiology, Department of Life and Environmental Sciences, University of Cagliari, Cagliari, Italy
This mini review provides an update of the thylakoid lumen, shedding light on its intricate structure, unique proteome, and potential physiological significance. This compartment within the thylakoid membranes of chloroplasts was originally perceived as “empty”, only providing a site for proton accumulation to support ATP formation. Instead, recent investigations have revealed that the lumen houses a specific set of proteins each with potentially critical roles. The structure of this compartment has been shown to be dynamic, with changes in size and organization influenced by light exposure, impacting protein mobility and function. Noteworthy, some of the lumen proteins are permanently or transiently in contact with protein complexes located in the thylakoid membrane, such as PSII (PsbP-like and PsbQ-like proteins) cytochrome b6f, and PSI. Meanwhile, other lumen proteins seems to be more “independent” such as proteases, immunophilins, stress-related proteins, pentapeptide repeat proteins, and many others with unknown functions. All these proteins play crucial roles in maintaining photosynthetic machinery, adapting to environmental stress, and regulating cellular processes. Understanding the lumen’s function is vital as it holds promise for uncovering novel regulatory interactions and signaling pathways within the chloroplast.
1 Introduction
The process of photosynthesis, in which the solar energy is converted to bound chemical energy in the form of starch, is a specific characteristic of plants, algae, and cyanobacteria. In plants, this process takes place in a specific organelle, the chloroplast. The chloroplast has a double membrane, the envelope, which substantiates its bacterial origin. Inside the chloroplast, the thylakoid membranes are located, hosting Photosystems I and II (PSI and PSII), the cytochrome b6f complex, and ATP-synthase supramolecular complexes. The thylakoid membrane has a fascinating architecture, differentiated into cylindrical grana stacks and tubular stroma lamellae. Nestled within the thylakoid membrane is the thylakoid lumen, which forms a continuous compartment with the thylakoid membrane at its outer border. This secluded compartment emerges as a microscopic realm of immense significance in the process of photosynthesis. In this mini review, we provide an update on the content of this previously considered “empty” chloroplast compartment.
The main function of the thylakoid lumen is accumulation of protons to support ATP formation via ATP-synthase and only a few proteins were suggested to be in this compartment: two extrinsic PSII proteins (PsbP and PsbQ) and plastocyanin, the electron linker between PSII and PSI. Thus, many textbooks show an empty lumen space with protons (H+) inside. It has been 25 years since the first attempts to obtain a detailed general analysis of the lumen content was published (Kieselbach et al., 1998a). This paved the way for a more detailed proteomic analysis of this compartment until recent days (Kieselbach et al., 2000; Bricker et al., 2001; Peltier et al., 2002; Schubert et al., 2002; Järvi et al., 2013; Gollan et al., 2021). It is now well-established that the thylakoid lumen has a specific proteome (Table 1) that is distinct and separate from the stroma and cytoplasm compartments, containing several unique proteins and protein groups. The protein concentration in the lumen has been estimated to be 20 mg of proteins per mL, resulting in a protein concentration of the more abundant lumen proteins in the micro to millimolar range, which is similar to that of many stroma proteins (Kieselbach et al., 1998a). Interestingly, already in 1988 (Weibull and Alertsson, 1988), it was suggested that the lumen could contain proteins, as the space between the thylakoid membranes in electron microscopy studies was not transparent.
In the pioneer proteomic studies, the lumen protein content was estimated to be 80 (Schubert et al., 2002) or even up to 200 (Peltier et al., 2002) distinct proteins. In Table 1, we have, to the best of our knowledge, accumulated all the lumen protein identified or suggested in the literature so far. Table 1 contains 62 proteins that have been biochemically identified in various lumen extractions, and at least 16 further proteins have been predicted through various genome searches and the prediction of signal peptides and cleaving sites (Peltier et al., 2002; Schubert et al., 2002; Gollan et al., 2021), thus suggesting their import into the lumen. This makes a total of 78 lumen proteins at present time. Several of these lumen proteins have been found to have homologs in Synechocystis sp. PCC 6803 (see Table 4 in Kieselbach and Schröder, 2003). Of the 78 proteins, 43 have been both predicted and biochemically identified, giving stronger evidence for their location in the lumen. The functions of these proteins will be discussed in a separate section.
2 Structure and dynamics of the thylakoid lumen
The introduction of electron microscopy in the 1930s enabled a significant advancement from the “green dot” chloroplasts previously observed using light microscopy. More details could be seen, and new 3D structures of the thylakoid membrane were developed. A thorough description of the history of how microscopic studies have advanced the understanding of plant thylakoids can be found in (Staehelin and Paolillo, 2020). A typical thylakoid grana stack has a diameter of 300nm, containing 3-19 (average of 9) grana sacks with an average height of 160 nm (Bussi et al., 2019). In the helical fretwork model (Paolillo, 1970) each granum is surrounded by multiple stroma lamellae connected to the granum by slits at the rim of the grana stack (Mustárdy et al., 2008; Nevo et al., 2009; Daum et al., 2010). This model has been modified based on electron tomography studies resulting in the “bifurcation model”, in which the stroma lamella is located perpendicularly to the grana and connected by slit-like apertures to the rim of the grana stacks (Figure 1 top). The connection points form a right-handed helical structure around the grana (Mustárdy et al., 2008; Daum et al., 2010; Austin and Staehelin, 2011). Recently, a mix of right and left-handed connection points between the grana and stroma membrane was suggested to reduce twisting tension (Bussi et al., 2019).
