- Univ. Bordeaux, CNRS, Laboratoire de Biogenèse Membranaire (LBM), UMR5200, Villenave D’Ornon, France
Lipids are not only structural elements that make up biological membranes, they also play a crucial role in functionalizing these membranes. Through their ability to modulate membrane physical properties, to act as sensors and signaling molecules, and to interact with proteins to influence their subcellular localization and activity, lipids contribute the intricate workings of plant cells. The enrichment of specific lipids within distinct subcellular compartments aids to the establishment of membranes unique identity and properties. Lipids are major regulators of many cellular processes including cell signaling, cell division, cell polarity, membrane trafficking, intra- and intercellular communication, cell growth, and responses to environmental stress. In fact, the immense diversity of lipid species provides plant cells with an extensive arsenal of tools to establish distinctive biochemical identities within their membranes. In this review, we present an overview of plant membrane lipids, emphasizing their role in environmental stress response by highlighting recent advancements in the field.
Introduction
Membranes play an essential role in supporting life by serving as semi-permeable barriers that delineate cells, organelles, and subcellular compartments (Harayama and Riezman, 2018). By confining molecules in a two-dimensional within the plan of the membrane, they facilitate the compartmentalization of biochemical reactions. This compartmentalization is crucial in the crowded cellular environment, allowing for the concentration and alignment of reaction partners, promoting the execution of molecular program with spatial and temporal coordination and ultimately ensuring the efficiency and effectiveness of cellular processes (Cassim et al., 2019; Gronnier et al., 2019; Leonard et al., 2023). Lipids are both building blocks of membranes and functional elements. They possess an amphipathic nature, meaning they have both hydrophobic and hydrophilic regions. When immersed in a water solution, such as the cytoplasm, they spontaneously arrange themselves into bilayers, forming closed compartments that can reseal if damaged. More than basic construction units, the very nature of lipids directly impact the biophysical properties of membranes, such as electrostatic charge, curvature, and thickness (Bigay and Antonny, 2012; Noack and Jaillais, 2020). They interact with proteins inside the bilayer and at the surface of the bilayers through they charged head and assist in spatial-temporal regulation biochemical reactions. Although often under looked, lipids are the cell undefeated superheroes. In recent decades, there has been a remarkable surge of ground breaking studies emphasizing the crucial role of lipids in cellular response mechanisms within plants. In this mini-review, we aim to offer an overview of the diverse lipid classes that make up plant membranes and present a curated selection of most recent and notable examples that illustrate how plant lipids play a regulatory role in plant cellular responses to environmental stresses.
A guided tour of the cell fatty universe
Let’s begin with some hallmarks on the identity and diversity of plant lipids. Lipids are classified into three main classes based on their chemical structures: glycerolipids, sphingolipids, and sterols (Figure 1). Every class possesses unique biochemical properties, follows a distinct biosynthesis pathway and are enriched to specific compartment resulting in a non-uniform distribution within the cell.
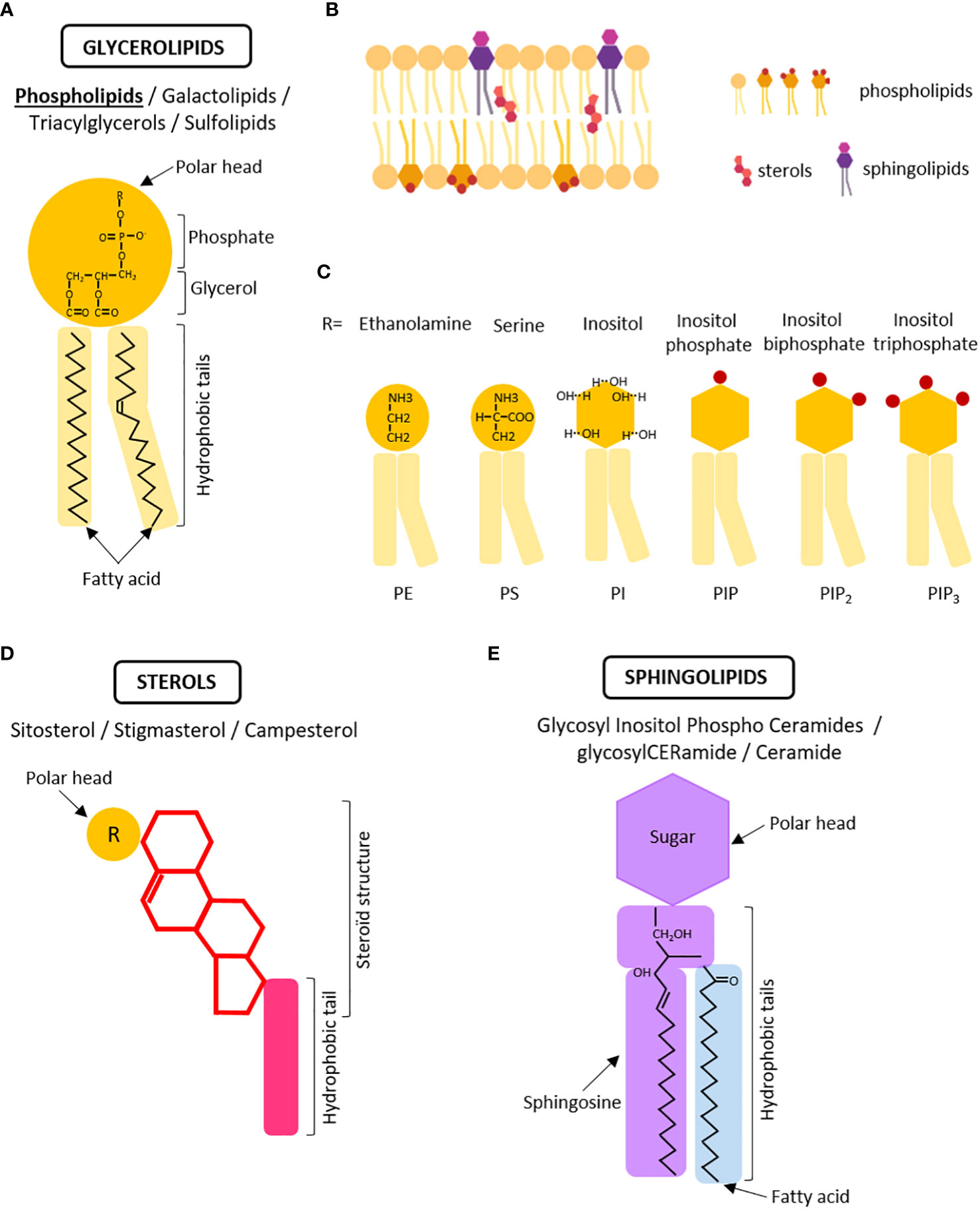
Figure 1 The three major classes of lipids within the membranes. (A) Schematic structure of phospholipids, the main class of glycerolipids. Phospholipids are composed of a polar head with a chemical moiety (R) linked to a phosphate group and a glycerol molecule connected to hydrophobic tails with fatty acids. (B) Simplified schema of a membrane in which glycerolipids, sterols, and sphingolipids are asymmetrically distributed between the inner and outer leaflets of the bilayer. The polar heads of membrane lipids face outward, whereas the hydrophobic tails face inward. (C) Modification of the polar head by chemical moieties determines the different classes of phospholipids affecting membrane properties. PE, phosphatidylethanolamine; PS, phosphatidylserine; PI, phosphatidylinositol; PIP, phosphoinositide monophosphate, PIP2=phosphoinositide biphosphate, PIP2 = phosphoinositide triphosphate. (D) Schematic representation of a sterol molecule which is characterized by the presence of a steroïd structure. (E) Schematic representation of a sphingolipid molecule identified by the presence of sphingosine in the hydrophobic tails and sugar in the polar head. Created with BioRender.com.
