- 1State Key Laboratory of Subtropical Silviculture, Zhejiang A&F University, Hangzhou, China
- 2Key Laboratory of Modern Silvicultural Technology of Zhejiang Province, Hangzhou, China
- 3State Forestry and Grassland Administration (SFGA) Research Center for Torreya Grandis, Zhejiang A&F University, Hangzhou, China
- 4Department of Forest Genetics and Plant Physiology, Umea Plant Science Center, Sveriges Lantbruksuniversitet (SLU), Umeå, Sweden
In autumn, the buds of extratropical trees are in a state of endodormancy, since regardless of the prevailing environmental conditions, growth cannot be activated in these buds because the dormancy is caused by physiological factors in the buds. In natural conditions the growth-arresting physiological factors are removed by prolonged exposure to low chilling temperatures. This phenomenon is a key adaptive trait, for it prevents ‘false spring’, i.e., untimely bud burst during mild spells in autumn and winter, which would lead to cold damage during subsequent cold periods. Traditionally, endodormancy and the chilling requirement have been important in practical horticulture, as cultivars with low and high chilling requirements have been bred for locations in warm and cool climates, respectively. More recently, endodormancy and the chilling requirement have become major research themes in climate change studies where climatic change impacts are assessed by means of process-based tree phenology models. The dormancy phenomenon has been studied thoroughly at the whole-tree level for a hundred years, and several genes and genetic pathways involved have recently been identified in tree species such as hybrid aspen, apple, and pear. There is an urgent need, however, to integrate molecular physiological studies with modelling studies so as to understand the impact of climate change on the regulation of dormancy. To that end, we shall provide an overview of bud endodormancy research.
Introduction
Buds of extratropical trees can be dormant for various reasons. On the basis of the factor causing the prevention of bud burst, three types of bud dormancy are often discerned (Fuchigami et al., 1982; Hänninen, 2016). First, in winter and early spring the buds remain dormant due to unfavourable environmental conditions, typically low air temperatures. This dormancy category is called ecodormancy (Lang et al., 1987), synonymous to quiescence (Fuchigami et al., 1982). Thus, while an ecodormant bud is not growing, being dormant from the perspective of growth, it has acquired the competence to grow and therefore has the potential to undergo bud burst. The onset of subsequent growth, however, is suppressed by unfavourable environmental factors. Second, in late summer, buds in less apical positions are often prevented from bud burst by hormonal regulation caused by the more apical buds. This phenomenon is referred to as paradormancy (Lang et al., 1987). Third, in autumn and early winter the buds of extratropical trees remain dormant due to physiological conditions inside the bud. This phenomenon is referred to as endodormancy (Lang et al., 1987), synonymous to rest (Fuchigami et al., 1982). Thus the major difference between the endo- and ecodormant bud is related to the competence to grow, which is lacking in endodormant but present in ecodormant buds. As a rule, the growth-arresting conditions are removed by prolonged exposure to low chilling temperatures, although other drivers, most importantly photoperiod, may also have an effect (Basler and Körner, 2012; Cooke et al., 2012; Vitasse and Basler, 2013; Singh et al., 2017; but see also Zohner et al., 2016).
The phenomena of endodormancy and the chilling requirement of its release have been known for more than a hundred years (Coville, 1920), but they are now more topical than ever before. Endodormancy and the chilling requirement prevent an untimely bud burst and growth onset in autumn and winter (‘false spring’), and for that reason they combine to form a key adaptive trait, which synchronizes the annual cycle of extratropical trees with the seasonal climate prevailing at the native growing sites of these trees (Sarvas, 1974; Murray et al., 1989; Hänninen, 2016). Whether or not this synchronization will also be retained under the climatic warming is a topical scientific question with important ecological, environmental, and economic implications (Vitasse et al., 2011; Chuine et al., 2016; Zhang H. et al., 2022). In recent decades, considerable insight into the genetic pathways regulating endodormancy and its release has been gained through studies of model trees such as Populus, apple and pear. In this minireview we briefly summarize how the research on endodormancy in buds of extratropical trees has evolved into its current state of the art, reaching into the molecular depths of plant life at one end of its spectrum and into the large-scale global ecological issues at the other.
‘Chilled to be forced’ – the classical black-box approach to endodormancy
The quotation in the section title, taken from the title of Baumgarten et al. (2021), describes the classical approach to endodormancy and its release (Coville, 1920). The existence of endodormancy and the chilling requirement are inferred from the observed change in the response of the plants to growth-promoting high-temperature forcing conditions caused by prolonged exposure to chilling conditions before the regrowth test in the forcing conditions. With only short periods of chilling or none at all, there is either no bud burst seen or the bud burst takes an exceptionally long period of forcing (= a high value of Days to Bud Burst, DBB). With successively longer durations of the chilling treatment, the Bud Burst Percentage, BB%, increases and/or the DBB decreases in the forcing conditions. The chilling requirement, Δt, i.e., the duration of chilling required for endodormancy release, is inferred from the BB% and/or DBB curves plotted against the duration of chilling (Hänninen et al., 2019).
In some studies, only the BB% has been addressed (Erez and Lavee, 1971; Erez and Couvillon, 1987). In some other studies, only the DBB has been looked at (Myking and Heide, 1995). In those studies, the criterion for the Δt has mostly been set on the basis of the levelling off of the decline of the DBB curve with increased duration of chilling: the chilling requirement is considered to have been met when additional chilling does not cause considerable reduction of the DBB any longer (Worrall and Mergen, 1967). Sarvas (1974) determined the chilling requirement on the basis of the BB% only. However, he used a relatively short forcing time (21 days), thus excluding all potential bud burst observations with DBB > 21days. In this way, DBB was implicitly addressed in Sarvas (1974) method also. Recently, Zhang R. et al. (2022, Zhang et al., 2023) set a criterion for both BB% and DBB, thus obtaining two candidate values for Δt. As both criteria are required to be met in their method, the final value of the chilling requirement is determined as equal to the higher one of the two obtained candidate values of Δt. Nevertheless, whichever definition of the Δt is used, the classical approach described above is essentially a black-box one, for it makes use of no observations or measurements of the growth-arresting physiological and molecular conditions or their removal inside the buds per se. In other words, the invisible endodormancy release that has taken place in the buds under the chilling conditions by the time of the transfer to the forcing conditions is determined on the basis of the implications of the endodormancy release for the occurrence and timing of the visible bud burst in the forcing conditions.