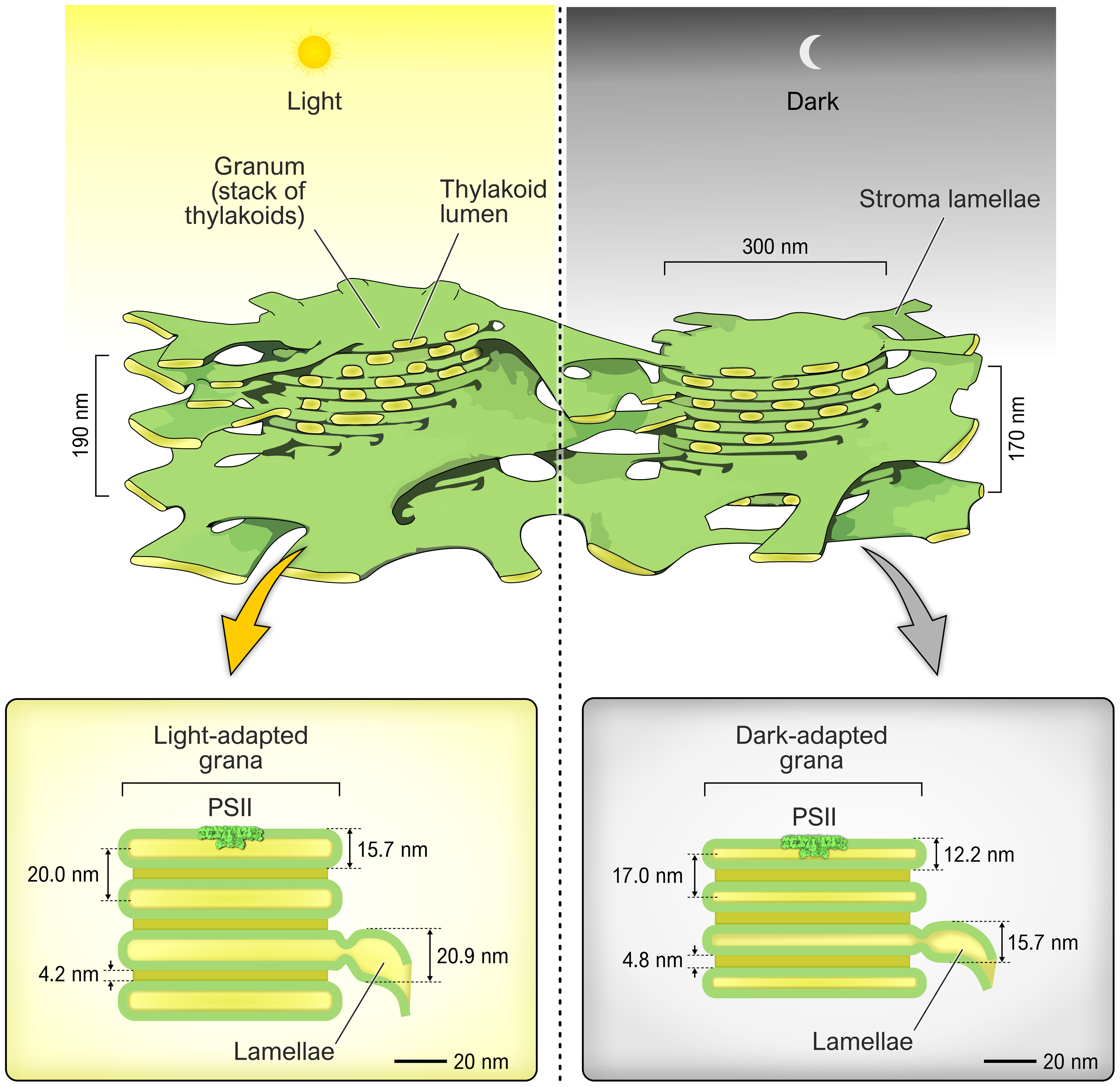
Figure 1 The top part shows two typical grana stacks in light (left) and dark (right) and their average sizes (modified from Staehelin and Paolillo, 2020). The lower part shows the distances of the various thylakoid membrane parts as Li et al. (2020) measured. The colour of the thylakoid lumen is yellow while the thylakoid membrane is green. The Photosystem II complex is shown in scale to the thylakoid membrane.
The original model of the thylakoid membrane had long extensions of the grana stacks forming stroma lamellae (see for instance Kieselbach and Schröder, 2003). This model implied a fast and free flow of proteins between the two membrane sections. However, the new structural findings with slit-like apertures between grana and stroma lamellae questions how freely proteins can move within the lumen compartment and/or between grana stroma regions. Restricted mobility between the two regions is supported by the finding that protein movement in the grana stack region is less than 20% compared to 50% in the stroma lamellae (Murphy, 1986; Kirchhoff et al., 2011; Kirchhoff et al., 2013). This could explain why some lumen-identified proteins are present in higher concentrations in grana, while others are more present in stroma fractions (Gollan et al., 2021). It must be kept in mind that most of the lumen-located proteins do not seem to form complexes, which would make their movement even more difficult.
The number of stacked membranes in the grana is dependent on the light conditions. At low light more grana are formed and the diameter of the grana stack increases (Anderson et al., 2012). There have also been publications suggesting that the width of the lumen in the grana can vary, especially between light-adapted and dark-adapted plants (Murakami and Packer, 1970; Kirchhoff et al., 2011). Recently, based on optimized fast sample fixation techniques for electron microscopy, it was possible to obtain numbers for the swelling of the lumen upon light exposure from Arabidopsis (Li et al., 2020). Li et al. (2020) showed that the swelling occurs both in the grana stack and in the stroma lamellae (Figure 1, lower part). The grana sack in the dark was found to be 12.2 nm high, meanwhile in the light this increased to 15.7 nm (Li et al., 2020). Assuming an average of 9 sacks in a granum stack, the dark grana stack will have a height of 170 nm height, while the light exposed photosynthesising grana stack will expand to 190 nm. If we assume that the thylakoid membrane is 4.0 nm on an average, then the inner lumen distance in the grana sack in light is 7.7 nm but only 4.2 nm in the dark (Figure 1, lower part). Meanwhile the diameter of the grana stack has been reported to be unchanged in different light conditions and also between plant species (Albertsson and Andreasson, 2004). These findings point toward some new ideas with respect to the thylakoid lumen; The light expansion of the lumen leads to a dilution (40-50%) of the lumen content in the light compared to the dark. Further investigation is required to determine if such dilution would influence or change the enzymatic activity of enzymes located in the lumen. An additional point of interest is that cryo-EM analysis shows that the PSII complex extends into the lumen by 5 nm (Graça et al., 2021), which raises the question, what happens to the extrinsic part of PSII when the space “shrinks” to only 4.2 nm in the dark (see Figure 1)? Are the extrinsic proteins PsbO, PsbP and PsbQ removed and reassembled in the light? There have been reports that the lumen contains a pool of free extrinsic proteins (Hashimoto et al., 1996). As discussed in Kirchhoff et al., 2011, the PSII complexes cannot be in a face-to-face orientation, but instead they must be staggered. The shrinking of the lumen space in the dark will severely obstruct the movement of PSII, and presumably the movement of lumen proteins. This has been shown be the case for the electron linker between PSII and PSI, plastocyanin (Kirchhoff et al., 2011). If this could lead to that the “compression” of the lumen compartment reduce or even stop electron transport and or enzymatic activity in the dark, needs to be further investigated.