Glycerolipids are the most abundant class of plant membrane lipids, accounting for up to 50% of total lipids. They all consist of a glycerol backbone with one to three fatty acid chains (long hydrocarbon chains) attached to it, and are divided into four subcategories; (glycero)phospholipids (PL), (glycero)galactolipids (GL), triacylglycerols (TAG), and (glycero)sulfolipids (SL) (Figure 1A). When constructing membranes, the primary building blocks are phospholipids (and galactolipids in plastids). When in an aqueous environment like the cell, phospholipids spontaneously arrange themselves into a bilayer structure. The hydrophilic, water-loving, phosphate heads face outward, interacting with the surrounding water molecules. Meanwhile, the hydrophobic, water-fearing, fatty acid tails face inward, shielding themselves from the water (Figure 1B). Phospholipids, like other classes, demonstrate significant structural diversity. For example, the length of the hydrocarbon fatty acid chain can vary, influencing both the membrane thickness and the sorting of proteins through hydrophobic mismatch (the disparity between the thickness of the hydrophobic region of a transmembrane protein and that of the biological membrane) (Milovanovic et al., 2015). Likewise, fatty acids can be saturated or unsaturated based on the presence or absence of double bonds between carbon atoms in the hydrocarbon chain. These double bonds introduce bends or kinks in the chain, making it less rigid and once again affecting the properties of the membrane (Figure 1A) (Bigay and Antonny, 2012). For instance, polyunsaturated glycerophospholipids tend to promote membrane vesiculation, by allowing the membrane to be flexible enough to divide by fission (Manni et al., 2018). Phospholipids also brings different chemical flavor depending on the polar head they carry: Phosphatidylethanolamine (PE) consists of a glycerol linked to a phosphate and an ethanolamine moiety, while phosphatidylserine (PS) contains a glycerol linked to a phosphate and a serine moiety (Figure 1C). Phosphatidylinositol, which carry a glycerol linked to a phosphate and an inositol moiety can be further phosphorylated at three different positions, leading to PI3P, PI4P, PI5P, and bi/tri-phosphate derivatives (PI(3,4)P2, PI(3,5)P2, PI(4,5)P2, and PI(3,4,5)P3), collectively refer to as phosphoinositides (Figure 1C). Through their negative charges, PA, PS, PI, and PIPs (also collectively called anionic phospholipids) can interact and attract with proteins harboring lipid-binding domains (Noack and Jaillais, 2017; Noack and Jaillais, 2020). This diversity of polar heads and combinations of anionic lipids help defining the identity of biological membranes by creating unique electrostatic signatures (Platre et al., 2018). For instance, PI4P, PA, and PS are the main species generating the electrostatic signature of the PM. An electrostatic gradient is present along the endocytic pathway contributing to the distinctive protein localization within the cell hence to overall cellular function (Platre et al., 2018).
For instance, despite being present in minor amounts (approximately 2% of total phospholipids for PA, 1% for PS, and less than 1% for all PIPs combined), these anionic lipids are fundamental components of cell membranes and play a crucial role in regulating various membrane-associated processes, such as cell signaling, trafficking, cell division, and cell growth. Consequently, any imbalances in anionic lipids can lead to significant and diverse effects at the plant level including plant development, immunity, and stress adaptation.
Phospholipids associate with phytosterols and sphingolipids to form membranes. Higher plant present an extensive variety of sterols, up to 60 sterols and pentacyclic triterpenes identified in maize seedlings (Grosjean et al., 2015). Among these, -sitosterol, stigmasterol, and campesterol are predominant (Figure 1D). The molecular structure of phytosterols typically consists of 28 or 29 carbons and differs from cholesterol, found in animal, due to the presence of an additional alkyl group (either ethyl or methyl) on the side chain at the C24 position and an extra carbon-carbon double bond in the sterol nucleus (Figure 1D). Phytosterols associated with phospholipids and sphingolipids through their fatty acyl chain, been embedded with the bilayer. They constitute up to 30% of plasma membrane lipids (Cassim et al., 2019; Bahammou et al., 2023) and act as regulator of membrane fluidity and permeability to small solutes (Grosjean et al., 2015; Cassim et al., 2019; Frallicciardi et al., 2022).
