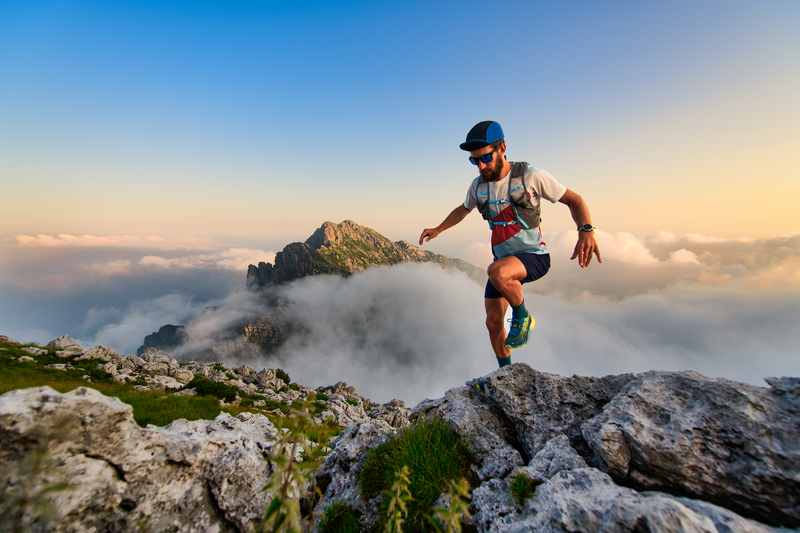
95% of researchers rate our articles as excellent or good
Learn more about the work of our research integrity team to safeguard the quality of each article we publish.
Find out more
REVIEW article
Front. Physiol. , 20 March 2025
Sec. Integrative Physiology
Volume 16 - 2025 | https://doi.org/10.3389/fphys.2025.1520669
Sepsis is defined as a life-threatening organ dysfunction caused by a dysregulated host response to infection. Skeletal muscle atrophy due to critical illness is a common phenomenon in the intensive care unit (ICU) and is referred to as ICU-acquired weakness (ICU-AW). The occurrence of ICU-AW in patients with sepsis is known as sepsis-acquired weakness (SAW). Furthermore, it is well known that maintaining normal muscle function closely relates to mitochondrial homeostasis. Once mitochondrial function is impaired, both muscle quality and function are affected. Copper plays a key role in mitochondrial homeostasis as a transition metal that regulates the function and stability of various enzymes. Copper is also involved in oxidation-reduction reactions, and intracellular copper overload causes oxidative stress and induces cell death. Previous studies have shown that excess intracellular copper induces cell death by targeting lipid-acylated proteins that regulate the mitochondrial tricarboxylic acid (TCA) cycle, which differs from the known canonical mechanisms of regulated cell death. Furthermore, inhibitors of cell death, such as apoptosis, necroptosis, pyroptosis and ferroptosis, are not effective in preventing copper-induced cell death. This new form of cell death has been termed “Cuproptosis”; however, the mechanism by which copper-induced cell death is involved in SAW remains unclear. In this paper, we review the possible relationship between cuproptosis and SAW. Cuproptosis may be involved in regulating the pathological mechanisms of SAW through mitochondria-related signaling pathways, mitochondria-related ferroptosis mechanisms, and mitochondria-related genes, and to provide new ideas for further investigations into the mechanism of SAW.
Sepsis is a life-threatening organ dysfunction caused by a dysregulated host response to infection (Singer et al., 2016), and is one of the leading causes of death in the intensive care unit (ICU), with a mortality rate of 30%–50% (Chen et al., 2019). In critically ill patients, common symptoms include skeletal muscle weakness and muscle atrophy, characterized by flaccid, symmetrical weakness of the skeletal muscles of the extremities. Respiratory muscles may also be affected, through a syndrome known as ICU-acquired weakness (ICU-AW) (Klawitter et al., 2023). The incidence of ICU-AW ranges from 25% to 100%, with risk factors including sepsis, immobilization, hyperglycemia, glucocorticoids, neuromuscular blocking agents, and multi-organ failure (Zorowitz, 2016). ICU-AW is most common in persistently critically ill patients (Latronico, 2016), and up to 60%–100% of patients with sepsis can develop ICU-AW, known as sepsis-acquired weakness (SAW) (Mitobe et al., 2019; Bellaver et al., 2023). ICU-AW is reportedly an additional organ failure after severe sepsis and septic shock (Schefold et al., 2010), and that mitochondrial dysfunction in skeletal muscle and lymphocytes is a key trigger of SAW (Maestraggi et al., 2017).
Mitochondria are encapsulated by an outer and an inner phospholipid membrane that divides the organelle into a matrix and a membrane space (Kühlbrandt, 2015). Mitochondria are the “powerhouses” of the cell, providing adenosine triphosphate (ATP) to the organism through oxidative phosphorylation. This process involves a group of enzymes assembled in the mitochondrial electron transport chain to provide energy. Additionally, mitochondria are involved in calcium homeostasis, intracellular reactive oxygen species (ROS) production, intracellular signaling mediation, and regulation of apoptosis (Kanova and Kohout, 2022). Maintaining mitochondrial quality is critical for the functioning of the organism. Therefore, cells have evolved a series of mitochondrial quality maintenance mechanisms to protect normal mitochondrial physiological functions (Ng et al., 2021). However, when any of these factors causes an imbalance in mitochondrial homeostasis, a series of dysfunctions and diseases, such as SAW, can occur (Maestraggi et al., 2017). Mitochondria accumulate copper for assembling copper enzymes, such as cytochrome c oxidase and superoxide dismutase 1 (SOD1). Thus, copper plays an important role in mitochondrial function and signaling related to mitochondrial bioenergetics, kinetics, and autophagy, affecting cell fate through mitochondrial metabolic reprogramming (Ruiz et al., 2021).
The role of copper primarily affects mitochondrial function and metabolism. Copper deficiency reduces activity of the mitochondrial respiratory complex, lowering the metabolic level (Jensen et al., 2019). Conversely, excessive copper accumulation can lead to apoptosis or necrosis (Tarin et al., 2023). Copper overload causes oxidative stress due to excessive accumulation of ROS, resulting in mitochondrial dysfunction and cell death (Yang et al., 2019; Liu et al., 2020a; Kang et al., 2019). In 2022, Tsvetkov et al. demonstrated that excess intracellular copper ions target lipoylated proteins that regulate the mitochondrial TCA cycle, leading to cell death through a mechanism distinct from known forms of regulated cell death (RCD). Interestingly, canonical cell death inhibitors, such as apoptosis, necrosis, pyroptosis, and ferroptosis inhibitors, failed to prevent copper-induced cell death. This new form of cell death is termed “Cuproptosis” (Tsvetkov et al., 2022) and copper is involved in mitochondrial metabolism and plays an important role in maintaining the morphological and functional integrity of mitochondria (Ruiz et al., 2021). The proposition of cuproptosis has advanced the understanding of cell death mechanisms, focusing on its occurrence in mitochondria and its reliance on mitochondrial respiration (Tsvetkov et al., 2022). Previous studies have highlighted the significance of mitochondrial disorders in conditions such as sepsis and ICU-AW (Maestraggi et al., 2017; Li X. et al., 2023; Nedel et al., 2023). In this study, we aim to elucidate the mechanism of cuproptosis in SAW, exploring the relationships between mitochondria, cuproptosis, and SAW, and proposing new ideas for its prevention and treatment.
Copper exhibits strong redox activity and protein binding capacity, serving as a crucial cofactor for key enzymes involved in mitochondrial aerobic respiration, superoxide dismutation, and other vital biological processes. It is an essential trace metal for all living organisms (Huang et al., 2024). Additionally, copper metabolism is closely linked with other trace elements. Copper deficiency can impair iron mobilization, resulting in secondary iron deficiency (Araya et al., 2007), and when copper levels are elevated they exacerbate ferroptosis (Xue et al., 2023). Alterations in trace elements and heavy metal levels have been linked to sepsis. Specifically, elevated serum copper levels in septic patients suggest a potential association between copper and sepsis (Huang et al., 2024).
During sepsis, a decrease in pH due to systemic or localized acidosis can cause the release of copper from cuprocyanin and other carrier proteins, leading to elevated levels of free copper (Carr et al., 2015). Free copper can participate in various biochemical pathways, including the inactivation of activated protein C (APC), stimulation of endothelial cells (ECs), and the production of ROS (Zhou et al., 2022; Bar-Or et al., 2002; He et al., 2020). APC has anticoagulant and anti-inflammatory effects in severe sepsis. APC is generated from inactive protein C through the activation by thrombin in association with platelet regulatory proteins (Annane et al., 2013). However, sepsis impairs the regulation of thrombomodulin by inflammatory cytokines, which hinders the conversion of protein C to APC. Recombinant human APC, on the other hand, reduces serum interleukin-6 and plasma D-dimer levels, thereby improving survival in sepsis patients (Bernard et al., 2001). ECs are the main target of inflammatory mediators in sepsis (Li Y. et al., 2021). ECs contribute to sepsis pathogenesis through the activation of intracellular inflammatory pathways mediated by nuclear factor kappa B (NF-κB) and mitogen-activated protein kinase (MAPK), which are dependent on Toll-like receptors (TLR) (Khakpour et al., 2015).
Furthermore, copper overload damages T- and B-lymphocytes, leading to immunosuppression. Copper also influences apoptosis-regulating molecules in immune organs like the thymus and spleen, thereby inducing apoptosis in immune cells (Mitra et al., 2012). In addition, copper overload can disrupt mitochondrial energy metabolism (Ruiz et al., 2021). Low ATP levels lead to hyperphosphorylation of 5′adenosine monophosphate-activated protein kinase (AMPK), which in turn reduces the activity of mammalian Target of Rapamycin (mTOR). This reduction impairs cellular autophagy, ultimately resulting in compromised protein synthesis in sepsis and damage to skeletal muscle (Shi et al., 2020; Liao et al., 2020). Although copper deficiency affects SOD1 synthesis leading to impaired neurotransmitter release, neuromuscular junction instability and reduced muscle strength in mice (Shi et al., 2014), high intracellular copper concentrations still lead to neurological dysfunction and muscle atrophy (Sakellariou et al., 2014), further impairing neuromuscular function in septic patients. Additionally, several studies have shown that copper induces oxidative stress in cells, leading to muscle atrophy and inhibiting skeletal muscle regeneration (Wang et al., 2018a; Zhao et al., 2018; Wang et al., 2018b), suggesting that copper may play a similar role in SAW.