3 The lumen proteins
The thylakoid lumen is a highly unusual compartment with a proteome that is unique not only in the plant kingdom but also in the other kingdoms of life. Below is a summary of the different groups of proteins found in the lumen and Table 1 gives a summary.
3.1 Photosystem II associated proteins
At the lumenal side of PSII, three PSII subunits build the so-called Oxygen Evolving Complex (OEC) which, through its manganese cluster (Mn4O5Ca), catalyzes the oxidation of water. These extrinsic subunits, namely PsbO, PsbP, and PsbQ (Kieselbach et al., 1998a), each have specific roles in maintaining the functionality of PSII. Positioned in close proximity to the manganese cluster, the PsbO subunit (of which two forms O1 and O2 have been identified in Arabidopsis) is suggested to serve as a GTPase (regulating PSII repair) (Lundin et al., 2008; Spetea, 2012). Both PsbP and PsbQ (are present in several forms vide infra) play a general role in ensuring PSII stability, and they may cooperate in orchestrating the insertion/removal of the manganese cluster during the PSII repair cycle. For more details on these intensely studied extrinsic proteins see for instance reviews De Las Rivas et al. (2007); Bricker et al. (2012), and Roose et al. (2016).
Finally, there is the HCF136 protein, which is a nuclear-encoded protein regulating the mechanism underlying the biogenesis of PSII (Meurer et al., 1998; Yu et al., 2018).
3.2 PsbP-like proteins
As above-mentioned, the PsbP protein is an extrinsic component of Photosystem II (PSII), located on the lumenal side of the thylakoid membrane and involved in the lumenal part of the oxygen evolving complex in higher plants. In addition to these well-studied extrinsic subunits of PSII, the thylakoid lumen contains a new protein family of PsbP-like proteins.
The PsbP gene family has ten members in the genome of the model plant Arabidopsis thaliana, with two encoding for true PsbP proteins (PsbP1 and PsbP2), and eight encoding PsbP-like proteins. Two of the eight homologs with the highest sequence similarity to PsbP1 have been classified as PsbP-like proteins (PPL1 and PPL2) (Liu et al., 2012), while the remaining six are referred to as PsbP-domain proteins (PPD). For the first two a distinct function has been assigned. In fact, the PPL1 is required for the efficient repair of photodamaged PSII and PPL2 is crucial for the accumulation of the chloroplastic NDH complex (Ishihara et al., 2007). On the contrary, no function has yet been found for any of the six PPD proteins but results from non-reducing/reducing electrophoresis indicate that at least some of these proteins might form complexes with other lumenal proteins. In addition, crystal structure studies suggests that the PsbP proteins bind metals (Cao et al., 2015).
3.3 PsbQ-like proteins
As in the previously mentioned scenario, there exists a group of PsbQ-like proteins that has undergone extensive investigation in A. thaliana. The NAD(P)H dehydrogenase-like (NDH) complex, which is involved in the PSI cyclic electron flow, has three lumenal PsbQ-like protein subunits referred to as PQL1, PQL2, and PQL3. While PQL3 is an assembly factor of the NDH, the other two proteins were shown to be essential for the functionality of NDH and tightly associate with the NDH-PSI supercomplex (Yabuta et al., 2010; Ishikawa et al., 2020). Nevertheless, there remains a lack of information regarding PsbQ-like proteins.
3.4 Cytochrome b6f, plastocyanin, and PSI proteins
The electron transport activity is intricately linked to the pH of the lumen through a process known as the “Q-cycle” (Crofts, 2004). During this cycle, two protons are actively transported from the stromal side to the lumen. This process involves the utilization of a portion of the electrons produced by PSII in a series of consecutive reduction and oxidation reactions involving the plastoquinone (PQ), a membrane-soluble carrier responsible for transporting both electrons and protons. Subsequently, these electrons are transferred to the cytochrome b6f complex (cyt b6f), which features a specific subunit known as the Rieske protein. Although this is an integral thylakoid membrane protein, it possesses a binding domain for a lumen iron-sulphur cluster (2Fe-2S), eventually playing a pivotal role in facilitating the transfer of electrons between cytochrome b6 and the cytochrome f within the complex (Carrell et al., 1997). The membrane located cyt b6f complex then channels the electrons to plastocyanin (PC), a lumenal electron carrier containing copper. The PC acts as a conduit for the electrons, ultimately delivering them to the PSI. Two homologous PC (PLAS1 and PLAS2) isoforms have been identified in the lumen compartment of which PLAS2 is expressed at considerably higher level than PLAS1. However, knockout and over-expression mutants did not reveal any specific functional difference between the two forms (Pesaresi et al., 2009). The interaction between PC and PSI is mediated by the only identified lumenal subunit of PSI, referred to as PsaN, which is essential for ensuring an efficient electron flow. Eventually, the lumen localized cytochrome c6 like protein (AT5g45040) has been hypothesized to be an alternative electron carrier in the thylakoid lumen of Arabidopsis (Gupta et al., 2002), however this role is still controversial (Weigel et al., 2003).
3.5 Proteases
The thylakoid membrane hosts the four major complexes of the photosynthetic machinery, as most of their subunits are integral membrane proteins. To adapt to changing environmental conditions in which photosynthesis operates, the turnover of several subunits becomes essential to sustain this process. Within the chloroplast, several members of the Deg proteases, ATP-independent serine-type proteases diffused across the kingdoms of life, play a crucial role. There are two members in the stroma compartment (Deg2 and Deg7) and three in the lumen (Deg1, Deg5 and Deg8), each responsible for different degradation events (Schuhmann and Adamska, 2012; Knopf and Adam, 2018).