The last class of lipids, known as sphingolipids, exhibits some differences between animals and plants. Unlike animals, plants do not contain sphingomyelin or gangliosides. Instead, their sphingolipids predominantly consist of Glycosyl Inositol Phospho Ceramides (GIPCs), glycosylCERamide (gluCER), and ceramide (Markham et al., 2006; Buré et al., 2014; Gronnier et al., 2016) (Figure 1E). Sphingolipids are composed of a sphingosine or sphingoid backbone linked to a fatty acid chain and a polar head group. In Arabidopsis, GIPCs make up approximately 64% of total sphingolipids, while glycosylCERamide constitutes around 34%, and ceramide accounts for approximately 2% (Gronnier et al., 2016; Cassim et al., 2019). These lipids are believed to be mainly located in the outer leaflet of the plasma membrane, and harbor with a highly-polar head group consisting of a phosphate attached to an inositol, which is further modified with various sugar moieties.
The intricate interactions of these three lipid classes, facilitated by the diversity of species, subcellular localization, and inner and outer leaflet localizations, play a vital role in forming and regulating complex biological membrane-based cellular processes. In the upcoming sections, we have selected five recent and noteworthy examples illustrating how lipids play a crucial role in regulating plant cellular and physiological responses to various forms of stress, whether biotic or abiotic.
Membrane contact site: lipid on the move for stress relief
Most lipids are synthesized in ER and transported to their final membrane compartments through vesicular or non-vesicular trafficking at membrane contact sites. These sites enable direct lipid exchange by bringing two membranes into close proximity and recruiting lipid-transfer proteins (Michaud and Jouhet, 2019). Non-vesicular lipid transfer from the endoplasmic reticulum (ER) to the plasma membrane (PM) has been originally suggested by pulse chase experiments showing that the transfer of phospholipids is reduced but not blocked upon inhibition of vesicular trafficking (Moreau et al., 1998). However, the specific mechanisms and actors involved in this transfer had remained unknown. In a recent study, Ruiz-Lopez et al. (2021) (Ruiz-Lopez et al., 2021) uncovered the molecular participants and functional aspects of lipid transfer at membrane contact sites in response to abiotic stress.
When plants are subjected to cold or drought stress, a lipid-dependent signaling pathway is activated, resulting in the production of diacylglycerol (two fatty acid chains covalently bonded to a glycerol molecule with the polar head consisting only of a hydroxyl group) at the PM. However, an excessive accumulation of this lipid can be problematic, and lead to a loss of membrane integrity (Campomanes et al., 2019). While part of the diacylglycerol pool is converted into phosphatidic acid by diacylglycerol kinases, Ruiz-Lopez et al. (2021) discovered that the remaining diacylglycerol is efficiently removed through the action of Synaptotagmin (SYT)1/SYT3 proteins at ER-PM membrane contact sites (Figure 2). The Synaptotagmin family comprises five members, each containing an ER anchor, a lipid transfer domain called Synaptotagmin-like Mitochondrial-lipid-binding Protein (SMP), and two C2 lipid-binding domains for PM interaction (Pérez-Sancho et al., 2015; Reinisch and De Camilli, 2016). Both SYT1 and SYT3 are found at ER-PM membrane contact sites, and their localization depends on the presence of phosphatidylinositol 4-phosphate (PI4P) anionic lipid which interact with their C2 domains. Under cold stress, PI4P levels increase at the PM, promoting the expansion of ER-PM contact sites by recruiting SYT1/SYT3 proteins. Interestingly, a similar increase in ER-PM contact sites marked by SYT1 has been observed in response to salt stress (Lee et al., 2019), indicating that SYT proteins function as general abiotic stress-regulated tethers. Arabidopsis mutants lacking SYT1, SYT3, or both genes exhibit compromised PM integrity under cold stress, accompanied by diacylglycerol accumulation at the PM. SYT1, through its SMP domain’s hydrophobic groove, selectively binds to diacylglycerol species, indicative of a role in transporting this lipid from the PM to the ER (Ruiz-Lopez et al., 2021). These findings not only provide a first mechanistic understanding of how lipid transfer can help preventing the harmful accumulation of diacylglycerol during abiotic stress but also highlight the significance of ER-PM contact sites in lipid homeostasis and the adaptive response of plants to abiotic stresses. Future work will elucidate whether or not, as in animal and yeast, ER-PM contact sites serve the exchange of other lipid species in the context of stress but also developmental program responses.