Interestingly, mitochondria consistently play a central mediating role in copper-induced cell death and sepsis pathogenesis. Although some reports indicate that the mitochondrial respiratory capacity of peripheral blood immune cells in sepsis patients may be increased or unchanged (Sjövall et al., 2013; Merz et al., 2017), other studies have demonstrated that impaired mitochondrial respiration in immune cells, such as macrophages and leukocytes, reduces their energy supply. This reduction in energy exacerbates septic immune paralysis (Weiss et al., 2020; McBride et al., 2020). Mitochondrial oxidative stress modulates copper-induced ECs dysfunction and is involved in sepsis pathogenesis (Zhou et al., 2022; Wang M. et al., 2023; Huet et al., 2009). ROS induces the upregulation of NOD-like receptor family pyrin domain-containing 3 (NLRP3), caspase-1, interleukin-1β (IL-1β), and interleukin-18 (IL-18) in ECs. This upregulation leads to the assembly and activation of the NLRP3 inflammasome through the thioredoxin-interacting protein. Activation of the NLRP3 inflammasome promotes the maturation of IL-1β and IL-18 and facilitates the formation of the pore protein Gasdermin D (GSDMD), which subsequently triggers cellular pyroptosis via the classical inflammasome pathway (Zheng et al., 2022). In addition, ROS-induced DNA damage can lead to hyperactivation of poly-ADP-ribose-polymerase-1 and trigger parthanatos in ECs (Li S. et al., 2022). The role of mitochondrial oxidative stress in sepsis mechanisms has been extensively described (van der Slikke et al., 2021; Bertozzi et al., 2024). Copper, an essential metallic element, plays a crucial role in regulating mitochondrial ROS (Zhou et al., 2022). Thus, mitochondria are key mediators in the pathogenesis of sepsis regulated by copper.
One view is that copper levels are elevated in patients with sepsis (Zhang et al., 2023; Akkaş et al., 2020), and animal studies have shown that inhibition of copper levels attenuates caspase-1 activation and reduces lymphocyte death, which in turn increases survival in models of sepsis (Deigendesch et al., 2018). This suggests that elevated copper levels may promote sepsis progression. However, another view is that copper may have some therapeutic value. During septic infections, the body can enhance antimicrobial function by increasing the release of copper (Huang et al., 2024). Copper selenium nanoclusters can produce synergistic antimicrobial effects for the treatment of mice with sepsis by in situ sulphation of endogenous H2S, triggering ROS bursts and photothermal therapy (Gao et al., 2022). These two views appear contradictory. However, the role of copper is complex and its role at different stages in the pathogenesis of sepsis remain poorly understood. While the body can enhance antimicrobial action during septic infections by increasing the release of copper, copper toxicity results from an elevated copper level, which cannot be controlled by the copper-resistant genes (Rafati Rahimzadeh et al., 2024), resulting in excessive accumulation of intracellular copper and thus inducing different forms of cell death (Huang et al., 2024). Recent studies have shown that cuproptosis occurs in cardiomyocytes during sepsis, which induces cardiotoxicity (Yan et al., 2024); Under these circumstances, does copper-induced cell death play a role in SAW?
Many previous studies have shown that copper-induced cell death is closely associated with ROS and inflammation, which triggers different forms of cell death including apoptosis, necroptosis, pyroptosis and ferroptosis. For example, elevated ROS levels caused by copper overload disrupt mitochondrial membrane permeability, leading to a decrease in mitochondrial membrane potential, which in turn triggers the release of apoptotic proteins, ultimately leading to apoptosis in mouse cells (Liu et al., 2020a). Copper overload activates the ROS/NF-κB signaling pathway and induces impaired mitochondrial autophagy, ultimately leading to pyroptosis (Zhou et al., 2022). Similarly, recent studies have shown that copper exposure induces an inflammatory response and concomitantly induces apoptosis, necroptosis and pyroptosis in mice (Zhao et al., 2024). Ferroptosis is an iron-dependent cell death characterized by disruption of iron homeostasis and lipid peroxidation reactions (Gao et al., 2019). Generally, iron is the inducer of ferroptosis, but interestingly, it was found that elesclomol promotes the degradation of copper ion-transporting ATPase alpha polypeptide (ATP7A). In this case, co-treatment of elesclomol and copper leads to copper retention in mitochondria, and excess copper induces the Fenton reaction leading to accumulation of ROS and enhanced cellular oxidative stress, ultimately leading to ferroptosis (Gao et al., 2021). Furthermore, copper promotes ferroptosis by inducing autophagic degradation of glutathione peroxidase 4 (GPX4) (Xue et al., 2023). However, the different modes of cell death mentioned above have something in common with cuproptosis, in that they can both be caused by copper overload.
In recent years, the proposal of cuproptosis has attracted attention. Cuproptosis, a newly defined form of cell death, is mainly caused by the loss of iron-sulfur (Fe-S) cluster proteins and aggregation of mitochondrial lipoylated proteins induced by intracellular copper overaccumulation, which together lead to proteotoxic stress (Tang et al., 2022). Previous studies have found that copper can interact with proteins and cause protein aggregation, but have not focused on copper-induced cytotoxicity (Weibull et al., 2019). Furthermore, unlike apoptosis, necroptosis, pyroptosis and ferroptosis, cuproptosis occurred independently of ROS production (Li et al., 2023b). As mentioned above, cell death including apoptosis, necroptosis, pyroptosis and ferroptosis can be induced by copper. That would also suggest that cuproptosis interacts with various forms of cell death rather than being independent of them.
The body obtains copper from the diet, which is absorbed by the epithelial cells of the small intestine and transported through the portal vein to the liver, where it enters the bloodstream or is excreted through the bile (Baker et al., 2017). This process involves the uptake and export of copper ions by specific transporter proteins (Maung et al., 2021). Copper is chelated and stored by metallothionein (MT), and excess copper is transported and excreted by specific peptides. Major copper chaperones include the cytochrome c oxidase copper chaperone (COX17), the copper chaperone for SOD1 (CCS) (La et al., 2010). Intracellular copper ions enter the mitochondrial matrix via COX17 and play a relevant role. In turn, copper ions in the cytoplasm bind to chelators, such as glutathione (GSH) and MT, or are taken to SOD1, thereby regulating intracellular ROS levels and copper homeostasis (Xie et al., 2023).
Copper is a cofactor for important enzymes, but excessive accumulation can lead to cellular metabolic disorders and death (Lelièvre et al., 2020). Overloading or depleting metal ions within mitochondria can disrupt their morphology and function, causing cellular damage (Nam et al., 2018). Cytochrome c oxidase (COX) and SOD1 are copper-dependent enzymes (Garza et al., 2023). Copper deficiency hinders COX assembly, reduces ATP production, triggers oxidative stress and exacerbates cell death (Li F. et al., 2022). SOD is an antioxidant defence system and copper supplementation enhances its activity and maintains mitochondrial function. In conclusion, copper is closely related to mitochondrial function (Ruiz et al., 2021; Acetoze et al., 2017; Zeng et al., 2020; Zheng et al., 2023).
Although copper overload has been found to stimulate COX biosynthesis and assembly in the absence of cytotoxicity, resulting in the production of physiological amounts of ROS in the erythroid cell line K562, thereby improving mitochondrial function, copper has also been shown to improve mitochondrial function (Jensen et al., 2019; Ruiz et al., 2016). However, Tsvetkov et al. proposed that cuproptosis is induced by proteotoxic stress caused by an excess of intracellular copper ions. On the one hand, FDX1 encodes a reductase that enhances copper ion toxicity and promotes degradation of iron-sulfur cluster proteins. On the other hand, FDX1/LIAS is an upstream regulator of protein lipoylation and promotes the aggregation of lipoylated mitochondrial enzymes. Together, these aberrant processes trigger proteotoxic stress and cell death (Tsvetkov et al., 2022) (Figure 1). The above studies suggest that cuproptosis involves a copper-triggered mode of mitochondrial cell death, challenging the conventional view that oxidative stress is the underlying molecular mechanism of metal-induced toxicity, thus reinforcing the view that mitochondria are multifaceted regulators of cell death (Tang et al., 2022). Whether the mechanism involves the previously elucidated oxidative stress-induced cytotoxicity mechanism due to copper overload or the hypothesized copper-regulated cell death, it should be noted that mitochondria are always involved.
Figure 1. Mechanism of cuproptosis. Excess intracellular copper targets lipoylated proteins that regulate the mitochondrial tricarboxylic acid (TCA) cycle to induce cell death. Excess copper enters the cell, and ferredoxin 1 (FDX1) reduces copper to toxic monovalent copper ions, which results in the lipoylation and oligomerization of proteins involved in the TCA cycle, as well as iron-sulfur cluster protein loss. Together, these factors lead to proteotoxic stress and induce cuproptosis.