One significant event in this context is the turnover of the D1 subunit of PSII (Knopf and Adam, 2018). This process involves a two-step procedure: first, the degradation of the exhausted D1 subunit, followed by the incorporation of a newly synthesized one, leading to the reactivation of PSII. In the initial step, Deg1 plays an essential role as it cleaves the hydrophilic lumen-located segment of D1. Importantly, the activity of Deg1 is regulated by both pH and light. Deg1 forms an active homo-hexameric complex only under illumination when the lumen pH becomes acidic and via a dephosphorylation event, ensuring activation only in the presence of photodamage, specifically when D1 is damaged. Indeed, it is worth noting that Deg1 is not limited to the D1 subunit; it also participates in the degradation of other important photosynthetic proteins such as plastocyanin and PsbO, actively contributing to the maintenance of lumenal homeostasis.
On the other hand, the other two proteases, Deg 5 and Deg8, were observed to form a hetero-oligomeric complex with a 1:1 stoichiometry. These proteases are known to collaborate with Deg1 in the initial cleavage of D1 and may potentially play a role in the degradation of the PsbF subunit of PSII (Knopf and Adam, 2018).
3.6 Immunophilins
The immunophilin superfamily comprises a group of conserved and widely distributed proteins, consisting of two structurally distinct subfamilies, each sharing a common enzymatic activity: the cyclophilins and the FK506/rapamycin-binding proteins (FKBPs) (Gollan et al., 2012). Within land plants, immunophilins are found in various tissues and cellular compartments, including the chloroplast, where they are present in both the stroma and the lumen (Buchanan and Luan, 2005). Several examples of these proteins include:
i) TLP40, initially isolated in spinach, serves as a folding catalyst and regulator of dephosphorylation in the turnover of the D1 subunit (Fulgosi et al., 1998);
ii) Cyclophilin 28 (CYP28), which plays a role in regulating the assembly and accumulation of PSII-LHCII supercomplexes by modifying the conformation of Lhcb6 through its peptidyl prolyl cis/trans isomerase (PPIase) activity (Zhu et al., 2022);
iii) Cyclophilin 38 (CYP38), which is critically involved in the assembly and maintenance of PSII supercomplexes. CYP38 also facilitates the proper folding of the D1 and CP43 (PsbC) subunits of PSII, contributing to the successful assembly of the Oxygen Evolving Complex (OEC) (Vasudevan et al., 2012). Therefore, immunophilins play vital roles in the functioning and structural maintenance of the photosynthetic machinery within chloroplasts.
3.7 Stress-related proteins
The thylakoid lumen also contains several proteins designed to address harmful conditions, such as the presence of Reactive Oxygen Species (ROS) (Petersson et al., 2006; Foyer, 2018). One notable example is the xanthophyll cycle, during which the conversion of violaxanthin to zeaxanthin is catalyzed by the lumenal enzyme violaxanthin de-epoxidase (VDE), utilizing ascorbate as a reducing agent (Simionato et al., 2015). As previously mentioned, when exposed to intense light, the pH of the lumen decreases. This change causes VDE activation through a transition from a soluble state to an association with the thylakoid membranes, where its substrate, violaxanthin, is located. Conversely, on the stromal side, zeaxanthin epoxidase (ZE) is responsible for the conversion back to violaxanthin when light intensity decreases. Another set of pH-sensing events is associated with the PsbS protein, a 22kDa membrane protein with a multifaceted activity related mainly to PSII protection (Nicol and Croce, 2021; Fantuzzi et al., 2023). While it is not a lumen protein per se, it possesses a domain whose functionality is linked to the lumen side of the thylakoid membranes.
3.8 Pentapeptide repeat proteins
The pentapeptide repeat domain consists of tandem repeats of five amino acids, with each such repeat forming a beta-strand and constituting a quadrangle. Most PRPs are found in prokaryotes. The genome of Arabidopsis thaliana encodes for six pentapeptide repeat proteins, of which three are located in the thylakoid lumen: TL15, TL17 and TL20.3 (Kieselbach et al., 1998b). Interestingly, these PRP proteins stand out by having more than 50% predicted intrinsic disorder but no clear function has been assigned yet.
3.9 Proteins with unknown function
Surprisingly, after 25 years of research, no function has been assigned to the largest protein group in the lumen (at least 28 proteins). This show that the bottle neck in biochemistry of proteins is to find functions for them. Traditionally, to understand the function of a protein, knock-out mutants are created and the mutant plant is subjected to various stresses to determine whether a particular phenotype could be associated with the specific mutation. However, for many proteins located in the chloroplast lumen, a knock-out mutant often does not show a clear phenotype. Instead, when a phenotype is observed, questions about secondary effects are raised. One exception from this is the hcf136 mutant in which the amount of PSII is strongly reduced (Plücken et al., 2002). On the other end is the case of the TL29 protein. It is one of the most abundant proteins in the thylakoid lumen, has sequence and structural similarities typical to ascorbate peroxidases, and due to this it was actually renamed by some researchers to APX4. The TL29 protein is expressed at higher levels in light and seems to be mainly in the grana lumen close to PSII. However, crystallisation of the protein revealed that TL29 has an additional loop in the suggested active site that make the binding of the catalytically heme group impossible (Granlund et al., 2009). The isolated TL29 protein does not bind any heme group, it cannot be reconstituted with a heme group and does not have any peroxidase activity (Granlund et al., 2009). In summary we know many features of the TL29 protein, except its function.
We are entering a new era where not only protein sequences but also structure predictions and searches can be made by Artificial intelligence (AI). The AlphaFold database (Varadi et al., 2022) that contains the dizzying amount of 214 million predicted proteins can be searched using programs such as Foldseek (Barrio-Hernandez et al., 2023). Obtaining or prediction of the structures for the proteins in the lumen with unknown function could through this type of searches identify remote structural similarity and identify domain families. This could then lead to clues for their function and an opening to better understand the thylakoid lumen proteome.