In most chilling-forcing experiments, the chilling treatments have been conducted either outdoors under naturally fluctuating autumn and winter temperature conditions or in growth chambers under one constant temperature. These methods have been helpful for testing the existence of endodormancy (Zhang et al., 2021) as well as comparing the chilling requirements of tree species, provenances and cultivars (Leinonen, 1996a; Gao et al., 2012), and especially for identifying the components of the genetic pathways and molecular events (see below) that regulate the dormancy release and facilitate bud burst. Comparisons of chilling requirements have been especially important for breeding horticultural crop cultivars: cultivars with a low chilling requirement are needed in geographical locations with mild winters, whereas cultivars with a higher chilling requirement are suitable for cooler climates (Erez, 2000).
Process-based phenology modelling – quantifying the effects of chilling in global change studies
Since the 1970s, endodormancy and chilling have been addressed in process-based models of tree spring phenology (Chuine et al., 2013; Hänninen, 2016). In addition to effects of chilling on endodormancy release, these models also address the effects of high temperatures on ecodormancy release, thus predicting the timing of spring phenological events such as leaf-out of leaf buds and the flowering of flower buds (Figure 1). Today, process-based tree spring phenology models are major tools in global change studies where the ecological effects of climatic change are evaluated (Wang et al., 2020; Zheng et al., 2021). This is because changes in phenology have several important implications at the ecosystem level, such as changes in the cycling of nutrients, carbon, and water, or changes in the trees’ relationships with herbivores (Kramer and Hänninen, 2009; Richardson et al., 2009; Senior et al., 2020).
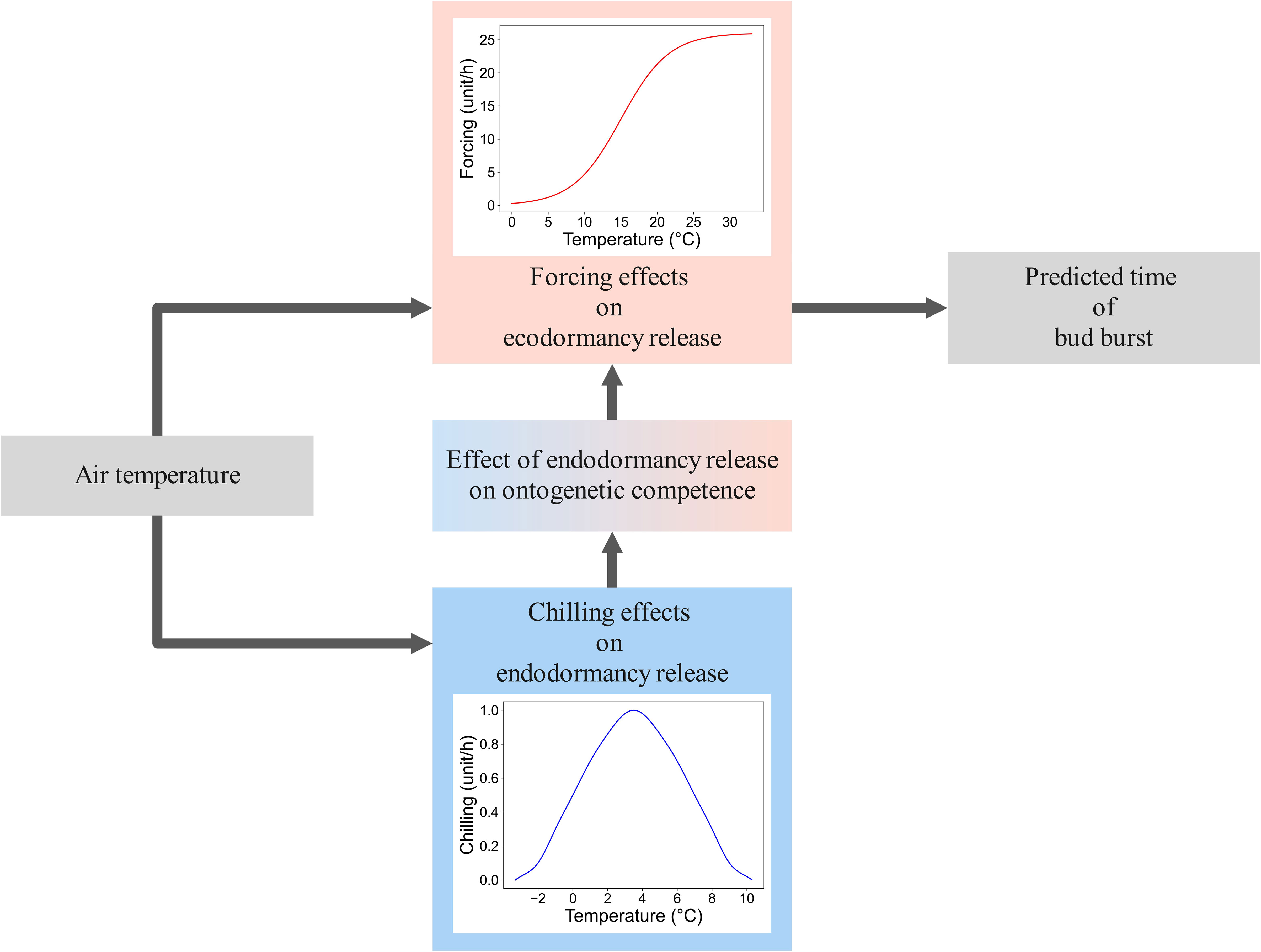
Figure 1 Rationale of the process-based tree spring phenology models. The models address both the chilling effects of endodormancy release (the blue bell-shaped air temperature response at the bottom of the figure) and the forcing effects (effects of high temperatures) on ecodormancy release (the red sigmoidal air temperature response at the top of the figure). The predicted status of endodormancy release affects the rate of ecodormancy release: in the absence of sufficient accumulated chilling, ecodormancy release either does not progress at all or progresses at a lower rate than predicted on the basis of the sigmoidal forcing temperature response alone. In the Hänninen-Kramer (HK) modelling framework (Hänninen, 1990; Kramer, 1994a; Kramer, 1994b; Hänninen and Kramer, 2007; Hänninen, 2016; Zhang et al., 2023), an explicit sub-model is introduced for a theoretical variable ‘ontogenetic competence’, Co, for transferring the growth-arresting effects of endodormancy on ecodormancy release (the small box in the middle of the figure).