SAW is primarily characterized by skeletal muscle atrophy and weakness, often affecting skeletal and respiratory muscles, and in severe cases, it can lead to quadriplegia (Schefold et al., 2010). Although the pathophysiological mechanism of SAW remains incompletely understood, it is currently thought to be associated with skeletal muscle mitochondrial dysfunction (Maestraggi et al., 2017; Mankowski et al., 2021; Chatre et al., 2017). Damaged mitochondria fuse with healthy mitochondria to rescue impaired function, thereby preserving the overall physiological function of the mitochondrial network (Gustafsson and Dorn, 2019). However, in sepsis, healthy mitochondria decrease in the diaphragm muscle cells, leading to a reduced level of mitochondrial fusion marker mRNA and increased levels of non-functional small optic atrophy one protein isoforms. This inhibits mitochondrial fusion, resulting in dysfunction of the diaphragm (Wai et al., 2015; Oliveira et al., 2021). Reduction in mitochondria has been linked to an increased release of mitochondrial degradation products and damage-associated molecular patterns, which interact with their receptors, thereby stimulating the secretion of inflammatory factors in peripheral cells (Mankowski et al., 2021; Zhang et al., 2010). Conversely, the prolonged inflammatory process of sepsis increases tumor necrosis factor α production, leading to reduced mitochondrial respiration and impaired mitochondria biosynthesis, in mouse skeletal muscle (Mofarrahi et al., 2012). Additionally, mitochondrial respiratory complexes were dysfunctional and quantitatively defective in muscle samples from patients with sepsis compared to healthy controls, further confirming the association between mitochondrial dysfunction and SAW (Jiroutková et al., 2015). Tsvetkov et al. have demonstrated that mitochondria play a role in copper ion-overload-induced cell death (Tsvetkov et al., 2022). Thus, copper may regulate SAW by disrupting mitochondrial homeostasis.
The intracellular accumulation of copper ions is the key to copper-induced cell death, which is dependent on the regulation of mitochondrial respiration. Cells that are dependent on mitochondrial respiration are almost 1,000 times more sensitive to copper ions than cells dependent on glycolysis (Tsvetkov et al., 2022). Approximately two-thirds of the copper in the human body is found in bone and muscle, and skeletal muscle is rich in mitochondrial ions (Liu Y. et al., 2022). ATP produced by mitochondrial respiration provides energy for skeletal muscle movement (Hood et al., 2019). In addition, severe mitochondrial damage is observed in the skeletal muscle of patients with sepsis and multiple organ failure in the ICU, and the muscle ATP concentration is significantly reduced (Brealey et al., 2002). A study has confirmed that septic cardiotoxicity is associated with cuproptosis (Yan et al., 2024). Thus, cuproptosis may play a role in SAW through impaired mitochondrial function.
Serine/threonine kinase (AKT) is involved in mitochondria-mediated apoptosis, oxidation-reduction state, dynamic homeostasis, autophagy, and metabolism (Xie et al., 2022). Copper accumulation significantly reduces antioxidant enzyme activity, disrupts mitochondrial dynamics, and inhibits the phosphatidylinositol-3-kinase/AKT/mTOR (PI3K/AKT/mTOR) pathway in chicken skeletal muscle (Wang et al., 2018b). Notably, the PI3K/AKT/mTOR pathway is inhibited by copper accumulation, which induces septic skeletal muscle atrophy (Yin et al., 2022) and atrophy of the diaphragm (Wu et al., 2019). Members of the forkhead box O (FOXO) family are downstream targets of AKT (Farhan et al., 2017; Zhang and Zhang, 2019), and the FOXO pathway also play a role in SAW. For example, copper-induced cell death is associated with increased expression of FOXO1, FOXO3 and FOXO4 (Zeng et al., 2022; Hassani et al., 2018), which induces mitochondrial dysfunction by triggering mitochondrial dynamics and excessive autophagy (Chen et al., 2023). In addition, FOXO1 is an important factor in proteolysis, and increased FOXO1 expression during sepsis is a key factor that causes SAW (Smith et al., 2010; Castillero et al., 2013).This suggests that copper-induced cell death may be involved in the pathomechanism of SAW through the FOXO-related signaling pathway. Excess copper activates the Janus kinase/signal transducer and activator of transcription (JAK-STAT) pathway (Deng et al., 2023), and JAK-STAT activation induces mitochondrial autophagy and upregulates suppressor of cytokine signaling 3 (SOCS 3) expression, which in turn inhibits AKT expression through an insulin-dependent pathway, ultimately leading to SAW (Zanders et al., 2022; Huang et al., 2020). In addition, energy metabolism plays an important role in SAW (Jiang et al., 2022). NF-κB is involved in energy metabolism by regulating glycolytic utilization and mitochondrial respiratory homeostasis (Vander Heiden et al., 2009). Copper accumulation also activates the NF-κB pathway (Deng et al., 2023; Liu et al., 2020b), which induces an increase in SPRY domain-containing SOCS box protein 1 (SPSB1) and has been found to lead to muscle atrophy and weakness in mice with sepsis (Li et al., 2023c). AMPK is a critical cellular energy sensor that regulates cellular metabolism and maintains energy homeostasis (Herzig and Shaw, 2018). Under energy stress, AMPK enhances the mitochondrial ATP production pathway and reduces ATP consumption pathways, to reduce unnecessary energy consumption (Hardie, 2011). Therefore, AMPK activation is an adaptive mechanism in sepsis (Jin et al., 2020). Interestingly, excess copper activates the AMPK-mTOR signaling pathway, and activation of AMPK inhibits mTOR expression, leading to impaired mitochondrial function and ultimately cell death (Lei et al., 2021). Thus, copper may be involved in the activation of AMPK in sepsis and play a role in SAW. Therefore, copper may induce SAW through mitochondria-related pathways such as PI3K/AKT/mTOR, FOXO, JAK-STAT, NF-κB, AMPK-mTOR, and others (Figure 2).
Figure 2. Mechanism of copper overload leading to SAW. Intracellular copper overload induces mitochondrial dysfunction and thus regulates SAW through multiple signaling pathways.
Ferroptosis, is a form of iron-dependent cell death that is induced by the overaccumulation of iron and lipid peroxides. Mitochondria, the central organelles responsible for iron metabolism and energy production, play a key role in ferroptosis (Gao et al., 2019). Moreover, cuproptosis also occurs within mitochondria, and the onset of cuproptosis is accompanied by disruption of mitochondrial morphology (Cobine and Brady, 2022). Mitochondrial respiration and mitochondrial enzymes play key roles in regulating cuproptosis (Tang et al., 2022).Several recent studies have demonstrated the existence of crosstalk between the mechanisms of cuproptosis and ferroptosis. Elesclomol as a copper ion carrier promotes the degradation of ATP7A. In this case, co-treatment of elesclomol and copper leads to copper retention in mitochondria, and excess copper induces the Fenton reaction, leading to accumulation of ROS and enhanced cellular oxidative stress, and ultimately to ferroptosis (Gao et al., 2021). Furthermore, ferroptosis inducers inhibit mitochondrial matrix-associated protease-mediated degradation of ferredoxin 1 (FDX1), which in turn upregulates protein lipidation and induces cuproptosis by reducing the intracellular synthesis of the copper chelator GSH via the inhibition of cystine production (Wang W. et al., 2023). Whereas mitochondria play a role in the crosstalk between cuproptosis and ferroptosis. Specifically, it is manifested in the mitochondrial TCA cycle. Studies have demonstrated the necessity of the TCA cycle and glutamine catabolism in ferroptosis, and blockade of the TCA cycle or glutamine deficiency ameliorates ferroptosis induced by cystine depletion or erastin (Gao et al., 2019). FDX1, a key factor in cuproptosis, promotes lipoylation of the mitochondrial enzyme DLAT thereby affecting the TCA cycle (Tang et al., 2022). In addition, GSH not only acts as a common inhibitor of ferroptosis and cuproptosis, but also participates in the regulation of the mitochondrial TCA cycle (Liu and Chen, 2024). The tumor suppressor p53 is an important metabolic regulator, p53 not only promotes mitochondrial TCA cycling but also plays an important role in cuproptosis (Zhang et al., 2023). In different cellular environments, p53 is thought to promote or inhibit ferroptosis (Akkaş et al., 2020; Deigendesch et al., 2018). The above studies have revealed that cuproptosis and ferroptosis have similarities, with strong associations between the mechanisms of cuproptosis and ferroptosis. Importantly, ferroptosis is involved in sepsis-induced muscle atrophy and weakness and that STAT6 inhibition significantly reduces mitochondrial dysfunction and thus rescues ferroptosis in the skeletal muscle of mice with sepsis (Sheng et al., 2024).This suggests that cuproptosis may induce SAW through the mitochondrial dysfunction mechanisms that have been associated with ferroptosis. This also suggests that copper homeostatic imbalance exacerbates ferroptosis, which in turn is involved in the induction of cuproptosis, which in turn leads to SAW (Figure 3).
Figure 3. Mechanisms of cuproptosis and ferroptosis in the SAW. Ferroptosis and cuproptosis may crosstalk with each other and induce SAW by impairing mitochondrial function. Ferroptosis inducers (FINs), Sorafenib (Sora) and Erastin (Era) promote cuproptosis induced by copper ion carriers (CINS) by stabilizing the ferredoxin 1 (FDX1) protein and depleting the intracellular glutathione (GSH) level. Mechanistically, FIN stabilizes FDX1 by inhibiting mitochondrial proteases. Stabilized FDX1 enhances protein-lipid acylation and facilitates the transfer of the reduced copper ion, Cu+. In addition, FINs inhibit cystine input by inhibiting the Xc-system, which leads to a lower GSH level and thus a higher copper ion concentration. Together, these factors enhance the aggregation of lipoylated proteins, thereby promoting cellular cuproptosis. Elesclomol decreases the expression of ATP7A, resulting in intracellular retention of copper, which in turn leads to ROS accumulation. This effect promotes the degradation of SLC7A11, which further enhance oxidative stress and ultimately lead to ferroptosis. This suggests that ferroptosis and cuproptosis may crosstalk with each other and induce SAW by impairing mitochondrial function.
A genome-wide Clustered Regularly Interspaced Palindromic Repeats (CRISPR)/CRISPR-associated protein nine screen revealed that cuproptosis was highly associated with 10 genes: FDX1, LIPT1, LIAS, DLD, DLAT, PDHA1, PDHB, MTF1, GLS, and CDKN2A (Tsvetkov et al., 2022).