4 The function of lumen compartment
There is clear evidence that the chloroplast lumen is closely linked to plant cell signalling. For instance, most lumenal proteins are light-dependently co-expressed (Granlund et al., 2009) and respond to cold (Goulas et al., 2006). The extrinsic PsbP protein is considerably affected by viral infection and oxidative stress (Pérez-Bueno et al., 2004; Karamoko et al., 2011). In addition, there is evidence that the function of the STN7 kinase depends on the correct folding of its C-terminus through its lumenal disulfide bridge (Lemeille et al., 2009; Kieselbach, personal observation). There is also clear evidence that lumenal proteins have important functions in the assembly of photosystem II (Meurer et al., 1998; Plücken et al., 2002; Che et al., 2013) and its supercomplexes (Lima et al., 2006; Fu et al., 2007), but little is known about the details and the time course of the assembly process. For instance, the lumenal chloroplast proteome of Arabidopsis thaliana has eight immunophilins, which is the highest number known for a subcellular compartment. In addition, it also includes ten PsbP proteins that are unique to the thylakoid lumen and three pentapeptide repeat proteins, accounting for half of the members of this protein family in Arabidopsis. Furthermore, 20 of the 45 experimentally confirmed lumenal proteins of Arabidopsis (which is more than 40%) are in vitro targets of thioredoxin (Hall et al., 2010), which are enzymes found in each compartment of the chloroplast except for the inner space for the envelope (Kang and Wang, 2016). For a comparison, in the chloroplast stroma, about 2% of the known proteins are in vitro targets of thioredoxin (Lindahl and Kieselbach, 2009) even though the stroma contains an extended family of thioredoxins (Cain et al., 2009). This suggests that the lumen is important for thioredoxin-dependent signalling pathways. In summary, the unique features of the lumen chloroplast compartment suggest that it is a rich source for the discovery of novel knowledge and regulatory interactions between proteins.
Author contributions
DF: Writing – original draft, Writing – review & editing. WS: Writing – original draft, Writing – review & editing.
Funding
The author(s) declare that no financial support was received for the research, authorship, and/or publication of this article.
Acknowledgments
DF thanks the Carl Tryggers Foundation for Scientific Research (CTS 19:324). Dr. Dmitry Shevela (Scigrafik, Sweden) is acknowledged for preparation of Figure 1. We also thank Dr. T. Kieselbach for useful comments on the manuscript.
Conflict of interest
The authors declare that the research was conducted in the absence of any commercial or financial relationships that could be construed as a potential conflict of interest.
The author(s) WS declared that they were an editorial board member of Frontiers, at the time of submission. This had no impact on the peer review process and the final decision.
Publisher’s note
All claims expressed in this article are solely those of the authors and do not necessarily represent those of their affiliated organizations, or those of the publisher, the editors and the reviewers. Any product that may be evaluated in this article, or claim that may be made by its manufacturer, is not guaranteed or endorsed by the publisher.
References
Albertsson P. A., Andreasson E. (2004). The constant proportion of grana and stroma lamellae in plant chloroplasts. Physiol. Plant 121 (2), 334–342. doi: 10.1111/j.0031-9317.2004.00315.x
Anderson J. M., Horton P., Kim E. H., Chow W. S. (2012). Towards elucidation of dynamic structural changes of plant thylakoid architecture. Philos. Trans. R Soc. Lond B Biol. Sci. 367 (1608), 3515–3524. doi: 10.1098/rstb.2012.0373
Austin J. R., Staehelin L. A. (2011). Three-dimensional architecture of grana and stroma thylakoids of higher plants as determined by electron tomography. Plant Physiol. 155 (4), 1601–1611. doi: 10.1104/pp.110.170647
Barrio-Hernandez I., Yeo J., Jänes J., Mirdita M., Gilchrist C. L. M., Wein T., et al. (2023). Clustering predicted structures at the scale of the known protein universe. Nature. doi: 10.1038/s41586-023-06510-w
Bricker T. M., Prevost M., Vu V., Laborde S., Womack J., Frankel L. K. (2001). Isolation of lumenal proteins from spinach thylakoid membranes by triton X-114 phase partitioning. Biochim. Biophys. Acta 1503 (3), 350–356. doi: 10.1016/s0005-2728(00)00212-7
Bricker T. M., Roose J. L., Fagerlund R. D., Frankel L. K., Eaton-Rye J. J. (2012). The extrinsic proteins of Photosystem II. Biochim. Biophys. Acta 1817 (1), 121–142. doi: 10.1016/j.bbabio.2011.07.006
Buchanan B. B., Luan S. (2005). Redox regulation in the chloroplast thylakoid lumen: a new frontier in photosynthesis research. J. Exp. Bot. 56 (416), 1439–1447. doi: 10.1093/jxb/eri158
Bussi Y., Shimoni E., Weiner A., Kapon R., Charuvi D., Nevo R., et al. (2019). Fundamental helical geometry consolidates the plant photosynthetic membrane. Proc. Natl. Acad. Sci. U. S. A. 116 (44), 22366–22375. doi: 10.1073/pnas.1905994116