In process-based models, the effects of chilling on endodormancy release have been addressed by examining the accumulation of chilling units (Chuine et al., 2013) or, to use a less well-known but physiologically more accurate wording, by calculating the rate and state of the endodormancy release (synonymous to the rate and state of rest break, Hänninen, 2016). Various rules, fixed a priori, have often been applied to the response. In the simplest case, i.e., the threshold model, it has been assumed that all temperatures below a given threshold, typically +5°C, are equally effective in endodormancy release (Cannell and Smith, 1983). So, at any hour with the air temperature below +5°C (or on any day with the daily mean temperature below +5°C), one chilling unit (CU) is accumulated. This quantifies the momentary (hourly, daily) rate of endodormancy release. The state of endodormancy release at the end of any given hour (day) is obtained by means of numerical integration, i.e., by summing the hourly (daily) rate values (Chuine et al., 2013; Hänninen, 2016). Endodormancy is predicted to be released when the accumulated CU sum attains a given critical genotype-specific value. In addition to the threshold model, some other a priori fixed forms of the chilling response have been used in process-based spring phenology models. These include the range model, where chilling units are accumulated at a constant rate in the temperature range between a lower and an upper threshold temperature (Lin et al., 2022), and the triangular model, where the response is bell-shaped between the lower and the upper threshold temperatures (Sarvas, 1974; Figure 1).
The technique for experimentally determining the chilling response of endodormancy release is straightforward: the chilling-forcing experiment described in the previous section is repeated in several constant chilling temperatures (Sarvas, 1974). In that way the chilling requirement, Δt, is obtained for several temperatures, and the rate of endodormancy release (rate of chill accumulation) is obtained for each temperature as the reciprocal of Δt: the less time is needed for endodormancy release, the higher the rate of endodormancy release. Lastly, the obtained values of the rate of endodormancy release are plotted against the experimental temperature applied (Sarvas, 1974; Campbell and Sugano, 1975; Hänninen et al., 2019; Zhang R. et al. 2022, Zhang et al., 2023). However, though the experimental technique for determining the chilling response of endodormancy release is straightforward, it is laborious and time-consuming. This is evidently the reason why the chilling response of endodormancy has usually not been determined experimentally. Rather, the response has mostly been determined either a priori (Cannell and Smith, 1983; Murray et al., 1989), or by means of inverse modelling, meaning that the values of all model parameters (not only the ones related to chilling effects) are estimated by fitting the model statistically to observational long-term phenological and air temperature records (Kramer, 1994a; Kramer, 1994b; Chuine et al., 1998; Wang et al., 2020; Luedeling et al., 2021, Zhang H. et al., 2022). In the absence of explicit experimental data, the use of inverse modelling has been seen as necessary even though it is known to be prone to produce biologically unrealistic models (Hänninen et al., 2019).
Genes and blocked plasmodesmata – opening the black box
In recent years, considerable progress has been made in identifying genetic components that regulate dormancy, its release, and bud burst (Figure 2; Singh et al., 2017; Singh et al., 2018; Tylewicz et al., 2018; Azeez et al., 2021; Falavigna et al., 2021; Yang et al., 2021; Yang et al., 2023). By using model trees such as Populus, apple, and pear, time-course analysis of global gene expression has been able to identify patterns of genes whose expression in the buds changes during the transition from dormancy to dormancy release and subsequent bud burst (Vimont et al., 2020). These analyses, combined with proteomic, metabolic and hormonal analyses of buds, have led to identification of hundreds of genes, metabolites and hormones whose change is temporally associated with the transition from endodormancy to ecodormancy change and bud burst (Beauvieux et al., 2019). Only in a few cases, however, has any functional analysis been performed to check whether such changes, may they be in gene expression or hormonal levels, actually contribute functionally to the release of dormancy or to bud burst or whether they represent processes that could be unrelated to dormancy but simply share a common temporal regulation. As a result, conclusions regarding the role of specific genes or hormones in phenology, unless and until functionally validated, should be treated with caution. However, on the basis of functional data using gain and loss of function approaches, the role of plant hormone ABA in dormancy regulation is now well established, with ABA response being essential for short-day-induced bud dormancy (Tylewicz et al., 2018; Yang et al., 2023). Moreover, transcription factors acting downstream of ABA, e.g., tree orthologs of SVP (SHORT VEGETATIVE PHASE), a floral repressor, have been implicated in these processes in several species (Singh et al., 2018; Falavigna et al., 2021). Downstream of ABA-SVP, considerable attention has been paid to the dynamic control of plasmodesmata (PDs), the intercellular channels, by hormonal and environmental factors. Closure of PDs is associated with and essential for the establishment of dormancy, whereas their opening is associated with dormancy release in a number of tree species, e.g., populus, birch, and peach (Rinne and van der Schoot, 2003; Rinne et al, 2011; Tylewicz et al., 2018; Singh et al., 2019; Zhao et al., 2023). The dynamic control of PDs with closure during dormancy prevents the movement of growth regulators, e.g., tree orthologs of FT (FLOWERING LOCUS T), to the shoot apical meristem, whereas their opening restores intercellular communication to induce competence to respond to these growth promoters such as FT, but possibly also GA (Rinne et al., 2011). Additionally, dormancy and its release are associated with massive change in the gene expression, and these transcriptional changes may be enabled by chromatin-based master regulators acting on global scales, as shown earlier for poplar, cherry, and kiwifruit (Conde et al., 2017; Voogd et al., 2022).