Notably, DLAT, DLD, PDHA1, and PDHB are subunits of the pyruvate dehydrogenase complex (PDC) (Pavlu-Pereira et al., 2020). Mitochondrial PDC irreversibly decarboxylates pyruvate to acetyl coenzyme A, which feeds the mitochondrial TCA cycle (Stacpoole, 2017) and plays an important role in mitochondrial aerobic respiration (Park et al., 2018). Decreased PDC activity in the skeletal muscle of rats with sepsis leads to myocyte hypoxia and skeletal muscle dysfunction (Vary, 1996). Furthermore, FDX1 is a key factor in copper-induced cell death and is involved in the formation of Fe-S cluster proteins, which are essential for mitochondrial function (Cai et al., 2017). Upregulation of FDX1 leads to elevated cellular ROS and ferrous iron overload, which in turn induces ferroptosis, due to the continuous accumulation of lipid peroxides (Li L. et al., 2023). Importantly, ferroptosis has been shown to be involved in the development of SAW, suggesting that FDX1 may also be involved in the regulation of SAW (Sheng et al., 2024).
A bioinformatic analysis of the relationship between the pathogenesis of sepsis and cuproptosis-related genes identified LIAS and PDHB as potential diagnostic biomarkers for cuproptosis-associated sepsis (Sun et al., 2024). LIAS is responsible for encoding components in the lipoic acid pathway and for synthesizing the potent mitochondrial antioxidant α-lipoic acid (LA) (Krishnamoorthy et al., 2017). Furthermore, previous studies have shown that LIAS plays a key role in maintaining redox and mitochondrial homeostasis in sepsis (Sun et al., 2024). Cuproptosis-related genes are closely associated with sarcopenia and that the upregulation of LIAS gene expression promotes LA accumulation in mitochondria, thereby reducing oxidative damage in skeletal muscle (Lin et al., 2022). Therefore, increasing LIAS expression may rescue SAW by facilitating the maintenance of mitochondrial homeostasis.
Pyruvate Dehydrogenase E1 Subunit Beta (PDHB) protein is a key enzyme of the mitochondrial TCA cycle, which plays a crucial role in cellular energy metabolism by acting on acetyl coenzyme A and promoting its entry into the TCA cycle, ultimately producing ATP (Lee and Banerjee, 2020). Downregulation of PDHB is strongly associated with development of sepsis (Sun et al., 2024). PDHB is considered to be a protective factor for muscle tissue that promotes myogenic differentiation and improves muscle function by inhibiting the forkhead box P1-ariadne two homolog axis (Jiang et al., 2023). Therefore, targeting PDHB, a key gene for cuproptosis, may be an important means of treating SAW.
High mobility group protein B1 (HMGB1) is a pro-inflammatory factor that induces a lethal systemic inflammatory response in the late stages of sepsis, and targeting HMGB1 may reduce the impact of sepsis and the associated organ damage (Deng et al., 2022). HMGB1 induces mitochondrial oxidative damage (Dong et al., 2015), whereas HMGB1 inhibition activates the AMPK pathway and attenuates mitochondrial dysfunction (Liu et al., 2021). Copper accumulation-induced ATP depletion activates AMPK to promote HMGB1 phosphorylation, which leads to an increased extracellular release of HMGB1. HMGB one release precedes elesclomol-copper induced cell death, which suggests that HMGB1 may serve as a sensitive predictor of early cuproptosis (Liu J. et al., 2022). In addition, HMGB1 inhibition rescues muscle atrophy caused by cachexia (Li L. et al., 2021); therefore, cuproptosis through HMGB1 may be a key factor in sepsis-induced SAW.
In addition to mitochondria, relationships exist between copper and SAW. For example, copper directly binds to GPX4 proteins, leading to the formation of GPX4 aggregates and subsequent autophagic degradation of GPX4, which in turn induces ferroptosis (Xue et al., 2023). As discussed above, ferroptosis is involved in SAW (Sheng et al., 2024), and therefore, copper may be indirectly involved in SAW by directly inducing ferroptosis. GSH is a co-regulator of ferroptosis and cuproptosis and acts as an antioxidant in ferroptosis by hindering lipid peroxidation, thereby inhibiting cell death (Ursini and Maiorino, 2020). However, in cuproptosis, GSH acts as a copper ion chaperone to chelate copper ions, attenuates the aggregation of fatty acylated proteins, and inhibits cell death induced by excessive intracellular copper ions (Tsvetkov et al., 2022). Glutamine (Gln) depletion in skeletal muscle is significant in patients in the ICU after severe trauma and sepsis, and Gln supplementation is protective against sepsis-induced skeletal muscle injury (Hou et al., 2021). While Gln is a precursor of GSH, Gln deficiency causes a decrease in GSH concentration (Matés et al., 2020). As a key inhibitor of cuproptosis, a decrease in GSH concentration provides an opportunity for cuproptosis to occur. In addition, when there is severe Gln deficiency, muscle atrophy occurs (Cruzat et al., 2018), which further strengthens the correlation between cuproptosis and SAW. In addition, a recent study has shown that N-acetylcysteine (NAC) alleviates sepsis-induced muscle atrophy by downregulating endoplasmic reticulum stress (Chen et al., 2024). Interestingly, as a precursor of GSH, NAC functions not only to regulate redox homeostasis but also to chelate copper ions to alleviate copper toxicity (Wolfram et al., 2020) (Table 1).
Copper has been reported to have a partial bactericidal effect in the treatment of septicemia (Huang et al., 2024; Gao et al., 2022). However, it has also been claimed that copper induces interleukin-8 expression and plays a role in early inflammation in sepsis (Bar-Or et al., 2003). Furthermore, cuproptosis has been shown to be involved in septic myocardial injury (Yan et al., 2024), thus it is possible to speculate that sepsis promotes cuproptosis in skeletal muscle cells, inducing SAW.
The copper level is elevated in humans with sepsis, but animal studies suggest that copper may have some therapeutic effect. One possible explanation is that copper, although it has some antimicrobial properties, has a limited role in counteracting the systemic inflammatory response of sepsis, leading to a constant release of copper from the organism; however, when the copper level exceeds the threshold that the organism can tolerate, cytotoxicity is exacerbated, which can lead to cell death. This cell death may coincide with the onset of cuproptosis; after all, overloading the body with copper levels also provides an opportunity for cuproptosis to occur. And the mechanism of cuproptosis is likely to accompany the development of sepsis and SAW.
The role of copper in sepsis and cell death has been extensively studied, but its role in copper-induced cell death in SAW remains poorly understood. This paper reviews how copper overload-induced cuproptosis may contribute to SAW pathogenesis through mitochondria-related pathways, including signaling pathways (such as PI3K/AKT/mTOR, FOXO, JAK-STAT, NF-κB, AMPK-mTOR), cuproptosis-related genes (like DLAT, DLD, PDHA1, PDHB, FDX1, LIAS, HMGB1, NAC), and ferroptosis. As a newly identified mode of cell death, the regulatory pathways of cuproptosis in SAW require further investigation. Current studies on cuproptosis largely rely on bioinformatics, however, the specific regulatory mechanisms regarding cuproptosis in sepsis as well as in skeletal muscle cells are not known. More cellular and animal experiments are urgently needed to complement this to clarify its relationship with SAW.
Importantly, cell death does not occur through isolated pathways; rather, various cell death pathways interact to induce cell death at different stages of sepsis. Mitochondria play a crucial role in regulating cell death across multiple pathways. Future research should use mitochondria as a focus to explore the mechanisms of cuproptosis in SAW, particularly concerning mitochondrial oxidative stress, energy metabolism, and the TCA cycle. These insights could offer new targets for understanding SAW mechanisms and for the diagnosis and treatment of patients.
LY: Writing–original draft, Writing–review and editing. LX: Writing–original draft, Writing–review and editing. ML: Writing–original draft, Writing–review and editing. YM: Writing–original draft, Writing–review and editing. JY: Writing–original draft, Writing–review and editing. SC: Writing–original draft, Writing–review and editing. XM: Writing–original draft, Writing–review and editing. PX: Writing–original draft, Writing–review and editing.
The author(s) declare that financial support was received for the research and/or publication of this article. This work was supported by the National Natural Science Foundation (Grant Nos.82060359 and 82360382) of China; Guizhou Province Social Development Project: Qiankehe [2021] General 088; Key Project of Guizhou Natural Science Foundation: Qiankehe Fundamentals ZK [2022] Key 049; Guizhou Province Excellent Youth Science and Technology Talent Project: Qiankehe Platform Talent [2021] No. 5648.
The authors thank Xiaoming Zhang for assistance in reviewing and proofreading this paper. The authors acknowledge the use of Figdraw (www.figdraw.com) to create the figures.
The authors declare that the research was conducted in the absence of any commercial or financial relationships that could be construed as a potential conflict of interest.
The authors declare that no Generative AI was used in the creation of this manuscript.
All claims expressed in this article are solely those of the authors and do not necessarily represent those of their affiliated organizations, or those of the publisher, the editors and the reviewers. Any product that may be evaluated in this article, or claim that may be made by its manufacturer, is not guaranteed or endorsed by the publisher.