Cain P., Hall M., Schröder W. P., Kieselbach T., Robinson C. (2009). A novel extended family of stromal thioredoxins. Plant Mol. Biol. 70 (3), 273–281. doi: 10.1007/s11103-009-9471-4
Cao P., Xie Y., Li M., Pan X., Zhang H., Zhao X., et al. (2015). Crystal structure analysis of extrinsic PsbP protein of photosystem II reveals a manganese-induced conformational change. Mol. Plant 8 (4), 664–666. doi: 10.1016/j.molp.2015.01.002
Carrell C. J., Zhang H., Cramer W. A., Smith J. L. (1997). Biological identity and diversity in photosynthesis and respiration: structure of the lumen-side domain of the chloroplast Rieske protein. Structure 5 (12), 1613–1625. doi: 10.1016/s0969-2126(97)00309-2
Che Y., Fu A., Hou X., McDonald K., Buchanan B. B., Huang W., et al. (2013). C-terminal processing of reaction center protein D1 is essential for the function and assembly of photosystem II in Arabidopsis. Proc. Natl. Acad. Sci. U. S. A. 110 (40), 16247–16252. doi: 10.1073/pnas.1313894110
Crofts A. R. (2004). The Q-cycle - A personal perspective. Photosynth. Res. 80 (1-3), 223–243. doi: 10.1023/B:PRES.0000030444.52579.10
Daum B., Nicastro D., Austin J. 2nd, McIntosh J. R., Kühlbrandt W. (2010). Arrangement of photosystem II and ATP synthase in chloroplast membranes of spinach and pea. Plant Cell. 22 (4), 1299–1312. doi: 10.1105/tpc.109.071431
De Las Rivas J., Heredia P., Roman A. (2007). Oxygen-evolving extrinsic proteins (PsbO,P,Q,R): bioinformatic and functional analysis. Biochim. Biophys. Acta 1767 (6), 575–582. doi: 10.1016/j.bbabio.2007.01.018
Fantuzzi A., Haniewicz P., Farci D., Loi M. C., Park K., Büchel C., et al. (2023). Bicarbonate activation of the monomeric photosystem II-PsbS/Psb27 complex. Plant Physiol. 192 (4), 2656–2671. doi: 10.1093/plphys/kiad275
Foyer C. H. (2018). Reactive oxygen species, oxidative signaling and the regulation of photosynthesis. Environ. Exp. Bot. 154, 134–142. doi: 10.1016/j.envexpbot.2018.05.003
Friso G., Giacomelli L., Ytterberg A. J., Peltier J. B., Rudella A., Sun Q., et al. (2004). In-depth analysis of the thylakoid membrane proteome of Arabidopsis thaliana chloroplasts: new proteins, new functions, and a plastid proteome database. Plant Cell 16 (2), 478–499. doi: 10.1105/tpc.017814
Fu A., He Z., Cho H. S., Lima A., Buchanan B. B., Luan S. (2007). A chloroplast cyclophilin functions in the assembly and maintenance of photosystem II in Arabidopsis thaliana. Proc. Natl. Acad. Sci. U. S. A. 104 (40), 15947–15952. doi: 10.1073/pnas.0707851104
Fulgosi H., Vener A. V., Altschmied L., Herrmann R. G., Andersson B. (1998). A novel multi-functional chloroplast protein: identification of a 40 kDa immunophilin-like protein located in the thylakoid lumen. EMBO J. 17 (6), 1577–1587. doi: 10.1093/emboj/17.6.1577
Gollan P. J., Bhave M., Aro E. M. (2012). The FKBP families of higher plants: Exploring the structures and functions of protein interaction specialists. FEBS Lett. 586 (20), 3539–3547. doi: 10.1016/j.febslet.2012.09.002
Gollan P. J., Trotta A., Bajwa A. A., Mancini I., Aro E. M. (2021). Characterization of the free and membrane-associated fractions of the thylakoid lumen proteome in Arabidopsis thaliana. Int. J. Mol. Sci. 22 (15), 8126. doi: 10.3390/ijms22158126
Goulas E., Schubert M., Kieselbach T., Kleczkowski L. A., Gardeström P., Schröder W., et al. (2006). The chloroplast lumen and stromal proteomes of Arabidopsis thaliana show differential sensitivity to short- and long-term exposure to low temperature. Plant J. 47 (5), 720–734. doi: 10.1111/j.1365-313X.2006.02821.x
Graça A. T., Hall M., Persson K., Schröder W. P. (2021). High-resolution model of Arabidopsis Photosystem II reveals the structural consequences of digitonin-extraction. Sci. Rep. 11 (1), 15534. doi: 10.1038/s41598-021-94914-x
Granlund I., Storm P., Schubert M., García-Cerdán J. G., Funk C., Schröder W. P. (2009). The TL29 protein is lumen located, associated with PSII and not an ascorbate peroxidase. Plant Cell Physiol. 50 (11), 1898–1910. doi: 10.1093/pcp/pcp134
Gupta R., He Z., Luan S. (2002). Functional relationship of cytochrome c(6) and plastocyanin in Arabidopsis. Nature 417 (6888), 567–571. doi: 10.1038/417567a
Hall M., Mata-Cabana A., Akerlund H. E., Florencio F. J., Schröder W. P., Lindahl M., et al. (2010). Thioredoxin targets of the plant chloroplast lumen and their implications for plastid function. Proteomics 10 (5), 987–1001. doi: 10.1002/pmic.200900654
Hashimoto A., Yamamoto Y., Theg S. M. (1996). Unassembled subunits of the photosynthetic oxygen-evolving complex present in the thylakoid lumen are long-lived and assembly-competent. FEBS Lett. 391 (1-2), 29–34. doi: 10.1016/0014-5793(96)00686-2
Ishihara S., Takabayashi A., Ido K., Endo T., Ifuku K., Sato F. (2007). Distinct functions for the two PsbP-like proteins PPL1 and PPL2 in the chloroplast thylakoid lumen of Arabidopsis. Plant Physiol. 145 (3), 668–679. doi: 10.1104/pp.107.105866