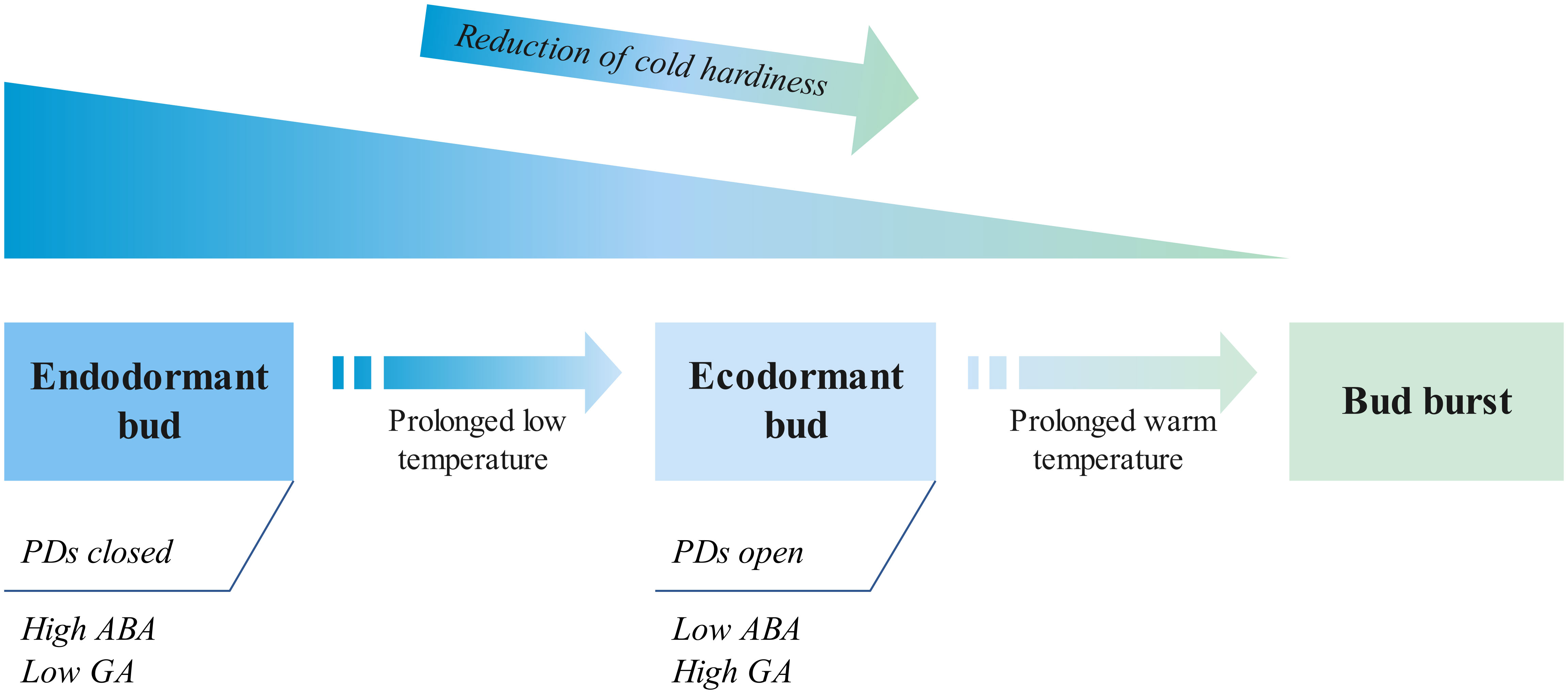
Figure 2 A schematic representation of key molecular events during the switch from the endodormant to the ecodormant state induced by prolonged exposure to low temperature and the subsequent bud burst induced by progressively warmer temperatures in the spring. Plasmodesmata (PDs) are closed in endodormant buds, and a prolonged low-temperature treatment presumably reduces the abscissic acid (ABA) levels (and/or sensitivity to ABA) and increases the Gibberellic acid (GA) levels, which could contribute to the switch to ecodormancy. Simultaneously, there is a reduction of cold hardiness prior to budburst. This description of the key events is not exhaustive, and more details can be found in the reviews by Singh et al. (2017) and Yang et al. (2021).
Endodormancy and other phenomena of the annual cycle – relationships to cold hardiness
As explained in the preceding sections, endodormancy is defined by observations of bud burst at the whole-tree level, but the phenomenon has its roots in other phenomena occurring at the cellular and molecular levels. For this reason, it is no wonder that endodormancy is related to other whole-tree phenomena of the annual cycle, especially to the cold hardiness of trees (Hänninen, 2016), which may partly share their physiological and molecular basis with endodormancy. Numerous empirical studies over several decades have shown that the air temperature response of acclimation/deacclimation changes during dormancy and subsequent bud burst (Edgerton, 1954; Proebsting, 1963). For instance, a given air temperature causing acclimation at an early phase of endodormancy may even cause deacclimation at a later phase (Leinonen et al., 1995). This phenomenon has been addressed for several decades in integrated cold hardiness models using a process-based spring phenology model as a sub-model (Winter, 1973; Kobayashi et al., 1983; Kellomäki et al., 1992; Kellomäki et al., 1995; Leinonen, 1996b; Charrier et al., 2018). In those models, the dormancy phase is used for explaining the changes of the responses of acclimation and deacclimation to environmental factors, most importantly the air temperature and photoperiod.
Recently, Kovaleski (2022) introduced an alternative to the prevalent approach of the integrated cold hardiness models in his spring phenology model by modelling the prevailing cold hardiness first. Cold hardiness is then taken for a measure of the ecodormancy status, and bud burst is predicted to occur when the cold hardiness attains the species-specific threshold value corresponding to bud burst. Together with the previous integrated cold hardiness models, Kovaleski (2022) study shows that the two whole-tree traits of dormancy status and cold hardiness correlate with each other. This suggests that they could share the same physiological and molecular basis, at least partially. Without detailed molecular studies, however, it is impossible to tell if one of the traits is the cause and the other the effect of if they are just simultaneous manifestations of the basic physiological and molecular phenomena.