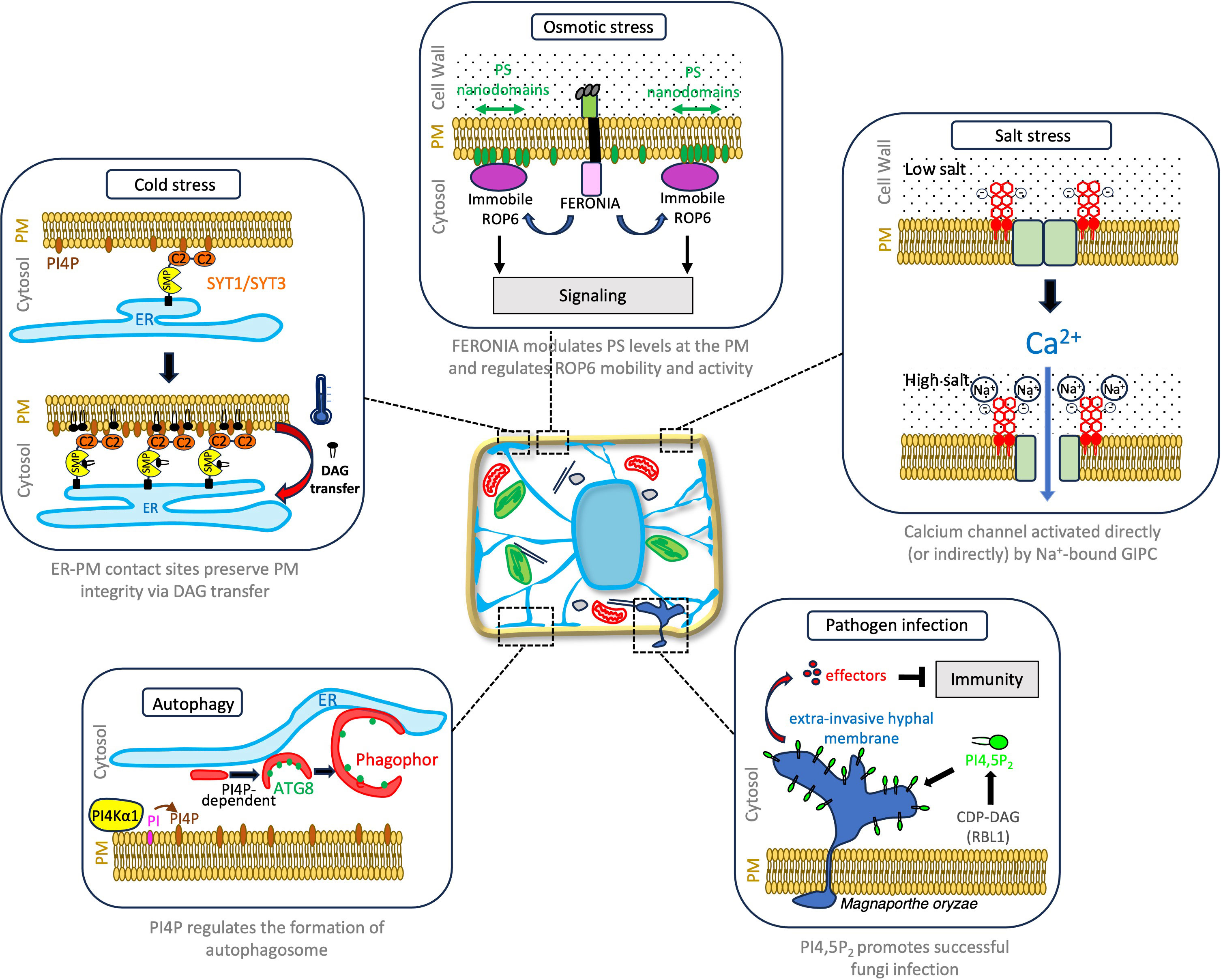
Figure 2 Notable examples of how plant lipids play a regulatory role in plant cellular responses to environmental stresses.
Autophagy: one lipid at every step
Autophagy, a conserved recycling process, transports cellular components like proteins, protein aggregates, and organelles to the lytic vacuole for degradation (Marshall and Vierstra, 2018). While autophagy occurs at a basal level during optimal growth conditions, it is highly induced under stress to eliminate unwanted or toxic components, and recycle resources. Autophagy involves the formation of double membrane vesicles called autophagosomes that encapsulate cellular components and direct them to the lytic vacuole for degradation. This process entails intense membrane remodeling, including nucleation and assembly of the pre-autophagosome, formation of the cup-shaped phagophore, and subsequent expansion and fusion to form a fully-formed autophagosome. The multi-step regulation of this process involves the sequential recruitment of conserved ATG proteins to the autophagosome structure, and lipids (Enrique Gomez et al., 2018; Gomez et al., 2021). Upon autophagy induction, ATG1/ATG13 proteins recruit the phosphatidylinositol 3-kinase (PI3K) complex, to initiate the nucleation of the phagophore (Nascimbeni et al., 2017). Early accumulation of PI3P is a hallmark of autophagy, occurring in mammals, yeast, and plants, which enables the recruitment of downstream ATG proteins such as ATG18, ATG2, and the ATG12-ATG5-ATG16 complex (Gomez et al., 2021). Concomitantly, lipidation of ATG8 by the membrane lipid phosphatidylethanolamine (PE) through a in a ubiquitin-like conjugation reaction, where ATG8 becomes attached to the headgroup of PE, is necessary for membrane elongation and autophagosome biogenesis. Mutants with defects in ATG8 conjugation are unable to form mature autophagosomes (Yoshimoto et al., 2004; Thompson et al., 2005; Chung et al., 2010). The autophagy field has long recognized the importance of studying lipids, and our understanding of their significance in the autophagy process continues to grow, including in plants. Recently, by performing a lipid inhibitor screen, Gomez et al. (2022) identified PI4P as an additional regulatory lipid of autophagy. Decrease in PI4P levels prevents ATG8 lipidation and is required for the proper structuration and elongation of the phagophore (Figure 2). In contrast, PI4P is not crucial for the initial membrane association of ATG proteins, suggesting its non-essential role in the early stages of phagophore nucleation (Gomez et al., 2022). While previous research has primarily emphasized the critical role of the ER as a platform for autophagophore formation, this study uncovers the involvement of the PI4P-enriched PM and revealing a consistent association between autophagy structures and the PM. In line with these finding, autophagy is inhibited in Arabidopsis mutants lacking functional PM-located PI4Kα1, an enzyme responsible for generating PI4P through phosphatidylinositol phosphorylation. Gomez et al.’s work nicely complements a recent study by Wang et al., 2019, which highlighted the role of ER-PM contact sites and associated proteins in autophagy (Wang et al., 2019). What’s more, as previously pointed PI4P contribute to the establishment and expansion of ER-PM contact sites under stress conditions (Ruiz-Lopez et al., 2021). The accumulation of PI4P, in conjunction with other anionic lipids, contributes significantly to membrane identity and function by selectively recruiting specific protein sets through electrostatic interactions (Noack and Jaillais, 2020). In the context of autophagy, it remains to be determined whether PI4P is directly localized within autophagic membranes or at PM sites in contact with the pre-autophagosomal structure (PAS), the point of origin for phagophores. A plausible hypothesis is that PI4P facilitates the recruitment of a yet unidentified PI4P-binding protein(s) that operates upstream of ATG8 lipidation and plays a role in the proper assembly and expansion of the phagophore, most likely at ER-PM contact sites.