Acetoze G., Champagne J., Ramsey J. J., Rossow H. A. (2017). Liver mitochondrial oxygen consumption and efficiency of milk production in lactating Holstein cows supplemented with copper, manganese and zinc. J. Animal Physiology Animal Nutr. 102 (2), e787–e797. doi:10.1111/jpn.12836
Akkaş İ., Ince N., Sungur M. A. (2020). Serum trace element and heavy metal levels in patients with sepsis. Aging Male 23 (3), 222–226. doi:10.1080/13685538.2020.1740200
Annane D., Timsit J.-F., Megarbane B., Martin C., Misset B., Mourvillier B., et al. (2013). Recombinant human activated protein C for adults with septic shock. A randomized controlled trial. Am. J. Respir. Crit. care Med. 187 (10), 1091–1097. doi:10.1164/rccm.201211-2020OC
Araya M., Olivares M., Pizarro F. (2007). Copper in human health. Int. J. Environ. Health 1 (4), 608–620. doi:10.1504/ijenvh.2007.018578
Baker Z. N., Cobine P. A., Leary S. C. (2017). The mitochondrion: a central architect of copper homeostasis. Metallomics 9 (11), 1501–1512. doi:10.1039/c7mt00221a
Bar-Or D., Rael L. T., Winkler J. V., Yukl R. L., Thomas G. W., Shimonkevitz R. P. (2002). Copper inhibits activated protein C: protective effect of human albumin and an analogue of its high-affinity copper-binding site, d-DAHK. Biochem. biophysical Res. Commun. 290 (5), 1388–1392. doi:10.1006/bbrc.2002.6363
Bar-Or D., Thomas G. W., Yukl R. L., Rael L. T., Shimonkevitz R. P., Curtis C. G., et al. (2003). Copper stimulates the synthesis and release of interleukin-8 in human endothelial cells: a possible early role in systemic inflammatory responses. Shock 20 (2), 154–158. doi:10.1097/01.shk.0000068318.49350.3a
Bellaver P., Schaeffer A. F., Leitao C. B., Rech T. H., Nedel W. L. (2023). Association between neuromuscular blocking agents and the development of intensive care unit-acquired weakness (ICU-AW): a systematic review with meta-analysis and trial sequential analysis. Anaesth. Crit. Care Pain Med. 42 (3), 101202. doi:10.1016/j.accpm.2023.101202
Bernard G. R., Vincent J.-L., Laterre P.-F., LaRosa S. P., Dhainaut J.-F., Lopez-Rodriguez A., et al. (2001). Efficacy and safety of recombinant human activated protein C for severe sepsis. N. Engl. J. Med. 344 (10), 699–709. doi:10.1056/NEJM200103083441001
Bertozzi G., Ferrara M., Di Fazio A., Maiese A., Delogu G., Di Fazio N., et al. (2024). Oxidative stress in sepsis: a focus on cardiac pathology. Int. J. Mol. Sci. 25 (5), 2912. doi:10.3390/ijms25052912
Brealey D., Brand M., Hargreaves I., Heales S., Land J., Smolenski R., et al. (2002). Association between mitochondrial dysfunction and severity and outcome of septic shock. Lancet 360 (9328), 219–223. doi:10.1016/S0140-6736(02)09459-X
Cai K., Tonelli M., Frederick R. O., Markley J. L. (2017). Human mitochondrial ferredoxin 1 (FDX1) and ferredoxin 2 (FDX2) both bind cysteine desulfurase and donate electrons for iron-sulfur cluster biosynthesis. Biochemistry 56 (3), 487–499. doi:10.1021/acs.biochem.6b00447
Carr A. C., Shaw G. M., Fowler A. A., Natarajan R. (2015). Ascorbate-dependent vasopressor synthesis: a rationale for vitamin C administration in severe sepsis and septic shock? Crit. Care 19, 418–8. doi:10.1186/s13054-015-1131-2
Castillero E., Alamdari N., Aversa Z., Gurav A., Hasselgren P. O. (2013). PPARβ/δ regulates glucocorticoid- and sepsis-induced FOXO1 activation and muscle wasting. PLoS One 8 (3), e59726. doi:10.1371/journal.pone.0059726
Chatre L., Verdonk F., Rocheteau P., Crochemore C., Chrétien F., Ricchetti M. (2017). A novel paradigm links mitochondrial dysfunction with muscle stem cell impairment in sepsis. Biochim. Biophys. Acta Mol. Basis Dis. 1863 (10 Pt B), 2546–2553. doi:10.1016/j.bbadis.2017.04.019
Chen H., Zhao C., Wei Y., Jin J. (2019). Early lactate measurement is associated with better outcomes in septic patients with an elevated serum lactate level. Crit. Care 23 (1), 351. doi:10.1186/s13054-019-2625-0
Chen R., Zheng Y., Zhou C., Dai H., Wang Y., Chu Y., et al. (2024). N-acetylcysteine attenuates sepsis-induced muscle atrophy by downregulating endoplasmic reticulum stress. Biomedicines 12 (4), 902. doi:10.3390/biomedicines12040902
Chen X., Ji Y., Liu R., Zhu X., Wang K., Yang X., et al. (2023). Mitochondrial dysfunction: roles in skeletal muscle atrophy. J. Transl. Med. 21 (1), 503. doi:10.1186/s12967-023-04369-z
Cobine P. A., Brady D. C. (2022). Cuproptosis: cellular and molecular mechanisms underlying copper-induced cell death. Mol. Cell 82 (10), 1786–1787. doi:10.1016/j.molcel.2022.05.001
Cruzat V., Macedo Rogero M., Noel Keane K., Curi R., Newsholme P. (2018). Glutamine: metabolism and immune function, supplementation and clinical translation. Nutrients 10 (11), 1564. doi:10.3390/nu10111564
Deigendesch N., Zychlinsky A., Meissner F. (2018). Copper regulates the canonical NLRP3 inflammasome. J. Immunol. 200 (5), 1607–1617. doi:10.4049/jimmunol.1700712
Deng C., Zhao L., Yang Z., Shang J. J., Wang C. Y., Shen M. Z., et al. (2022). Targeting HMGB1 for the treatment of sepsis and sepsis-induced organ injury. Acta Pharmacol. Sin. 43 (3), 520–528. doi:10.1038/s41401-021-00676-7
Deng H., Zhu S., Yang H., Cui H., Guo H., Deng J., et al. (2023). The dysregulation of inflammatory pathways triggered by copper exposure. Biol. Trace Elem. Res. 201 (2), 539–548. doi:10.1007/s12011-022-03171-0
Dong W. W., Liu Y. J., Lv Z., Mao Y. F., Wang Y. W., Zhu X. Y., et al. (2015). Lung endothelial barrier protection by resveratrol involves inhibition of HMGB1 release and HMGB1-induced mitochondrial oxidative damage via an Nrf2-dependent mechanism. Free Radic. Biol. Med. 88 (Pt B), 404–416. doi:10.1016/j.freeradbiomed.2015.05.004
Farhan M., Wang H., Gaur U., Little P. J., Xu J., Zheng W. (2017). FOXO signaling pathways as therapeutic targets in cancer. Int. J. Biol. Sci. 13 (7), 815–827. doi:10.7150/ijbs.20052
Gao M., Yi J., Zhu J., Minikes A. M., Monian P., Thompson C. B., et al. (2019). Role of mitochondria in ferroptosis. Mol. Cell 73 (2), 354–363. doi:10.1016/j.molcel.2018.10.042
Gao W., Huang Z., Duan J., Nice E. C., Lin J., Huang C. (2021). Elesclomol induces copper-dependent ferroptosis in colorectal cancer cells via degradation of ATP7A. Mol. Oncol. 15 (12), 3527–3544. doi:10.1002/1878-0261.13079
Gao Y., Wang Z., Li Y., Yang J., Liao Z., Liu J., et al. (2022). A rational design of copper–selenium nanoclusters that cures sepsis by consuming endogenous H 2 S to trigger photothermal therapy and ROS burst. Biomaterials Sci. 10 (12), 3137–3157. doi:10.1039/d2bm00172a
Garza N. M., Swaminathan A. B., Maremanda K. P., Zulkifli M., Gohil V. M. (2023). Mitochondrial copper in human genetic disorders. Trends Endocrinol. and Metabolism 34 (1), 21–33. doi:10.1016/j.tem.2022.11.001
Gustafsson Å. B., Dorn G. W. (2019). Evolving and expanding the roles of mitophagy as a homeostatic and pathogenic process. Physiol. Rev. 99 (1), 853–892. doi:10.1152/physrev.00005.2018
Hardie D. G. (2011). Energy sensing by the AMP-activated protein kinase and its effects on muscle metabolism. Proc. Nutr. Soc. 70 (1), 92–99. doi:10.1017/S0029665110003915
Hassani S., Ghaffari P., Chahardouli B., Alimoghaddam K., Ghavamzadeh A., Alizadeh S., et al. (2018). Disulfiram/copper causes ROS levels alteration, cell cycle inhibition, and apoptosis in acute myeloid leukaemia cell lines with modulation in the expression of related genes. Biomed. Pharmacother. 99, 561–569. doi:10.1016/j.biopha.2018.01.109