Most importantly, Kovaleski (2022) showed convincingly that trees with different phylogenetic backgrounds and different cold hardiness dynamics share an unexpected common feature: in all of them, the maximum rate of deacclimation (loss of cold hardiness) is attained with approximately the same amount of accumulated chilling. This is a major novel finding, if not a breakthrough. In addition to this intriguing finding, Kovaleski (2022) further showed that the traditional bud burst-related chilling requirement varies considerably between the species examined, thus confirming earlier findings from several decades of research.
While Kovaleski (2022) experimental results provide important new insight into the seasonality phenomena examined, his subsequent inferences stemming from them raise several questions. In particular, he states that (i) a cold hardiness-related chilling requirement would provide a more realistic picture of the true dormancy progression than the classical bud burst-related chilling requirement and (ii) because the cold hardiness-related chilling requirement is similar in different species, the dormancy progression, too, is similar. As reviewed above, endodormancy is defined with reference to the occurrence – or lack of it – of bud burst under growth-promoting conditions. The fact that Kovaleski (2022) observed more regularity in the relationship of chilling with cold hardiness than with bud burst opens up new insights into the seasonality traits of trees, but in our view that does not warrant a change of the meaning of an established scientific concept. Furthermore, what exactly does Kovaleski (2022) mean by stating that dormancy progression is similar in all trees? For instance, how is that notion related to the traditional concepts of low-chill and high-chill cultivars, which are crucial for practical horticulture (Erez, 2000)? If dormancy progression is similar in both types of cultivars, what is the difference between them?
Discussion
In the research on bud endodormancy and the chilling requirement in extratropical trees, two quite different schools have been involved. On the one hand there is the black-box whole-tree school, including the process-based modelling approach, and on the other hand, the mechanistic physiological and molecular school. The two schools have worked largely in isolation from each other, even though the need for more co-operation has been emphasised over the years. Deeper considerations of the mechanistic basis of the dormancy phenomena would help the whole-tree modellers to improve the biological realism of the whole-tree phenological models by revealing the true causal effects of environmental factors and the causal relationships between the processes modelled. To that end, genetic approaches have identified relevant genes, metabolites and hormones and spatio-temporal changes in the expression of genes, or metabolites, associated with dormancy regulation. Yet this information, which is essentially quantitative, has not really been sufficiently integrated into process-based modelling so far. By integrating such quantitative molecular information, which provides mechanistic insight into dormancy and bud burst, with modelling, one could delineate the effects of factors such as day length and temperature as well as their interactions in the regulation of phenological traits. Furthermore, such linking of the two research traditions would be fruitful not only for process-based phenological modelling but also for the study of the molecular mechanisms of dormancy, cold hardiness and phenology. This is because without links to the operational models used in assessments of the climate change impact, the impact of detailed molecular laboratory studies on global change studies would remain rather limited.
Other types of mathematical phenology models have been successfully combined with molecular research (Izawa, 2015; Yeoh et al., 2017; Vimont et al., 2019; Hu et al., 2021; Vimont et al., 2021; Satake et al., 2022), suggesting that this should also be possible in the process-based tree phenology models discussed in this minireview. A major impediment to such attempts is the need to translate the mechanistic findings obtained with microscopy and molecular analyses into the environmental responses included in the whole-tree models. The Hänninen-Kramer (HK) framework uses the concept and model variable ‘ontogenetic competence’ to summarize the growth-arresting physiological and molecular phenomena into one variable with a value ranging from 0 (absolute endodormancy, no ontogenetic development towards bud burst under any conditions) to 1 (endodormancy fully released, ecodormancy attained, ontogenetic development towards bud burst at the full rate, regulated only by the ambient temperature) (Hänninen, 1990; Kramer, 1994a; Kramer, 1994b; Hänninen and Kramer, 2007; Hänninen, 2016; Zhang et al., 2023; Figure 1). On the basis of this reasoning, we suggest HK modelling as a potential framework (although others could also be used) for integrating the two approaches to bud endodormancy research. We know, for instance, that the opening of plasmodesmata is associated with dormancy release and that this opening shows a quantitative relationship with low temperatures. Thus the opening of plasmodesmata and its links with dormancy release could be linked to modelling by integrating them into the value assigned to the ontogenetic competence. Similarly, it would be possible to address gene expression, which varies quantitatively with dormancy release (or bud burst), by making use of the model variable ontogenetic competence.
Author contributions
RB: Conceptualization, Writing – original draft, Writing – review & editing. RZ: Conceptualization, Writing – original draft, Writing – review & editing. HH: Conceptualization, Writing – original draft, Writing – review & editing. JW: Conceptualization, Writing – original draft, Writing – review & editing.
Funding
The authors declare financial support was received for the research, authorship, and/or publication of this article. The study was financed by the Chinese National Natural Science Foundation (32171832), the National Forestry and Grassland Technological Innovation Program for Young TopNotch Talents (2020132604), the Youth Elite Science Sponsorship Program of CAST (YESS, 2020QNRC001), and Overseas Expertise Introduction Project for Discipline Innovation (111 Project D18008).
Acknowledgments
We thank Yu Zhao for drawing the figures, and Pekka Hirvonen (www.toisinsanoen.eu) for revising the language of the manuscript.
Conflict of interest
The authors declare that the research was conducted in the absence of any commercial or financial relationships that could be construed as a potential conflict of interest.
The author(s) JW, RZ, RB declared that they were an editorial board member of Frontiers, at the time of submission. This had no impact on the peer review process and the final decision.
Publisher’s note
All claims expressed in this article are solely those of the authors and do not necessarily represent those of their affiliated organizations, or those of the publisher, the editors and the reviewers. Any product that may be evaluated in this article, or claim that may be made by its manufacturer, is not guaranteed or endorsed by the publisher.