Cereal disease resistance: when harnessing anionic lipids helps to combine resistance and yield
Cereal diseases pose a major threat to global food security, with bacteria, fungi, and viruses being the primary culprits. Fungal attacks on cereals are particularly detrimental, causing a significant reduction in crop yields worldwide and endangering the food supply for many individuals. While plants exhibit remarkable adaptability, there is a trade-off between survival and growth, and resistance to pathogens often comes at the expense of lower yields.
A recent study published in Nature uncovered a link between phospholipid metabolism and disease resistance (Sha et al., 2023). Through a genetic screen, researchers identified a mutant variant in a mutagenized rice population that imparts resistance to ten different strains of Magnaporthe oryzae, five strains of the bacterium Xanthomonas oryzae, and two strains of the ascomycete fungus Ustilaginoidea virens. This mutant carries a 29-base-pair deletion in a gene called RESISTANCE TO BLAST1 (RBL1), which encodes a cytidine diphosphate diacylglycerol synthase necessary for phospholipid production. The mutation in RBL1 leads to decreased levels of phosphatidylinositol and its derivative, phosphatidylinositol 4,5-bisphosphate (P(4,5)P2) which has been previously shown to serve as a disease-susceptibility factor and to be recruited to the extra-invasive hyphal membrane infection sites to facilitate infection in Arabidopsis (Shimada et al., 2019; Qin et al., 2020). Similarly, in rice, P(4,5)P2 is enriched in cellular structures associated with effector secretion and fungal infection (Sha et al., 2023). While the RBL1 lesion mimic mutation confers broad-spectrum disease resistance, it also leads to a significant reduction in crop yield. Hence, the fungus relies on the host’s P(4,5)P2 for successful infection, so reducing P(4,5)P2 levels benefits the plant in terms of pathogen resistance (Figure 2). However, this lipid also plays a crucial role in various developmental processes (Noack and Jaillais, 2020), making the reduction of P(4,5)P2 detrimental to plant growth. To address this challenge, the researchers employed targeted genome editing to generate an RBL1 allele called RBL1Δ12. This allele carries a 12-base-pair deletion that significantly reduces gene expression but does not affect protein thermostability or subcellular localization. The RBL1Δ12 mutation still confers broad-spectrum disease resistance by reducing P(4,5)P2 levels at infection sites, but does not result in reduced yield. Further investigations are required to determine if RBL1Δ12 plants will equally cope with abiotic stresses.
When membrane surface sphingolipids get a taste for salt
Salt stress in plants primarily arises from sodium chloride (NaCl). When present in high concentrations, NaCl becomes cytotoxic for the cells as the Na+ ions compete with other ions, and disrupt the ion and water balance, resulting in osmotic perturbations. Until recently, it remained uncertain how plants perceive stress caused by excessive salt and whether they can differentiate between ionic and osmotic perturbations. The answer lies in their utilization of surface lipids, as recently shown by Jiang et al., 2019. When plants are exposed to salt stress, they immediately experience a rise in the concentration of cytosolic Ca2+, which exhibits a specific temporal and spatial signature (Knight et al., 1997; Jiang et al., 2019). This Ca2+ signal triggers cellular adaptations in plant tissues, both locally in the roots and in distant tissues. The removal of excess intracellular Na+ involves the Ca2+-related salt-overly-sensitive (SOS) pathway, which comprises the Ca2+ sensor SOS3, the protein kinase SOS2, and the Na+/H+ antiporter SOS1 (Ali et al., 2023). NaCl-triggered influx of Ca2+ stimulates an adaptive response to high salt levels, wherein the Ca2+-binding protein SOS3 activates the protein SOS2, which subsequently triggering active transport of Na+ out of the cell, through SOS1.
Through a genetic screening approach, Jiang and colleagues identified a mutant called monocation-induced Ca2+ intracellular increases 1 (moca1), which is unable to respond to high Na+ exposure. The moca1 mutant exhibited heightened sensitivity to salt stress, reduced Ca2+ signaling response, and impaired changes in membrane polarization and activation of the SOS pathway. MOCA1 encodes for IPUT1, a glucuronosyltransferase located in the Golgi apparatus, responsible for producing Glycosyl Inositol Phospho Ceramides (GIPCs) lipids that are major components of the outer leaflet of PM (Cacas et al., 2016). Taking an unexpected turn, the authors also demonstrated that GIPCs have the ability to directly bind external Na+ ions, suggesting that in contrast to animal that senses Na+ ions by protein receptors (Zhang et al., 2013), plants use surface lipids. Although the precise mechanism by which Na+-bound GIPC activates the calcium channel remains unknown, it is speculated that the binding of Na+ ions to GIPCs might modulate Ca2+ channel activity through either direct binding or by influencing the dynamics and assembly of protein complexes at the PM (Figure 2). Nevertheless, this study significantly highlights the critical role of GIPCs in directly binding Na+ ions as an essential step in sodium sensing in plants, triggering calcium signals that subsequently lead to salt-tolerance responses. The findings of this study echo the results another study, which revealed that GIPCs also serve as receptors for microbial toxins called NLP (necrosis and ethylene-inducing peptide 1-like protein) toxins that are produced by pathogenic bacteria, fungi, and oomycetes (Lenarčič et al., 2017). NLPs form complexes with the termina hexose moieties of GIPCs, which induces conformational changes within the toxin facilitating PM insertion. Taken together, these findings underscore the increasing significance of GIPCs as essential molecules involved in a wide range of sensing and signaling functions in plants, strategically positioned to face the extracellular space. Future research should focus on elucidating the precise mechanisms responsible for GIPC-dependent calcium channel activation and exploring whether GIPC also function as co-receptors for other extracellular signals.