Ishikawa N., Yokoe Y., Nishimura T., Nakano T., Ifuku K. (2020). PsbQ-like protein 3 functions as an assembly factor for the chloroplast NADH dehydrogenase-like complex in Arabidopsis. Plant Cell Physiol. 61 (7), 1252–1261. doi: 10.1093/pcp/pcaa050
Järvi S., Gollan P. J., Aro E. M. (2013). Understanding the roles of the thylakoid lumen in photosynthesis regulation. Front. Plant Sci. 4. doi: 10.3389/fpls.2013.00434
Karamoko M., Cline S., Redding K., Ruiz N., Hamel P. P. (2011). Lumen Thiol Oxidoreductase1, a disulfide bond-forming catalyst, is required for the assembly of photosystem II in Arabidopsis. Plant Cell 23 (12), 4462–4475. doi: 10.1105/tpc.111.089680
Kang Z. H., Wang G. X. (2016). Redox regulation in the thylakoid lumen. J. Plant Physiol. 192, 28–37. doi: 10.1016/j.jplph.2015.12.012
Kieselbach T., Bystedt M., Hynds P., Robinson C., Schröder W. P. (2000). A peroxidase homologue and novel plastocyanin located by proteomics to the Arabidopsis chloroplast thylakoid lumen. FEBS Lett. 480 (2-3), 271–276. doi: 10.1016/s0014-5793(00)01890-1
Kieselbach T., Hagman, Andersson B., Schröder W. P. (1998a). The thylakoid lumen of chloroplasts. Isolation and characterization. J. Biol. Chem. 273 (12), 6710–6716. doi: 10.1074/jbc.273.12.6710
Kieselbach T., Mant A., Robinson C., Schroder W. P. (1998b). Characterisation of an Arabidopsis cDNA encoding a thylakoid lumen protein related to a novel ‘pentapeptide repeat’ family of proteins. FEBS Lett. 428 (3), 241–244. doi: 10.1016/s0014-5793(98)00517-1
Kieselbach T., Schröder W. P. (2003). The proteome of the chloroplast lumen of higher plants. Photosynth. Res. 78 (3), 249–264. doi: 10.1023/B:PRES.0000006913.86689.f1
Kirchhoff H., Hall C., Wood M., Herbstová M., Tsabari O., Nevo R., et al. (2011). Dynamic control of protein diffusion within the granal thylakoid lumen. Proc. Natl. Acad. Sci. U. S. A. 108 (50), 20248–20253. doi: 10.1073/pnas.1104141109
Kirchhoff H., Sharpe R. M., Herbstova M., Yarbrough R., Edwards G. E. (2013). Differential mobility of pigment-protein complexes in granal and agranal thylakoid membranes of C3 and C4 plants. Plant Physiol. 161 (1), 497–507. doi: 10.1104/pp.112.207548
Knopf R. R., Adam Z. (2018). Lumenal exposed regions of the D1 protein of PSII are long enough to be degraded by the chloroplast Deg1 protease. Sci. Rep. 8 (1), 5230. doi: 10.1038/s41598-018-23578-x
Lemeille S., Willig A., Depège-Fargeix N., Delessert C., Bassi R., Rochaix J. D. (2009). Analysis of the chloroplast protein kinase Stt7 during state transitions. PloS Biol. 7 (3), e45. doi: 10.1371/journal.pbio.1000045
Li M., Mukhopadhyay R., Svoboda V., Oung H. M. O., Mullendore D. L., Kirchhoff H. (2020). Measuring the dynamic response of the thylakoid architecture in plant leaves by electron microscopy. Plant Direct. 4 (11), e00280. doi: 10.1002/pld3.280
Lima A., Lima S., Wong J. H., Phillips R. S., Buchanan B. B., Luan S. (2006). A redox-active FKBP-type immunophilin functions in accumulation of the photosystem II supercomplex in Arabidopsis thaliana. Proc. Natl. Acad. Sci. U. S. A. 103 (33), 12631–12636. doi: 10.1073/pnas.0605452103
Lindahl M., Kieselbach T. (2009). Disulphide proteomes and interactions with thioredoxin on the track towards understanding redox regulation in chloroplasts and cyanobacteria. J. Proteomics 72 (3), 416–438. doi: 10.1016/j.jprot.2009.01.003
Liu J., Yang H., Lu Q., Wen X., Chen F., Peng L., et al. (2012). PsbP-domain protein1, a nuclear-encoded thylakoid lumenal protein, is essential for photosystem I assembly in Arabidopsis. Plant Cell. 24 (12), 4992–5006. doi: 10.1105/tpc.112.106542
Lundin B., Nurmi M., Rojas-Stuetz M., Aro E. M., Adamska I., Spetea C. (2008). Towards understanding the functional difference between the two PsbO isoforms in Arabidopsis thaliana–insights from phenotypic analyses of psbo knockout mutants. Photosynth. Res. 98 (1-3), 405–414. doi: 10.1007/s11120-008-9325-y
Meurer J., Plücken H., Kowallik K. V., Westhoff P. (1998). A nuclear-encoded protein of prokaryotic origin is essential for the stability of photosystem II in Arabidopsis thaliana. EMBO J. 17 (18), 5286–5297. doi: 10.1093/emboj/17.18.5286
Murakami S., Packer L. (1970). Protonation and chloroplast membrane structure. J. Cell Biol. 47 (2), 332–351. doi: 10.1083/jcb.47.2.332
Murphy D. J. (1986). The molecular organisation of the photosyn- thetic membranes of higher plants. Biochim. Biophys. Acta 864, 33–94. doi: 10.1016/0304-4157(86)90015-8
Mustárdy L., Buttle K., Steinbach G., Garab G. (2008). The three-dimensional network of the thylakoid membranes in plants: quasihelical model of the granum-stroma assembly. Plant Cell. 20 (10), 2552–2557. doi: 10.1105/tpc.108.059147
Nevo R., Chuartzman S.G., Tsabari O., Reich Z., Charuvi D., Shimoni E. (2009). “Architecture of thylakoid membrane networks,” in Lipids in Photosynthesis, vol. 30 . Eds. Wada H., Murata N. (Dordrecht: Springer), 295–328.