References
Azeez A., Zhao Y. C., Singh R. K., Yordanov Y., Dash M., Misckolzi P., et al. (2021). The EARLY BUD-BREAK regulatory module in Populus. Nat. Com. 12, 1123. doi: 10.1038/s41467-021-21449-0
Basler D., Körner Ch. (2012). Photoperiod sensitivity of bud burst in 14 temperate forest tree species. Agric. For. Meteorol. 165, 73–81. doi: 10.1016/j.agrformet.2012.06.001
Baumgarten F., Zohner C. M., Gessler A., Vitasse Y. (2021). Chilled to be forced: the best dose to wake up buds from winter dormancy. New Phytol. 230, 1366–1377. doi: 10.1111/nph.17270
Beauvieux R., Wenden B., Ballias P., Christmann H., Gibon Y., Dirlewanger E. (2019). Dynamics of bud metabolites during dormancy in sweet cherry genotypes with contrasted chilling requirements. Acta Hortic. 1235, 407–412. doi: 10.17660/ActaHortic.2019.1235.56
Campbell R. K., Sugano A. I. (1975). Phenology of bud burst in Douglas-fir related to provenance, photoperiod, chilling, and flushing temperature. Bot. Gaz. 136, 290–298. doi: 10.1086/336817
Cannell M. G. R., Smith R. I. (1983). Thermal time, chill days and prediction of budburst in Picea sitchensis. J. Appl. Ecol. 20, 951–963. doi: 10.2307/2403139
Charrier G., Chuine I., Bonhomme M., Thierry A. (2018). Assessing frost damages using dynamic models in walnut trees: exposure rather than vulnerability controls frost risks. Plant Cell Envir. 41, 1008–1021. doi: 10.1111/pce.12935
Chuine I., Bonhomme M., Legave J.-M., de Cortázar-Atauri I. G., Charrier G., Lacointe A. (2016). Can phenological models predict tree phenology accurately in the future? The unrevealed hurdle of endodormancy break. Glob. Change Biol. 22, 3444–3460. doi: 10.1111/gcb.13383
Chuine I., Cour P., Rousseau D. D. (1998). Fitting models predicting dates of flowering of temperate-zone trees using simulated annealing. Plant Cell Envir. 21, 455–466. doi: 10.1046/j.1365-3040.1998.00299.x
Chuine I., de Cortazar-Atauri I. G., Kramer K., Hänninen H. (2013). “Plant development models,” in Phenology: An Integrative Environmental Science., 2nd ed. Ed. Schwartz M. D. (Dordrecht: Springer Science+Business Media B.V), 275–293.
Conde D., Le Gac A. L., Perales M., Dervinis C., Kirst M., Maury S., et al. (2017). Chilling-responsive DEMETER-LIKE DNA demethylase mediates in poplar bud break. Plant Cell Environ. 10, 2236–2249. doi: 10.1111/pce.13019
Cooke J. E. K., Eriksson M. E., Junttila. O. (2012). The dynamic nature of bud dormancy in trees: environmental control and molecular mechanisms. Plant Cell Envir. 35, 1707–1728. doi: 10.1111/j.1365-3040.2012.02552.x
Coville F. V. (1920). The influence of cold in stimulating the growth of plants. J. Agric. Res. 20, 151–160.
Edgerton L. J. (1954). Fluctuations in the cold hardiness of peach flower buds during rest period and dormancy. Proc. Am. Soc. Hortic. Sci. 64, 175–180.
Erez A. (2000). “Bud dormancy: phenomenon, problems and solutions in the tropics and subtropics,” in Temperate Fruit Trees in Warm Climates. Ed. Erez A. (Alphen aan den Rijn: Kluwer Academic Publishers), 17–48.
Erez A., Couvillon G. A. (1987). Characterization of the influence of moderate temperatures on rest completion in peach. J. Am. Soc. Hortic. Sci. 112, 677–680. doi: 10.21273/JASHS.112.4.677
Erez A., Lavee S. (1971). The effect of climatic conditions on dormancy development of peach buds. I. Temperature. J. Am. Soc. Hortic. Sci. 96, 711–714. doi: 10.21273/JASHS.96.6.711
Falavigna V., Severing E., Lai X., Estevan J., Farrera I., Hugouviex V., et al. (2021). Unraveling the role of MADS transcription factor complexes in apple tree dormancy. New Phytol. 232, 2071–2088. doi: 10.1111/nph.17710
Fuchigami L. H., Weiser C. J., Kobayashi K., Timmis R., Gusta L. V. (1982). “A degree growth stage (°GS) model and cold acclimation in temperate woody plants,” in Plant Cold Hardiness and Freezing Stress. Mechanisms and Crop Implications., vol. 2 . Eds. Li P. H., Sakai A. (New York, NY: Academic Press), 93–116.
Gao Z., Zhuang W., Wang L., Shao J., Luo X., Cai B., et al. (2012). Evaluation of chilling and heat requirements in Japanese apricot with three models. HortScience 47, 1826–1831. doi: 10.21273/HORTSCI.47.12.1826
Hänninen H. (1990). Modelling bud dormancy release in trees from cool and temperate regions. Acta For. Fenn. 213, 1–47. doi: 10.14214/aff.7660
Hänninen H. (2016). Boreal and temperate trees in a changing climate: Modelling the ecophysiology of seasonality (Dordrecht: Springer Science+Business Media).
Hänninen H., Kramer K. (2007). A framework for modelling the annual cycle of trees in boreal and temperate regions. Silva Fenn. 41, 167–205. doi: 10.14214/sf.313
Hänninen H., Kramer K., Tanino K., Zhang R., Wu J., Fu Y. H. (2019). Experiments are necessary in process-based tree phenology modelling. Trends Plant Sci. 24, 199–209. doi: 10.1016/j.tplants.2018.11.006
Hu P., Chapman S. C., Dreisigacker S., Sukumaran S., Reynolds M., Zheng B. (2021). Using a gene-based phenology model to identify optimal flowering periods of spring wheat in irrigated mega-environments. J. Exp. Bot. 72, 7203–7218. doi: 10.1093/jxb/erab326
Izawa T. (2015). Deciphering and prediction of plant dynamics under field conditions. Curr. Opin. Plant Biol. 24, 87–92. doi: 10.1016/j.pbi.2015.02.003
Kellomäki S., Hänninen H., Kolström M. (1995). Computations on frost damage to Scots pine under climatic warming in boreal conditions. Ecol. Appl. 5, 42–52. doi: 10.2307/1942050
Kellomäki S., Väisänen H., Hänninen H., Kolström T., Lauhanen R., Mattila U., et al. (1992). A simulation model for the succession of the boreal forest ecosystem. Silva Fenn. 26, 1–18. doi: 10.14214/sf.a15626
Kobayashi K. D., Fuchigami L. H., Weiser C. J. (1983). Modeling cold hardiness of red-osier dogwood. J. Am. Soc. Hortic. Sci. 108, 376–381. doi: 10.21273/JASHS.108.3.376
Kovaleski A. P. (2022). Woody species do not differ in dormancy progression: Differences in time to budbreak due to forcing and cold hardiness. Proc. Natl. Acad. Sci. U.S.A. 119, e2112250119. doi: 10.1073/pnas.2112250119
Kramer K. (1994a). Selecting a model to predict the onset of growth of Fagus sylvatica. J. Appl. Ecol. 31, 172–181. doi: 10.2307/2404609
Kramer K. (1994b). A modelling analysis of the effects of climatic warming on the probability of spring frost damage to tree species in The Netherlands and Germany. Plant Cell Envir. 17, 367–377. doi: 10.1111/j.1365-3040.1994.tb00305.x
Kramer K., Hänninen H. (2009). “The annual cycle of development of trees and process-based modelling of growth to scale up from the tree to the stand,” in Phenology of ecosystem processes. Ed. Noormets A. (LLC: Springer Science+Business Media), 201–227.