Plant under osmotic pressure: dealing with water loss through PS nanodomains
When plants experience hyperosmotic stress, one of the initial cellular responses is the accumulation of reactive oxygen species (ROS) in the apoplast, through notably the PM-located RESPIRATORY BURST OXIDASE HOMOLOGUE (RBOH). Acting downstream of osmotic signal perception, ROS molecules serve as secondary messengers, which trigger plant adaptative responses (Martinière et al., 2019). One pathway leading to ROS accumulation is through Rho-of-plants (ROP) 6, a small guanosine triphosphatases (GTPases) (Smokvarska et al., 2020). Under hyperosmotic stress conditions ROP6 is rapidly targeted to specific nanodomains of the PM, that contain RBOHs and are responsible for ROS production (Figure 2). In the context of auxin signaling, ROP6 was shown to interact through lysine residues present in its hyper-variable tail with anionic lipids, including PS, one of the anionic lipids on the PM inner leaflet. This interaction with PS has an impact on the duration of ROP6 retention within the nanodomains (Platre et al., 2019). Nevertheless, the connection between PS and the activation of ROP6 in the context of osmotic stress had not been established until now.
In a recent study Smokvarska et al. (2023) revealed the involvement of FERONIA, a PM-located protein, member of the Catharanthus roseus receptor-like kinase (CrRLK1L) family, in PS-regulated osmotic perception (Figure 2). FERONIA, well-known for its role as a sensor for cell wall integrity, was found to play a crucial role in the reorganization of PS at the PM which impact on ROP6 localization and ROS-signaling (Smokvarska et al., 2023). PS-nanodomains are lost in feronia loss-of-function mutant while the overall quantity of PS at the cellular level is not modified. Activation of the FERONIA pathway through RALF23, induces a transient accumulation of PS biosensors at the PM after 15 min, a response that is lost in ferronia mutant. Moreover, in the absence of FER, the formation of ROP6 nanodomains upon osmotic stress still occurs. However, there is a notable difference as ROP6 is no longer immobilized within these nanodomains, and instead exhibits increased mobility, moving in and out of the nanodomains more frequently compared to wild-type plants. A similar link between PS nanodomain organization and ROP6 dynamics and activation at the PM, was previously shown in the context of auxin-responses (Platre et al., 2019). Upon osmotic stress, loss of FERONIA function also reduces the level of activated ROP6 and hampers ROS production, hence cellular adaptative responses. FERONIA influences the cellular distribution and nanodomain organization of PS at the PM, which is in turn essential for the dynamic behavior and functional efficacy of ROP6. Furthermore, complementation assay of exogenously added PS or genetic overexpression results in rescue of the cellular and some physiological phenotypes of feronia mutant, straightening this tripartite relationship between FERONIA, PS and ROP6.
Interestingly this works also echoes on the function of FERONIA in the context of light stress response (Shin et al., 2021), which also induces ROS production at the PM in phloem and xylem parenchyma cells (Zandalinas et al., 2020). Altogether Smokvarska et al. (2023) work exemplifies the active role of lipids in orchestrating precise molecular programs in spatial and temporal dimensions, highlighting the crucial function of membranes as the foundational platform upon which cellular processes are organized and supported.
Conclusion
In conclusion, lipids play diverse and pivotal roles in various cellular processes in plants. Whether they function as sensors in response to extracellular cues, facilitate membrane remodeling and protein targeting for autophagy and osmotic stress regulation, or act as signaling molecules during cold-stress adaptation, lipids exhibit ubiquitous and fundamental involvement throughout cellular systems. In light of their essential role and despite the inherent challenges associated with their study, it is imperative to continue exploring the complexities of lipids, recognizing their fundamental significance in plant related cellular and molecular processes.
Author contributions
HM: Writing – original draft. EB: Writing – original draft.
Funding
The author(s) declare financial support was received for the research, authorship, and/or publication of this article. This work was supported by the European Research Council (ERC) under the European Union’s Horizon 2020 research and innovation program (project n° 772103-BRIDGING to EB), and ANR project DIVCON (project n° ANR-21-CE13-0016-01 to EB).
Conflict of interest
The authors declare that the research was conducted in the absence of any commercial or financial relationships that could be construed as a potential conflict of interest.
The author(s) EB declared that they were an editorial board member of Frontiers, at the time of submission. This had no impact on the peer review process and the final decision.
Publisher’s note
All claims expressed in this article are solely those of the authors and do not necessarily represent those of their affiliated organizations, or those of the publisher, the editors and the reviewers. Any product that may be evaluated in this article, or claim that may be made by its manufacturer, is not guaranteed or endorsed by the publisher.
References
Ali A., Petrov V., Yun D. J., Gechev T. (2023). Revisiting plant salt tolerance: novel components of the SOS pathway. Trends Plant Sci 28 (9), P1060–1069. doi: 10.1016/j.tplants.2023.04.003
Bahammou D., Recorbet G., Mamode Cassim A., Robert F., Balliau T., Van Delft P., et al. (2023). Revealing the lipidome and proteome of Arabidopsis thaliana plasma membrane. bioRxiv, 540643.
Bigay J., Antonny B. (2012). Curvature, lipid packing, and electrostatics of membrane organelles: defining cellular territories in determining specificity. Dev. Cell 23, 886–895.