Nicol L., Croce R. (2021). The PsbS protein and low pH are necessary and sufficient to induce quenching in the light-harvesting complex of plants LHCII. Sci. Rep. 11 (1), 7415. doi: 10.1038/s41598-021-86975-9
Paolillo D. J. Jr. (1970). The three-dimensional arrangement of intergranal lamellae in chloroplasts. J. Cell Sci. 6 (1), 243–255. doi: 10.1242/jcs.6.1.243
Peltier J. B., Emanuelsson O., Kalume D. E., Ytterberg J., Friso G., Rudella A., et al. (2002). Central functions of the lumenal and peripheral thylakoid proteome of Arabidopsis determined by experimentation and genome-wide prediction. Plant Cell. 14 (1), 211–236. doi: 10.1105/tpc.010304
Pérez-Bueno M. L., Rahoutei J., Sajnani C., García-Luque I., Barón M. (2004). Proteomic analysis of the oxygen-evolving complex of photosystem II under biotec stress: studies on Nicotiana benthamiana infected with tobamoviruses. Proteomics 4 (2), 418–425. doi: 10.1002/pmic.200300655
Pesaresi P., Scharfenberg M., Weigel M., Granlund I., Schröder W. P., Finazzi G., et al. (2009). Mutants, overexpressors, and interactors of Arabidopsis plastocyanin isoforms: revised roles of plastocyanin in photosynthetic electron flow and thylakoid redox state. Mol. Plant 2 (2), 236–248. doi: 10.1093/mp/ssn041
Petersson U. A., Kieselbach T., García-Cerdán J. G., Schröder W. P. (2006). The Prx Q protein of Arabidopsis thaliana is a member of the luminal chloroplast proteome. FEBS Lett. 580 (26), 6055–6061. doi: 10.1016/j.febslet.2006.10.001
Plücken H., Müller B., Grohmann D., Westhoff P., Eichacker L. A. (2002). The HCF136 protein is essential for assembly of the photosystem II reaction center in Arabidopsis thaliana. FEBS Lett. 532 (1-2), 85–90. doi: 10.1016/s0014-5793(02)03634-7
Roose J. L., Frankel L. K., Mummadisetti M. P., et al. (2016). The extrinsic proteins of photosystem II: update. Planta 243, 889–908. doi: 10.1007/s00425-015-2462-6
Schubert M., Petersson U. A., Haas B. J., Funk C., Schröder W. P., Kieselbach T. (2002). Proteome map of the chloroplast lumen of Arabidopsis thaliana. J. Biol. Chem. 277 (10), 8354–8365. doi: 10.1074/jbc.M108575200
Schuhmann H., Adamska I. (2012). Deg proteases and their role in protein quality control and processing in different subcellular compartments of the plant cell. Physiol. Plant 145 (1), 224–234. doi: 10.1111/j.1399-3054.2011.01533.x
Simionato D., Basso S., Zaffagnini M., Lana T., Marzotto F., Trost P., et al. (2015). Protein redox regulation in the thylakoid lumen: the importance of disulfide bonds for violaxanthin de-epoxidase. FEBS Lett. 589 (8), 919–923. doi: 10.1016/j.febslet.2015.02.033
Spetea C. (2012). “Role of chloroplast thylakoid lumen in photosynthetic regulation and plant cell signaling,” in Progress in Botany, vol. 73 . Eds. Lüttge U., Beyschlag W., Büdel B., Francis D. (Berlin, Heidelberg: Springer). doi: 10.1007/978-3-642-22746-2_8
Staehelin L. A., Paolillo D. J. (2020). A brief history of how microscopic studies led to the elucidation of the 3D architecture and macromolecular organization of higher plant thylakoids. Photosynth. Res. 145 (3), 237–258. doi: 10.1007/s11120-020-00782-3
Varadi M., Anyango S., Deshpande M., Nair S., Natassia C., Yordanova G., et al. (2022). AlphaFold Protein Structure Database: massively expanding the structural coverage of protein-sequence space with high-accuracy models. Nucleic Acids Res. 50 (D1), D439–D444. doi: 10.1093/nar/gkab1061
Vasudevan D., Fu A., Luan S., Swaminathan K. (2012). Crystal structure of Arabidopsis cyclophilin38 reveals a previously uncharacterized immunophilin fold and a possible autoinhibitory mechanism. Plant Cell. 24 (6), 2666–2674. doi: 10.1105/tpc.111.093781
Weibull C., Alertsson P.-Å. (1988). Ultrastructure of spinach thylakoids as seen in low-temperature and conventional embeddings. J. Ultrastruct. Mol. Struct. Res. 100, 55–59. doi: 10.1016/0889-1605(88)90058-4
Weigel M., Varotto C., Pesaresi P., Finazzi G., Rappaport F., Salamini F., et al. (2003). Plastocyanin is indispensable for photosynthetic electron flow in Arabidopsis thaliana. J. Biol. Chem. 278 (33), 31286–31289. doi: 10.1074/jbc.M302876200
Yabuta S., Ifuku K., Takabayashi A., Ishihara S., Ido K., Ishikawa N., et al. (2010). Three PsbQ-like proteins are required for the function of the chloroplast NAD(P)H dehydrogenase complex in Arabidopsis. Plant Cell Physiol. 51 (6), 866–876. doi: 10.1093/pcp/pcq060
Yu J., Knoppová J., Michoux F., Bialek W., Cota E., Shukla M. K., et al. (2018). Ycf48 involved in the biogenesis of the oxygen-evolving photosystem II complex is a seven-bladed beta-propeller protein. Proc. Natl. Acad. Sci. U. S. A. 115 (33), E7824–E7833. doi: 10.1073/pnas.1800609115
Zhu W., Xu L., Yu X., Zhong Y. (2022). The immunophilin CYCLOPHILIN28 affects PSII-LHCII supercomplex assembly and accumulation in Arabidopsis thaliana. J. Integr. Plant Biol. 64 (4), 915–929. doi: 10.1111/jipb.13235
Keywords: photosystem II, proteases, immunophillins, water oxidation, grana
Citation: Farci D and Schröder WP (2023) Thylakoid Lumen; from “proton bag” to photosynthetic functionally important compartment. Front. Plant Physiol. 1:1310167. doi: 10.3389/fphgy.2023.1310167
Received: 09 October 2023; Accepted: 20 November 2023;
Published: 11 December 2023.
Edited by:
Giles Nicholas Johnson, The University of Manchester, United KingdomReviewed by:
Wu Xu, University of Louisiana at Lafayette, United StatesRalf Bernd Klösgen, Martin Luther University of Halle-Wittenberg, Germany
Copyright © 2023 Farci and Schröder. This is an open-access article distributed under the terms of the Creative Commons Attribution License (CC BY). The use, distribution or reproduction in other forums is permitted, provided the original author(s) and the copyright owner(s) are credited and that the original publication in this journal is cited, in accordance with accepted academic practice. No use, distribution or reproduction is permitted which does not comply with these terms.
*Correspondence: Wolfgang P. Schröder, d29sZmdhbmcuc2Nocm9kZXJAdW11LnNl