Lang G. A., Early J. D., Martin G. C., Darnell R. L. (1987). Endo-, para-, and ecodormancy: physiological terminology and classification for dormancy research. HortScience 22, 371–377. doi: 10.21273/HORTSCI.22.3.371
Leinonen I. (1996a). Dependence of dormancy release on temperature in different origins of Pinus sylvestris and Betula pendula seedlings. Scand. J. For. Res. 11, 122–128. doi: 10.1080/02827589609382919
Leinonen I. (1996b). A simulation model for the annual frost hardiness and freeze damage of Scots pine. Ann. Bot. 78, 687–693. doi: 10.1006/anbo.1996.0178
Leinonen I., Repo T., Hänninen H., Burr K. E. (1995). A second-order dynamic model for the frost hardiness of trees. Ann. Bot. 76, 89–95. doi: 10.1006/anbo.1995.1082
Lin S., Wang H., Ge Q., Hu Z. (2022). Effects of chilling on heat requirement of spring phenology vary between years. Agric. For. Meteorol. 312, 108718. doi: 10.1016/j.agrformet.2021.108718
Luedeling E., Schiffers K., Fohrmann T., Urbach C. (2021). PhenoFlex – an integrated model to predict phenology in temperate fruit trees. Agric. For. Meteorol. 307, 108491. doi: 10.1016/j.agrformet.2021.108491
Murray M. B., Cannell M. G. R., Smith R. I. (1989). Date of budburst of fifteen tree species in Britain following climatic warming. J. Appl. Ecol. 26, 693–700. doi: 10.2307/2404093
Myking T., Heide O. M. (1995). Dormancy release and chilling requirement of buds of latitudinal ecotypes of Betula pendula and B. pubescens. Tree Physiol. 15, 697–704. doi: 10.1093/treephys/15.11.697
Proebsting E. L. (1963). The role of air temperatures and bud development in determining hardiness of dormant Elberta peach fruit buds. Proc. Am. Soc. Hortic. Sci. 83, 259–269.
Richardson A. D., Hollinger D. Y., Dail D. B., Lee J. T., Munger J. W., O'Keefe J. (2009). Influence of spring phenology on seasonal and annual carbon balance in two contrasting New England forests. Tree Physiol. 29, 321–331. doi: 10.1093/treephys/tpn040
Rinne P. L. H., van der Schoot C. (2003). Plasmodesmata at the crossroads between development, dormancy, and defence. Can. J. Bot. 81, 1182–1197. doi: 10.1139/b03-123
Rinne P., Welling A., Vahala J., Ripel L., Ruonala R., Kangasjärvi J., et al. (2011). Chilling of dormant buds hyperinduces FLOWERING LOCUS T and recruits GA-inducible 1,3-beta-glucanases to reopen signal conduits and release dormancy in Populus. Plant Cell 23, 130–146. doi: 10.1105/tpc.110.081307
Sarvas R. (1974). Investigations on the annual cycle of development of forest trees. II. Autumn dormancy and winter dormancy. Commun. Inst. For. Fenn. 84 (1), 1–101.