He H., Zou Z., Wang B., Xu G., Chen C., Qin X., et al. (2020). Copper oxide nanoparticles induce oxidative DNA damage and cell death via copper ion-mediated P38 MAPK activation in vascular endothelial cells. Int. J. nanomedicine 15, 3291–3302. doi:10.2147/IJN.S241157
Herzig S., Shaw R. J. (2018). AMPK: guardian of metabolism and mitochondrial homeostasis. Nat. Rev. Mol. Cell Biol. 19 (2), 121–135. doi:10.1038/nrm.2017.95
Hood D. A., Memme J. M., Oliveira A. N., Triolo M. (2019). Maintenance of skeletal muscle mitochondria in health, exercise, and aging. Annu. Rev. Physiol. 81, 19–41. doi:10.1146/annurev-physiol-020518-114310
Hou Y.-C., Pai M.-H., Wu J.-M., Yang P.-J., Lee P.-C., Chen K.-Y., et al. (2021). Protective effects of glutamine and leucine supplementation on sepsis-induced skeletal muscle injuries. Int. J. Mol. Sci. 22 (23), 13003. doi:10.3390/ijms222313003
Huang Z., Cao L., Yan D. (2024). Inflammatory immunity and bacteriological perspectives: a new direction for copper treatment of sepsis. J. Trace Elem. Med. Biol. 84, 127456. doi:10.1016/j.jtemb.2024.127456
Huang Z., Zhong L., Zhu J., Xu H., Ma W., Zhang L., et al. (2020). Inhibition of IL-6/JAK/STAT3 pathway rescues denervation-induced skeletal muscle atrophy. Ann. Transl. Med. 8 (24), 1681. doi:10.21037/atm-20-7269
Huet O., Harrois A., Duranteau J. (2009). Oxidative stress and endothelial dysfunction during sepsis. Yearb. Intensive Care Emerg. Med., 59–64. doi:10.1007/978-3-540-92276-6_6
Jensen E. L., Gonzalez-Ibanez A. M., Mendoza P., Ruiz L. M., Riedel C. A., Simon F., et al. (2019). Copper deficiency-induced anemia is caused by a mitochondrial metabolic reprograming in erythropoietic cells. Metallomics 11 (2), 282–290. doi:10.1039/c8mt00224j
Jiang X., Ji S., Yuan F., Li T., Cui S., Wang W., et al. (2023). Pyruvate dehydrogenase B regulates myogenic differentiation via the FoxP1-Arih2 axis. J. Cachexia Sarcopenia Muscle 14 (1), 606–621. doi:10.1002/jcsm.13166
Jiang Y., Wei Q., Liu W., Chen Q., Chen X., Yuan Z., et al. (2022). Exploring the muscle metabolomics in the mouse model of sepsis-induced acquired weakness. Evid. Based Complement. Altern. Med. 2022, 6908488. doi:10.1155/2022/6908488
Jin K., Ma Y., Manrique-Caballero C. L., Li H., Emlet D. R., Li S., et al. (2020). Activation of AMP-activated protein kinase during sepsis/inflammation improves survival by preserving cellular metabolic fitness. Faseb J. 34 (5), 7036–7057. doi:10.1096/fj.201901900R
Jiroutková K., Krajčová A., Ziak J., Fric M., Waldauf P., Džupa V., et al. (2015). Mitochondrial function in skeletal muscle of patients with protracted critical illness and ICU-acquired weakness. Crit. Care 19, 448. doi:10.1186/s13054-015-1160-x
Kang Z., Qiao N., Liu G., Chen H., Tang Z., Li Y. (2019). Copper-induced apoptosis and autophagy through oxidative stress-mediated mitochondrial dysfunction in male germ cells. Toxicol Vitro 61, 104639. doi:10.1016/j.tiv.2019.104639
Kanova M., Kohout P. (2022). Molecular mechanisms underlying intensive care unit-acquired weakness and sarcopenia. Int. J. Mol. Sci. 23 (15), 8396. doi:10.3390/ijms23158396
Khakpour S., Wilhelmsen K., Hellman J. (2015). Vascular endothelial cell Toll-like receptor pathways in sepsis. Innate Immun. 21 (8), 827–846. doi:10.1177/1753425915606525
Klawitter F., Ehler J., Bajorat R., Patejdl R. (2023). Mitochondrial dysfunction in intensive care unit-acquired weakness and critical illness myopathy: a narrative review. Int. J. Mol. Sci. 24 (6), 5516. doi:10.3390/ijms24065516
Krishnamoorthy E., Hassan S., Hanna L. E., Padmalayam I., Rajaram R., Viswanathan V. (2017). Homology modeling of Homo sapiens lipoic acid synthase: Substrate docking and insights on its binding mode. J. Theor. Biol. 420, 259–266. doi:10.1016/j.jtbi.2016.09.005
Kühlbrandt W. (2015). Structure and function of mitochondrial membrane protein complexes. BMC Biol. 13, 89. doi:10.1186/s12915-015-0201-x
La F. S., Ackland M. L., Mercer J. F. (2010). Mammalian copper-transporting P-type ATPases, ATP7A and ATP7B: emerging roles. Int. J. Biochem. Cell Biol. 42 (2), 206–209. doi:10.1016/j.biocel.2009.11.007
Latronico N. (2016). Critical illness polyneuropathy and myopathy 20 years later. No man’s land? No, it is our land. Intensive Care Med. 42 (11), 1790–1793. doi:10.1007/s00134-016-4475-4
Lee J., Banerjee D. (2020). Metabolomics and the microbiome as biomarkers in sepsis. Crit. Care Clin. 36 (1), 105–113. doi:10.1016/j.ccc.2019.08.008
Lei C., Liao J., Li Q., Shi J., Zhang H., Guo J., et al. (2021). Copper induces mitochondria-mediated apoptosis via AMPK-mTOR pathway in hypothalamus of Pigs. Ecotoxicol. Environ. Saf. 220, 112395. doi:10.1016/j.ecoenv.2021.112395
Lelièvre P., Sancey L., Coll J.-L., Deniaud A., Busser B. (2020). The multifaceted roles of copper in cancer: a trace metal element with dysregulated metabolism, but also a target or a bullet for therapy. Cancers 12 (12), 3594. doi:10.3390/cancers12123594
Li F., Wu X., Liu H., Liu M., Yue Z., Wu Z., et al. (2022b). Copper depletion strongly enhances ferroptosis via mitochondrial perturbation and reduction in antioxidative mechanisms. Antioxidants 11 (11), 2084. doi:10.3390/antiox11112084
Li L., Liu H., Tao W., Wen S., Fu X., Yu S. (2021b). Pharmacological inhibition of HMGB1 prevents muscle wasting. Front. Pharmacol. 12, 731386. doi:10.3389/fphar.2021.731386
Li L., Pei Z., Wu R., Zhang Y., Pang Y., Hu H., et al. (2023d). FDX1 regulates leydig cell ferroptosis mediates PM(2.5)-induced testicular dysfunction of mice. Ecotoxicol. Environ. Saf. 263, 115309. doi:10.1016/j.ecoenv.2023.115309
Li S., Deng J., Sun D., Chen S., Yao X., Wang N., et al. (2022a). FBXW7 alleviates hyperglycemia-induced endothelial oxidative stress injury via ROS and PARP inhibition. Redox Biol. 58, 102530. doi:10.1016/j.redox.2022.102530
Li X., Sun B., Li J., Ye W., Li M., Guan F., et al. (2023a). Sepsis leads to impaired mitochondrial calcium uptake and skeletal muscle weakness by reducing the micu1:mcu protein ratio. Shock 60 (5), 698–706. doi:10.1097/SHK.0000000000002221
Li Y., Dörmann N., Brinschwitz B., Kny M., Martin E., Bartels K., et al. (2023c). SPSB1-mediated inhibition of TGF-β receptor-II impairs myogenesis in inflammation. J. Cachexia Sarcopenia Muscle 14 (4), 1721–1736. doi:10.1002/jcsm.13252
Li Y., Du Y., Zhou Y., Chen Q., Luo Z., Ren Y., et al. (2023b). Iron and copper: critical executioners of ferroptosis, cuproptosis and other forms of cell death. Cell Commun. Signal. 21 (1), 327. doi:10.1186/s12964-023-01267-1
Li Y., Suo L., Fu Z., Li G., Zhang J. (2021a). Pivotal role of endothelial cell autophagy in sepsis. Life Sci. 276, 119413. doi:10.1016/j.lfs.2021.119413
Liao J., Yang F., Yu W., Qiao N., Zhang H., Han Q., et al. (2020). Copper induces energy metabolic dysfunction and AMPK-mTOR pathway-mediated autophagy in kidney of broiler chickens. Ecotoxicol. Environ. Saf. 206, 111366. doi:10.1016/j.ecoenv.2020.111366
Lin S., Huang H., Ling M., Zhang C., Yang F., Fan Y. (2022). Development and validation of a novel diagnostic model for musculoskeletal aging (sarcopenia) based on cuproptosis-related genes associated with immunity. Am. J. Transl. Res. 14 (12), 8523–8538.