Buré C., Cacas J., Mongrand S. (2014). Characterization of glycosyl inositol phosphoryl ceramides from plants and fungi by mass spectrometry. Anal Bioanal Chem 406, 995–1010.
Cacas J-LJ-LJL., Buré C., Grosjean K., Gerbeau-Pissot P., Lherminier J., Rombouts Y., et al. (2016). Re-visiting plant plasma membrane lipids in Tobacco: a focus on sphingolipids. Plant Physiol. 170, 367–384.
Campomanes P., Zoni V., Vanni S. (2019). Local accumulation of diacylglycerol alters membrane properties nonlinearly due to its transbilayer activity. Commun. Chem. 2, 1–8. doi: 10.1038/s42004-019-0175-7
Cassim A. M., Gouguet P., Gronnier J., Laurent N., Germain V., Grison M., et al. (2019). Plant lipids: Key players of plasma membrane organization and function. Prog. Lipid Res. 73, 1–27.
Chung T., Phillips A. R., Vierstra R. D. (2010). ATG8 lipidation and ATG8-mediated autophagy in Arabidopsis require ATG12 expressed from the differentially controlled ATG12A and ATG12B loci. Plant J. 62, 483–493.
Enrique Gomez R., Joubès J., Valentin N., Batoko H., Satiat-Jeunemaître B., Bernard A. (2018). Lipids in membrane dynamics during autophagy in plants. J. Exp. Bot. 69, 1287–1299.
Frallicciardi J., Melcr J., Siginou P., Marrink S. J., Poolman B. (2022). Membrane thickness, lipid phase and sterol type are determining factors in the permeability of membranes to small solutes. Nat. Commun. doi: 10.1038/s41467-022-29272-x
Gomez R. E., Chambaud C., Lupette J., Castets J., Pascal S., Brocard L., et al. (2022). Phosphatidylinositol-4-phosphate controls autophagosome formation in Arabidopsis thaliana. Nat. Commun. doi: 10.1038/s41467-022-32109-2
Gomez R. E., Lupette J., Chambaud C., Castets J., Ducloy A., Cacas J. L., et al. (2021). How lipids contribute to autophagosome biogenesis, a critical process in plant responses to stresses. Cells. doi: 10.3390/cells10061272
Gronnier J., Germain V., Gouguet P., Cacas J. L., Mongrand S. (2016). GIPC: Glycosyl inositol phospho ceramides, the major sphingolipids on earth. Plant Signal Behav 11, 4. doi: 10.1080/15592324.2016.1152438
Gronnier J., Legrand A., Loquet A., Habenstein B., Germain V., Mongrand S. (2019). Mechanisms governing subcompartmentalization of biological membranes. Curr. Opin. Plant Biol. 52, 114–123.
Grosjean K., Mongrand S., Beney L., Simon-Plas F., Gerbeau-Pissot P. (2015). Differential effect of plant lipids on membrane organization specificities of phytosphingolipids and phytosterols. J. Biol. Chem. 290, 5810–5825.
Harayama T., Riezman H. (2018). Understanding the diversity of membrane lipid composition. Nat. Rev. Mol. Cell Biol. 19, 281–296.
Jiang Z., Zhou X., Tao M., Yuan F., Liu L., Wu F., et al. (2019). Plant cell-surface GIPC sphingolipids sense salt to trigger Ca2+ influx. Nature 572, 341–346.
Knight H., Trewavas A. J., Knight M. R. (1997). Calcium signalling in Arabidopsis thaliana responding to drought and salinity. Plant J. 12, 1067–1078.
Lee E., Vanneste S., Pérez-sancho J., Benitez-Fuente F., Strelau M., Macho A. P., et al. (2019). Ionic stress enhances ER – PM connectivity via site expansion in Arabidopsis. PNAS 116, 1420–1429.
Lenarčič T., Albert I., Böhm H., Hodnik V., Pirc K., Zavec A. B., et al. (2017). Eudicot plant-specific sphingolipids determine host selectivity of microbial NLP cytolysins. Science 80-) 358, 1431–1434.
Leonard T. A., Loose M., Martens S. (2023). The membrane surface as a platform that organizes cellular and biochemical processes. Dev. Cell 58, 1–18.
Manni M. M., Tiberti M. L., Pagnotta S., Lè Ne Barelli H., Gautier R., Antonny B. (2018). Acyl chain asymmetry and polyunsaturation of brain phospholipids facilitate membrane vesiculation without leakage. Elife. doi: 10.7554/eLife.34394.001
Markham J. E., Li J., Cahoon E. B., Jaworski J. G. (2006). Separation and identification of major plant sphingolipid classes from leaves. J. Biol. Chem. 281, 22684–22694.
Marshall R. S., Vierstra R. D. (2018). Autophagy: the master of bulk and selective recycling. Annu. Rev. Plant Biol. 69, 173–208.
Martinière A., Fiche J. B., Smokvarska M., Mari S., Alcon C., Dumont X., et al. (2019). Osmotic stress activates two reactive oxygen species pathways with distinct effects on protein nanodomains and diffusion. Plant Physiol. 179, 1581–1593.
Michaud M., Jouhet J. (2019). Lipid Trafficking at membrane contact sites during plant development and stress response. Front. Plant Sci. 10. doi: 10.3389/fpls.2019.00002
Milovanovic D., Honigmann A., Koike S., Göttfert F., Pähler G., Junius M., et al. (2015). Hydrophobic mismatch sorts SNARE proteins into distinct membrane domains. Nat. Commun. 6, 1–26.
Moreau P., Bessoule J. J., Mongrand S., Testet E., Vincent P., Cassagne C. (1998). Lipid trafficking in plant cells. Prog. Lipid Res. 37, 371–391.
Nascimbeni A. C., Codogno P., Morel E. (2017). Phosphatidylinositol-3-phosphate in the regulation of autophagy membrane dynamics. FEBS J. 284, 1267–1278.