Satake A., Nagahama A., Sasaki E. (2022). A cross-scale approach to unravel the molecular basis of plant phenology in temperate and tropical climates. New Phytol. 233, 340–2353. doi: 10.1111/nph.17897
Senior V. L., Evans L. C., Leather S. R., Oliver T. H., Evans K. L. (2020). Phenological responses in a sycamore-aphid-parasitoid system and consequences for aphid population dynamics: A 20 year case study. Glob. Change Biol. 26, 2814–2828. doi: 10.1111/gcb.15015
Singh R., Misckolczi P., Maurya J. P., Azeez A., Tylewicz S., Busov V., et al. (2018). A genetic network mediating the control of bud break in hybrid aspen. Nat. Com. 9, 4173. doi: 10.1038/s41467-018-06696-y
Singh R., Misckolczi P., Maurya J. P., Bhalerao R. P. (2019). A tree ortholog SHORT VEGETATIVE PHASE floral repressor mediates photoperiodic control of bud dormancy. Curr. Biol. 29, 128–133. doi: 10.1016/j.cub.2018.11.006
Singh R., Svystun T., AlDahmash B., Jönsson A. M., Bhalerao R. (2017). Photoperiodic and temperature mediated control of phenology in trees - a molecular perspective. New Phytol. 213, 511–524. doi: 10.1111/nph.14346
Tylewicz S., Petterle A., Marttila S., Misckolzi P., Singh R., Immanen J., et al. (2018). Photoperiodic control of seasonal growth is mediated by ABA acting on cell-cell communication. Science 360, 212–215. doi: 10.1126/science.aan8576
Vimont N., Fouche M., Campoy J. A., Tong M., Arkoun M., Yvin J.-C., et al. (2019). From bud formation to flowering: transcriptomic state defines the cherry developmental phases of sweet cherry bud dormancy. BMC Genomics 20, 974. doi: 10.1186/s12864-019-6348-z
Vimont N., Quah F. X., Schöepfer D. G., Roudier F., Dirlewanger E., Wigge P., et al. (2020). ChIP-seq and RNA-seq for complex and low-abundance tree buds reveal chromatin and expression co-dynamics during sweet cherry bud dormancy. Tree Genet. Genomes 16, 9. doi: 10.1007/s11295-019-1395-9
Vimont N., Schwarzenberg A., Domijan M., Donkpegan A. S. L., Beauvieux R., de Dantec L., et al. (2021). Fine tuning of hormonal signaling is linked to dormancy status in sweet cherry flower buds. Tree Physiol. 41, 544–561. doi: 10.1093/treephys/tpaa122
Vitasse Y., Basler D. (2013). What role for photoperiod in the bud burst phenology of European beech. Eur. J. For. Res. 132, 1–8. doi: 10.1007/s10342-012-0661-2
Vitasse Y., François C., Delpierre N., Dufrêne E., Kremer A., Chuine I., et al. (2011). Assessing the effects of climate change on the phenology of European temperate trees. Agric. For. Meteorol. 151, 969–980. doi: 10.1016/j.agrformet.2011.03.003
Voogd C., Brian L., Wu R., Wang T., Allan A. C., Varkonyi-Gasic E. (2022). A MADS-box gene with similarity to FLC is induced by cold and correlated with epigenetic changes to control budbreak in kiwifruit. New Phytol. 233, 2111–2126. doi: 10.1111/nph.17916
Wang H., Wu C., Ciais P., Peñuelas J., Dai J., Fu Y., et al. (2020). Overestimation of the effect of climatic warming on spring phenology due to misrepresentation of chilling. Nat. Com. 11, 4945. doi: 10.1038/s41467-020-18743-8
Winter F. (1973). Ein Simulationsmodell über die Phänologie und den Verlauf der Frostresistenz von Apfelbäumen. Ecol. Plant 8, 141–152.
Worrall J., Mergen F. (1967). Environmental and genetic control of dormancy in Picea abies. Physiol. Plan. 20, 733–745. doi: 10.1111/j.1399-3054.1967.tb07217.x
Yang Q., Gao Y., Wu X., Moriguchi T., Bai S., Teng Y. (2021). Bud endodormancy in deciduous fruit trees: advances and prospects. Hortic. Res. 8, 139. doi: 10.1038/s41438-021-00575-2
Yang Q., Wu X., Gao Y., Ni J., Li J., Pei Z., et al. (2023). PpyABF3 recruits the COMPASS-like complex to regulate bud dormancy maintenance via integrating ABA signaling and GA catabolism. New Phytol. 237, 192–203. doi: 10.1111/nph.18508
Yeoh S. H., Satake A., Numata S., Ichie T., Lee S. L., Basherudin N., et al. (2017). Unravelling proximate cues of mass flowering in the tropical forests of South-East Asia from gene expression analyses. Mol. Ecol. 26, 5074–5085. doi: 10.1111/mec.14257
Zhang H., Chuine I., Regnier P., Ciais P., Yuan P. W. (2022). Deciphering the multiple effects of climate warming on the temporal shift of leaf unfolding. Nat. Clim. Change 12, 193–199. doi: 10.1038/s41558-021-01261-w
Zhang R., Lin J., Wang F., Delpierre N., Kramer K., Hänninen H., et al. (2022). Spring phenology in subtropical trees: Developing process-based models on an experimental basis. Agric. For. Meteorol. 314, 108802. doi: 10.1016/j.agrformet.2021.108802
Zhang R., Lin J., Wang F., Shen S., Wang X., Rao Y., et al. (2021). The chilling requirement of subtropical trees is fulfilled by high temperatures: A generalized hypothesis for tree endodormancy release and a method for testing it. Agric. For. Meteorol. 298-299, 108296. doi: 10.1016/j.agrformet.2020.108296
Zhang R., Lin J., Zheng J., Chuine I., Delpierre N., Janssens I., et al. (2023). Discovering ecophysiological causes of different spring phenology responses of trees to climatic warming. Agric. For. Meteorol. 340, 109593. doi: 10.1016/j.agrformet.2023.109593
Zhao L. Y., Li Y., Lin J., Cao K., Yao J.-L., Bie H-L., et al. (2023). MADS-box protein PpDAM6 regulates chilling requirement-mediated dormancy and bud break in peach. Plant Physiol. 193, 448–465. doi: 10.1093/plphys/kiad291
Zheng J., Hänninen H., Lin J., Shen S., Zhang R. (2021). Extending the cultivation area of pecan (Carya illinoinensis) toward the south in southeastern subtropical China may cause increased cold damage. Front. Plant Sci. 12, 768963. doi: 10.3389/fpls.2021.768963
Keywords: ABA, annual cycle, bud burst, chilling requirement, climatic adaptation, dormancy, GA, gene regulation
Citation: Zhang R, Hänninen H, Wu J and Bhalerao RP (2023) Bud endodormancy – a familiar but still unknown key adaptive trait in extratropical woody plants. Front. Plant Physiol. 1:1293057. doi: 10.3389/fphgy.2023.1293057
Received: 12 September 2023; Accepted: 23 October 2023;
Published: 16 November 2023.
Edited by:
Daisuke Takahashi, Saitama University, JapanReviewed by:
Namraj Dhami, Pokhara University, NepalHisayo Yamane, Kyoto University, Japan
Cristian Ibáñez, University of La Serena, Chile
Copyright © 2023 Zhang, Hänninen, Wu and Bhalerao. This is an open-access article distributed under the terms of the Creative Commons Attribution License (CC BY). The use, distribution or reproduction in other forums is permitted, provided the original author(s) and the copyright owner(s) are credited and that the original publication in this journal is cited, in accordance with accepted academic practice. No use, distribution or reproduction is permitted which does not comply with these terms.
*Correspondence: Jiasheng Wu, d3Vqc0B6YWZ1LmVkdS5jbg==; Rishikesh P. Bhalerao, cmlzaGkuYmhhbGVyYW9Ac2x1LnNl