Liu B., Gan X., Zhao Y., Gao J., Yu H. (2021). Inhibition of HMGB1 reduced high glucose-induced BMSCs apoptosis via activation of AMPK and regulation of mitochondrial functions. J. Physiol. Biochem. 77 (2), 227–235. doi:10.1007/s13105-021-00784-2
Liu H., Guo H., Deng H., Cui H., Fang J., Zuo Z., et al. (2020b). Copper induces hepatic inflammatory responses by activation of MAPKs and NF-κB signalling pathways in the mouse. Ecotoxicol. Environ. Saf. 201, 110806. doi:10.1016/j.ecoenv.2020.110806
Liu H., Guo H., Jian Z., Cui H., Fang J., Zuo Z., et al. (2020a). Copper induces oxidative stress and apoptosis in the mouse liver. Oxid. Med. Cell Longev. 2020, 1359164. doi:10.1155/2020/1359164
Liu J., Liu Y., Wang Y., Kang R., Tang D. (2022b). HMGB1 is a mediator of cuproptosis-related sterile inflammation. Front. Cell Dev. Biol. 10, 996307. doi:10.3389/fcell.2022.996307
Liu N., Chen M. (2024). Crosstalk between ferroptosis and cuproptosis: from mechanism to potential clinical application. Biomed. Pharmacother. 171, 116115. doi:10.1016/j.biopha.2023.116115
Liu Y., Zhu J., Xu L., Wang B., Lin W., Luo Y. (2022a). Copper regulation of immune response and potential implications for treating orthopedic disorders. Front. Mol. Biosci. 9, 1065265. doi:10.3389/fmolb.2022.1065265
Maestraggi Q., Lebas B., Clere-Jehl R., Ludes P.-O., Chamaraux-Tran T.-N., Schneider F., et al. (2017). Skeletal muscle and lymphocyte mitochondrial dysfunctions in septic shock trigger ICU-acquired weakness and sepsis-induced immunoparalysis. BioMed Res. Int. 2017, 7897325. doi:10.1155/2017/7897325
Mankowski R. T., Laitano O., Clanton T. L., Brakenridge S. C. (2021). Pathophysiology and treatment strategies of acute myopathy and muscle wasting after sepsis. J. Clin. Med. 10 (9), 1874. doi:10.3390/jcm10091874
Matés J. M., Campos-Sandoval J. A., de Los Santos-Jiménez J., Márquez J. (2020). Glutaminases regulate glutathione and oxidative stress in cancer. Archives Toxicol. 94 (8), 2603–2623. doi:10.1007/s00204-020-02838-8
Maung M. T., Carlson A., Olea Flores M., Elkhadragy L., Schachtschneider K. M., Navarro Tito N., et al. (2021). The molecular and cellular basis of copper dysregulation and its relationship with human pathologies. FASEB J. 35 (9), e21810. doi:10.1096/fj.202100273rr
McBride M. A., Owen A. M., Stothers C. L., Hernandez A., Luan L., Burelbach K. R., et al. (2020). The metabolic basis of immune dysfunction following sepsis and trauma. Front. Immunol. 11, 1043. doi:10.3389/fimmu.2020.01043
Merz T. M., Pereira A. J., Schürch R., Schefold J. C., Jakob S. M., Takala J., et al. (2017). Mitochondrial function of immune cells in septic shock: a prospective observational cohort study. PloS one 12 (6), e0178946. doi:10.1371/journal.pone.0178946
Mitobe Y., Morishita S., Ohashi K., Sakai S., Uchiyama M., Abeywickrama H., et al. (2019). Skeletal muscle index at intensive care unit admission is a predictor of intensive care unit-acquired weakness in patients with sepsis. J. Clin. Med. Res. 11 (12), 834–841. doi:10.14740/jocmr4027
Mitra S., Keswani T., Dey M., Bhattacharya S., Sarkar S., Goswami S., et al. (2012). Copper-induced immunotoxicity involves cell cycle arrest and cell death in the spleen and thymus. Toxicology 293 (1-3), 78–88. doi:10.1016/j.tox.2011.12.013
Mofarrahi M., Sigala I., Guo Y., Godin R., Davis E. C., Petrof B., et al. (2012). Autophagy and skeletal muscles in sepsis. PLoS One 7 (10), e47265. doi:10.1371/journal.pone.0047265
Nam E., Han J., Suh J. M., Yi Y., Lim M. H. (2018). Link of impaired metal ion homeostasis to mitochondrial dysfunction in neurons. Curr. Opin. Chem. Biol. 43, 8–14. doi:10.1016/j.cbpa.2017.09.009
Nedel W., Deutschendorf C., Portela L. V. C. (2023). Sepsis-induced mitochondrial dysfunction: a narrative review. World J. Crit. Care Med. 12 (3), 139–152. doi:10.5492/wjccm.v12.i3.139
Ng M. Y. W., Wai T., Simonsen A. (2021). Quality control of the mitochondrion. Dev. Cell 56 (7), 881–905. doi:10.1016/j.devcel.2021.02.009
Oliveira T. S., Santos A. T., Andrade C. B. V., Silva J. D., Blanco N., Rocha N. N., et al. (2021). Sepsis disrupts mitochondrial function and diaphragm morphology. Front. Physiol. 12, 704044. doi:10.3389/fphys.2021.704044
Park S., Jeon J. H., Min B. K., Ha C. M., Thoudam T., Park B. Y., et al. (2018). Role of the pyruvate dehydrogenase complex in metabolic remodeling: differential pyruvate dehydrogenase complex functions in metabolism. Diabetes Metab. J. 42 (4), 270–281. doi:10.4093/dmj.2018.0101
Pavlu-Pereira H., Silva M. J., Florindo C., Sequeira S., Ferreira A. C., Duarte S., et al. (2020). Pyruvate dehydrogenase complex deficiency: updating the clinical, metabolic and mutational landscapes in a cohort of Portuguese patients. Orphanet J. Rare Dis. 15 (1), 298. doi:10.1186/s13023-020-01586-3
Rafati Rahimzadeh M., Rafati Rahimzadeh M., Kazemi S., Moghadamnia A. A. (2024). Copper poisoning with emphasis on its clinical manifestations and treatment of intoxication. Adv. Public Health 2024 (1), 1–12. doi:10.1155/2024/6001014
Ruiz L. M., Jensen E. L., Rossel Y., Puas G. I., Gonzalez-Ibanez A. M., Bustos R. I., et al. (2016). Non-cytotoxic copper overload boosts mitochondrial energy metabolism to modulate cell proliferation and differentiation in the human erythroleukemic cell line K562. Mitochondrion 29, 18–30. doi:10.1016/j.mito.2016.04.005
Ruiz L. M., Libedinsky A., Elorza A. A. (2021). Role of copper on mitochondrial function and metabolism. Front. Mol. Biosci. 8, 711227. doi:10.3389/fmolb.2021.711227
Sakellariou G. K., Davis C. S., Shi Y., Ivannikov M. V., Zhang Y., Vasilaki A., et al. (2014). Neuron-specific expression of CuZnSOD prevents the loss of muscle mass and function that occurs in homozygous CuZnSOD-knockout mice. FASEB J. 28 (4), 1666–1681. doi:10.1096/fj.13-240390
Schefold J. C., Bierbrauer J., Weber-Carstens S. (2010). Intensive care unit—acquired weakness (ICUAW) and muscle wasting in critically ill patients with severe sepsis and septic shock. J. Cachexia, Sarcopenia Muscle 1 (2), 147–157. doi:10.1007/s13539-010-0010-6
Sheng Z., Yu Z., Wang M., Zhou R., Chen S., Yu X., et al. (2024). Targeting STAT6 to mitigate sepsis-induced muscle atrophy and weakness: modulation of mitochondrial dysfunction, ferroptosis, and CHI3L1-Mediated satellite cell loss. Biochem. Biophys. Rep. 37, 101608. doi:10.1016/j.bbrep.2023.101608
Shi M., Hu Z., Zhang X., You Q., Wang W., Yan R., et al. (2020). AMPK activation suppresses mTOR/S6K1 phosphorylation and induces leucine resistance in rats with sepsis. Cell Biol. Int. 44 (5), 1133–1141. doi:10.1002/cbin.11310
Shi Y., Ivannikov M. V., Walsh M. E., Liu Y., Zhang Y., Jaramillo C. A., et al. (2014). The lack of CuZnSOD leads to impaired neurotransmitter release, neuromuscular junction destabilization and reduced muscle strength in mice. PLoS One 9 (6), e100834. doi:10.1371/journal.pone.0100834
Singer M., Deutschman C. S., Seymour C. W., Shankar-Hari M., Annane D., Bauer M., et al. (2016). The third international consensus definitions for sepsis and septic shock (Sepsis-3). Jama 315 (8), 801–810. doi:10.1001/jama.2016.0287
Sjövall F., Morota S., Persson J., Hansson M. J., Elmér E. (2013). Patients with sepsis exhibit increased mitochondrial respiratory capacity in peripheral blood immune cells. Crit. Care 17, 1522. doi:10.1186/cc12831
Smith I. J., Alamdari N., O'Neal P., Gonnella P., Aversa Z., Hasselgren P. O. (2010). Sepsis increases the expression and activity of the transcription factor Forkhead Box O 1 (FOXO1) in skeletal muscle by a glucocorticoid-dependent mechanism. Int. J. Biochem. Cell Biol. 42 (5), 701–711. doi:10.1016/j.biocel.2010.01.006
Stacpoole P. W. (2017). Therapeutic targeting of the pyruvate dehydrogenase complex/pyruvate dehydrogenase kinase (PDC/PDK) Axis in cancer. J. Natl. Cancer Inst. 109 (11). doi:10.1093/jnci/djx071
Sun Z., Zhao Q., Zhang J., Hu Y., Qu J., Gao H., et al. (2024). Bioinformatics reveals diagnostic potential of cuproptosis-related genes in the pathogenesis of sepsis. Heliyon 10 (1), e22664. doi:10.1016/j.heliyon.2023.e22664
Tang D., Chen X., Kroemer G. (2022). Cuproptosis: a copper-triggered modality of mitochondrial cell death. Cell Res. 32 (5), 417–418. doi:10.1038/s41422-022-00653-7
Tarin M., Babaie M., Eshghi H., Matin M. M., Saljooghi A. S. (2023). Elesclomol, a copper-transporting therapeutic agent targeting mitochondria: from discovery to its novel applications. J. Transl. Med. 21 (1), 745. doi:10.1186/s12967-023-04533-5
Tsvetkov P., Coy S., Petrova B., Dreishpoon M., Verma A., Abdusamad M., et al. (2022). Copper induces cell death by targeting lipoylated TCA cycle proteins. Science 375 (6586), 1254–1261. doi:10.1126/science.abf0529
Ursini F., Maiorino M. (2020). Lipid peroxidation and ferroptosis: the role of GSH and GPx4. Free Radic. Biol. Med. 152, 175–185. doi:10.1016/j.freeradbiomed.2020.02.027
Vander Heiden M. G., Cantley L. C., Thompson C. B. (2009). Understanding the Warburg effect: the metabolic requirements of cell proliferation. Science 324 (5930), 1029–1033. doi:10.1126/science.1160809
van der Slikke E. C., Star B. S., van Meurs M., Henning R. H., Moser J., Bouma H. R. (2021). Sepsis is associated with mitochondrial DNA damage and a reduced mitochondrial mass in the kidney of patients with sepsis-AKI. Crit. Care 25, 36–13. doi:10.1186/s13054-020-03424-1