Noack L. C., Jaillais Y. (2017). Precision targeting by phosphoinositides: how PIs direct endomembrane trafficking in plants. Curr. Opin. Plant Biol. 40, 22–33.
Noack L. C., Jaillais Y. (2020). Functions of anionic lipids in plants. Annu. Rev. Plant Biol. 71, 71–102.
Pérez-Sancho J., Vanneste S., Lee E., McFarlane H., Esteban del Valle A., Valpuesta V., et al. (2015). The Arabidopsis SYT1 is enriched in ER-PM contact sites and confers cellular resistance to mechanical stresses. Plant Physiol. 168, 132–143.
Platre M. P., Bayle V., Armengot L., Bareille J., Del Mar Marquès-Bueno M., Creff A., et al. (2019). Developmental control of plant Rho GTPase nano-organization by the lipid phosphatidylserine. Science 80-) 364 (6435), 57–62. doi: 10.1126/science.aav9959ï
Platre M. P., Noack L. C., Doumane M., Bayle V., Simon M. L. A., Maneta-Peyret L., et al. (2018). A Combinatorial lipid code shapes the electrostatic landscape of plant endomembranes. Dev. Cell 45, 465–480.e11.
Qin L., Zhou Z., Li Q., Zhai C., Liu L., Quilichini T. D., et al. (2020). Specific recruitment of phosphoinositide species to the plant-pathogen interfacial membrane underlies Arabidopsis susceptibility to fungal infection. Plant Cell 32, 1665–1688.
Reinisch K. M., De Camilli P. (2016). SMP-domain proteins at membrane contact sites: Structure and function. Biochim. Biophys. Acta - Mol. Cell Biol. Lipids 1861, 924–927.
Ruiz-Lopez N., Pérez-Sancho J., del Valle A. E., Haslam R. P., Vanneste S., Catalá R., et al. (2021). Synaptotagmins at the endoplasmic reticulum–plasma membrane contact sites maintain diacylglycerol homeostasis during abiotic stress. Plant Cell 33, 2431–2453.
Sha G., Sun P., Kong X., Han X., Sun Q., Fouillen L., et al. (2023). Genome editing of a rice CDP-DAG synthase confers multipathogen resistance. Nature. doi: 10.1038/s41586-023-06205-2
Shimada T. L., Betsuyaku S., Inada N., Ebine K., Fujimoto M., Uemura T., et al. (2019). Enrichment of phosphatidylinositol 4,5-bisphosphate in the extra-invasive hyphal membrane promotes colletotrichum infection of arabidopsis thaliana. Plant Cell Physiol. 60, 1514–1524.
Shin S. Y., Park J. S., Park H.B., Moon K. B., Kim H. S., Jeon J. H., et al. (2021). FERONIA confers resistance to photooxidative stress in Arabidopsis. Front. Plant Sci. 12. doi: 10.3389/fpls.2021.714938
Smokvarska M., Bayle V., Maneta-Peyret L., Fouillen L., Poitout A., Dongois A., et al. (2023). The receptor kinase FERONIA regulates phosphatidylserine localization at the cell surface to modulate ROP signaling. Sci. Adv 9 (14). doi: 10.1126/sciadv.add4791
Smokvarska M., Francis C., Platre M. P., Fiche J. B., Alcon C., Dumont X., et al. (2020). A plasma membrane nanodomain ensures signal specificity during osmotic signaling in plants. Curr. Biol. 30, 4654–4664.e4.
Thompson A. R., Doelling J. H., Suttangkakul A., Vierstra R. D. (2005). Autophagic nutrient recycling in Arabidopsis directed by the ATG8 and ATG12 conjugation pathways. Plant Physiol. 138, 2097–2110.
Wang P., Pleskot R., Zang J., Winkler J., Wang J., Yperman K., et al. (2019). Plant AtEH/Pan1 proteins drive autophagosome formation at ER-PM contact sites with actin and endocytic machinery. Nat. Commun. doi: 10.1038/s41467-019-12782-6
Yoshimoto K., Hanaoka H., Sato S., Kato T., Noda T., Ohsumi Y. (2004). Processing of ATG8s, ubiquitin-like proteins, and their deconjugation by ATG4s are essential for plant autophagy. Plant Cell 16, 2967–2983.
Zandalinas S. I., Fichman Y., Mittler R. (2020). Vascular bundles mediate systemic reactive oxygen signaling during light stress. Plant Cell 32, 3425–3435.
Keywords: membrane lipids, plant cell, membrane contact site (MCS), autophagy, environmental stresses
Citation: Moreau H and Bayer EM (2023) Lipids: plant biology’s slippery superheroes. Front. Plant Physiol. 1:1273816. doi: 10.3389/fphgy.2023.1273816
Received: 07 August 2023; Accepted: 02 October 2023;
Published: 23 October 2023.
Edited by:
Christopher I. Cazzonelli, Western Sydney University, AustraliaReviewed by:
Yosef Fichman, Tel Aviv University, IsraelKai Xun Chan, Australian National University, Australia
Copyright © 2023 Moreau and Bayer. This is an open-access article distributed under the terms of the Creative Commons Attribution License (CC BY). The use, distribution or reproduction in other forums is permitted, provided the original author(s) and the copyright owner(s) are credited and that the original publication in this journal is cited, in accordance with accepted academic practice. No use, distribution or reproduction is permitted which does not comply with these terms.
*Correspondence: Hortense Moreau, aG9ydGVuc2UubW9yZWF1QHUtYm9yZGVhdXguZnI=; Emmanuelle M. Bayer, ZW1tYW51ZWxsZS5iYXllckB1LWJvcmRlYXV4LmZy
†ORCID: Hortense Moreau, orcid.org/0000-0001-9739-3987
Emmanuelle M. Bayer, orcid.org/0000-0001-8642-5293