Vary T. C. (1996). Sepsis-induced alterations in pyruvate dehydrogenase complex activity in rat skeletal muscle: effects on plasma lactate. Shock 6 (2), 89–94. doi:10.1097/00024382-199608000-00002
Wai T., García-Prieto J., Baker M. J., Merkwirth C., Benit P., Rustin P., et al. (2015). Imbalanced OPA1 processing and mitochondrial fragmentation cause heart failure in mice. Science 350 (6265), aad0116. doi:10.1126/science.aad0116
Wang M., Feng J., Zhou D., Wang J. (2023a). Bacterial lipopolysaccharide-induced endothelial activation and dysfunction: a new predictive and therapeutic paradigm for sepsis. Eur. J. Med. Res. 28 (1), 339. doi:10.1186/s40001-023-01301-5
Wang W., Lu K., Jiang X., Wei Q., Zhu L., Wang X., et al. (2023b). Ferroptosis inducers enhanced cuproptosis induced by copper ionophores in primary liver cancer. J. Exp. Clin. Cancer Res. 42 (1), 142. doi:10.1186/s13046-023-02720-2
Wang Y., Zhao H., Shao Y., Liu J., Li J., Luo L., et al. (2018a). Copper (II) and/or arsenite-induced oxidative stress cascades apoptosis and autophagy in the skeletal muscles of chicken. Chemosphere 206, 597–605. doi:10.1016/j.chemosphere.2018.05.013
Wang Y., Zhao H., Shao Y., Liu J., Li J., Luo L., et al. (2018b). Copper or/and arsenic induces autophagy by oxidative stress-related PI3K/AKT/mTOR pathways and cascaded mitochondrial fission in chicken skeletal muscle. J. Inorg. Biochem. 188, 1–8. doi:10.1016/j.jinorgbio.2018.08.001
Weibull M. G. M., Simonsen S., Oksbjerg C. R., Tiwari M. K., Hemmingsen L. (2019). Effects of Cu(II) on the aggregation of amyloid-β. J. Biol. Inorg. Chem. 24 (8), 1197–1215. doi:10.1007/s00775-019-01727-5
Weiss S. L., Zhang D., Bush J., Graham K., Starr J., Murray J., et al. (2020). Mitochondrial dysfunction is associated with an immune paralysis phenotype in pediatric sepsis. Shock 54 (3), 285–293. doi:10.1097/SHK.0000000000001486
Wolfram T., Schwarz M., Reuß M., Lossow K., Ost M., Klaus S., et al. (2020). N-acetylcysteine as modulator of the essential trace elements copper and zinc. Antioxidants 9 (11), 1117. doi:10.3390/antiox9111117
Wu J., Liu H., Chu T., Jiang P., Li S. T. (2019). Neuregulin-1β attenuates sepsis-induced diaphragm atrophy by activating the PI3K/Akt signaling pathway. J. Muscle Res. Cell Motil. 40 (1), 43–51. doi:10.1007/s10974-019-09512-2
Xie J., Yang Y., Gao Y., He J. (2023). Cuproptosis: mechanisms and links with cancers. Mol. Cancer 22 (1), 46. doi:10.1186/s12943-023-01732-y
Xie X., Shu R., Yu C., Fu Z., Li Z. (2022). Mammalian AKT, the emerging roles on mitochondrial function in diseases. Aging Dis. 13 (1), 157–174. doi:10.14336/AD.2021.0729
Xue Q., Yan D., Chen X., Li X., Kang R., Klionsky D. J., et al. (2023). Copper-dependent autophagic degradation of GPX4 drives ferroptosis. Autophagy 19 (7), 1982–1996. doi:10.1080/15548627.2023.2165323
Yan J., Li Z., Li Y., Zhang Y. (2024). Sepsis induced cardiotoxicity by promoting cardiomyocyte cuproptosis. Biochem. Biophysical Res. Commun. 690, 149245. doi:10.1016/j.bbrc.2023.149245
Yang F., Pei R., Zhang Z., Liao J., Yu W., Qiao N., et al. (2019). Copper induces oxidative stress and apoptosis through mitochondria-mediated pathway in chicken hepatocytes. Toxicol Vitro 54, 310–316. doi:10.1016/j.tiv.2018.10.017
Yin D., Lin D., Xie Y., Gong A., Jiang P., Wu J. (2022). Neuregulin-1β alleviates sepsis-induced skeletal muscle atrophy by inhibiting autophagy via AKT/mTOR signaling pathway in rats. Shock 57 (3), 397–407. doi:10.1097/SHK.0000000000001860
Zanders L., Kny M., Hahn A., Schmidt S., Wundersitz S., Todiras M., et al. (2022). Sepsis induces interleukin 6, gp130/JAK2/STAT3, and muscle wasting. J. Cachexia Sarcopenia Muscle 13 (1), 713–727. doi:10.1002/jcsm.12867
Zeng L., Ai C. X., Zhang J. S., Li W. C. (2020). Pre-hypoxia exposure inhibited copper toxicity by improving energy metabolism, antioxidant defence and mitophagy in the liver of the large yellow croaker Larimichthys crocea. Sci. Total Environ. 708, 134961. doi:10.1016/j.scitotenv.2019.134961
Zeng R., Peng B., Peng E. (2022). Downregulated copper homeostasis-related gene FOXO1 as a novel indicator for the prognosis and immune response of breast cancer. J. Immunol. Res. 2022, 9140461. doi:10.1155/2022/9140461
Zhang B., Yang Q., Wang X., Jia B., Cheng L., Zeng H.-L. (2023). Association of whole blood metals/metalloids with severity in sepsis patients: a prospective, single-center, pilot study. J. Trace Elem. Med. Biol. 75, 127098. doi:10.1016/j.jtemb.2022.127098
Zhang M., Zhang X. (2019). The role of PI3K/AKT/FOXO signaling in psoriasis. Archives Dermatological Res. 311, 83–91. doi:10.1007/s00403-018-1879-8
Zhang Q., Raoof M., Chen Y., Sumi Y., Sursal T., Junger W., et al. (2010). Circulating mitochondrial DAMPs cause inflammatory responses to injury. Nature 464 (7285), 104–107. doi:10.1038/nature08780
Zhao D., Wu L., Fang X., Wang L., Liu Q., Jiang P., et al. (2024). Copper exposure induces inflammation and PANoptosis through the TLR4/NF-κB signaling pathway, leading to testicular damage and impaired spermatogenesis in Wilson disease. Chemico-Biological Interact. 396, 111060. doi:10.1016/j.cbi.2024.111060
Zhao H., Wang Y., Shao Y., Liu J., Wang S., Xing M. (2018). Oxidative stress-induced skeletal muscle injury involves in NF-κB/p53-activated immunosuppression and apoptosis response in copper (II) or/and arsenite-exposed chicken. Chemosphere 210, 76–84. doi:10.1016/j.chemosphere.2018.06.165
Zheng D., Liu J., Piao H., Zhu Z., Wei R., Liu K. (2022). ROS-triggered endothelial cell death mechanisms: focus on pyroptosis, parthanatos, and ferroptosis. Front. Immunol. 13, 1039241. doi:10.3389/fimmu.2022.1039241
Zheng M., Liu Y., Zhang G., Yang Z., Xu W., Chen Q. (2023). The applications and mechanisms of superoxide dismutase in medicine, food, and cosmetics. Antioxidants (Basel) 12 (9), 1675. doi:10.3390/antiox12091675
Zhou Q., Zhang Y., Lu L., Zhang H., Zhao C., Pu Y., et al. (2022). Copper induces microglia-mediated neuroinflammation through ROS/NF-κB pathway and mitophagy disorder. Food Chem. Toxicol. 168, 113369. doi:10.1016/j.fct.2022.113369
Zorowitz R. D. (2016). ICU-acquired weakness: a rehabilitation perspective of diagnosis, treatment, and functional management. Chest 150 (4), 966–971. doi:10.1016/j.chest.2016.06.006
ICU Intensive care unit
ICU-AW Intensive care unit-acquired weakness
SAW Sepsis-acquired weakness
ATP Adenosine triphosphate
ROS Reactive oxygen species
SOD1 Superoxide dismutase 1
TCA Tricarboxylic acid
RCD Regulated cell death
APC Activated protein C
ECs Endothelial cells
NF-κB Nuclear Factor kappa B
MAPK Mitogen-activated protein kinase
TLR Toll-like receptors
AMPK 5' adenosine monophosphate-activated protein kinase
mTOR mammalian Target of Rapamycin
IL-1β Interleukin-1β
IL-18 Interleukin-18
GSDMD Gasdermin D
NLRP3 NOD-like receptor family pyrin domain-containing 3
AKT Serine/threonine kinase
Nrf2 Nuclear factor erythroid 2-related factor 2
Keap1 Kelch-like epichlorohydrin-associated protein 1
HSP70 Heat shock protein-70
MT Metallothionein
COX17 Cytochrome c oxidase copper chaperone
CCS Copper chaperone for SOD1
GSH Glutathione
COX Cytochrome c oxidase
Bcl-2 B-cell lymphoma-2
NAC N-acetylcysteine
Fe-S Iron-sulfur
PI3K Phosphatidylinositol-3-kinase
FOXO Forkhead box O
JAK Janus kinase
STAT Signal Transducer and Activator of Transcription
SOCS3 Suppressor of Cytokine Signaling 3
SPSB1 SPRY domain-containing SOCS box protein 1
GPX 4 Glutathione peroxidase 4
FDX1 Ferredoxin 1
CRISPR Clustered Regularly Interspaced Palindromic Repeats
PDC Pyruvate dehydrogenase complex
LA α-lipoic acid
PDHB Pyruvate dehydrogenase E1 subunit β
HMGB1 High mobility group protein B1
Gln Glutamine
ATP7A Copper ion-transporting ATPase alpha polypeptide
Keywords: sepsis-acquired weakness, cuproptosis, mitochondria, copper, cell death
Citation: Yang L, Xie L, Li M, Miao Y, Yang J, Chen S, Ma X and Xie P (2025) Potential relationship between cuproptosis and sepsis-acquired weakness: an intermediate role for mitochondria. Front. Physiol. 16:1520669. doi: 10.3389/fphys.2025.1520669
Received: 31 October 2024; Accepted: 05 March 2025;
Published: 20 March 2025.
Edited by:
Elizabeth S. Fernandes, Aarhus University, DenmarkReviewed by:
Arun Kumar Yadawa, Texas A and M University, United StatesCopyright © 2025 Yang, Xie, Li, Miao, Yang, Chen, Ma and Xie. This is an open-access article distributed under the terms of the Creative Commons Attribution License (CC BY). The use, distribution or reproduction in other forums is permitted, provided the original author(s) and the copyright owner(s) are credited and that the original publication in this journal is cited, in accordance with accepted academic practice. No use, distribution or reproduction is permitted which does not comply with these terms.
*Correspondence: Peng Xie, MjAyMzAyMDIyMUB1c2MuZWR1LmNu
†ORCID: Peng Xie, orcid.org/0009-0005-2438-3362
‡These authors have contributed equally to this work and share first authorship
Disclaimer: All claims expressed in this article are solely those of the authors and do not necessarily represent those of their affiliated organizations, or those of the publisher, the editors and the reviewers. Any product that may be evaluated in this article or claim that may be made by its manufacturer is not guaranteed or endorsed by the publisher.
Research integrity at Frontiers
Learn more about the work of our research integrity team to safeguard the quality of each article we publish.