- 1Department of Kinesiology, The University of Alabama, Tuscaloosa, AL, United States
- 2Department of Kinesiology, New Mexico State University, Las Cruces, NM, United States
- 3Department of Exercise Science, Mercer University, Macon, GA, United States
Introduction: Higher work rates may be sustainable when maintaining target rating of perceived exertion (RPE) compared to maintaining target heart rate (THR) during high-intensity interval training (HIIT) exercise in hot conditions, but may also result in greater thermal strain and cardiovascular drift, as well as greater decrements in maximal oxygen uptake (
Purpose: To test the hypotheses that maintaining target RPE compared to THR during HIIT in the heat results in 1) smaller work rate adjustments, 2) greater thermal and cardiovascular strain, and 3) larger decreases in
Methods: Eight adults (4 women) completed a graded exercise test on a cycle ergometer in 22°C and then 4 cycling trials in 35°C, consisting of an 8-min warm-up at 70% maximal heart rate (HRmax) or 12 RPE followed by 1 (15HR and 15RPE) or 5 (43HR and 43RPE) rounds of HIIT (1 round = 4 min work at 90% HRmax or 17 RPE and 3 min recovery at 70% HRmax or 12 RPE) totaling 15 min or 43 min of exercise, respectively. Each trial ended with a GXT to measure
Results: In the 43-min trials work rate decreased from the first to the fifth work interval in both conditions, but by a non-significant, yet moderately larger (ES = 0.53) amount during 43HR (46 ± 29 W) compared to 43RPE (30 ± 28 W). From the first to fifth work interval HR increased over time by 12 b⋅min–1 in 43RPE (p < 0.001), but did not increase during 43HR (p = 0.36). Rectal temperature increases were not different between conditions (43HR = 0.7°C, p < 0.001; 43RPE = 0.8°C, p < 0.001).
Conclusion: Maintaining target RPE and THR require considerable declines in work rate during HIIT in the heat, with ∼53% larger declines needed to maintain THR. The mitigation of cardiovascular drift in the THR trial may have contributed to the preservation of
1 Introduction
The intensity of work and rest intervals during high-intensity interval training (HIIT) can be prescribed using work rate (speed or power output), oxygen uptake (
Because of these limitations, and as a result of its ease of use and linear relationship with
A simple alternative to using THR when prescribing intensity of a HIIT session is to use rating of perceived exertion (RPE), a subjective measure of intensity (Borg and Noble, 1974). RPE is an appealing method of prescribing exercise intensity because it requires no equipment, allows the individual to adjust the intensity based on how the intensity of exercise is perceived, and in young healthy individuals, is directly related to HR (Borg, 1982). RPE has been repeatedly shown to be a valid method to gauge exercise intensity in temperate conditions (Eston and Williams, 1988; Edwards et al., 1972; Dunbar et al., 1992). During constant-intensity exercise in the heat, however, RPE is elevated compared to cooler environments (Maw et al., 1993), and, like HR, it may progressively increase over time despite no change in work rate (Pandolf, 1998; Wingo and Cureton, 2006a; Wingo et al., 2005; Wingo et al., 2020). Therefore, like THR, to maintain target RPE in hot environments, work rate must be lowered to a larger extent compared to that in cooler environments (Tucker et al., 2006; Roussey et al., 2018). Even so, the magnitude by which work rate needs to be lowered to maintain target RPE in the heat appears to be less than that to maintain THR (Tucker et al., 2006). Consequently, using target RPE to gauge exercise intensity during HIIT in the heat may be advantageous compared to using THR because a higher work rate can be maintained and thereby a greater training stimulus, but this has not been evaluated. An unintended, but important consequence of this, will likely be higher core body temperature and amplified cardiovascular strain (indexed as cardiovascular drift), but no study has addressed the extent to which this may occur.
In addition to the aforementioned considerations regarding cardiovascular drift, a consequence of cardiovascular drift is that it corresponds to reduced maximal oxygen uptake (
Given the preceding notions, the purposes of this study were to test the hypotheses that 1) work rate would be lowered to a greater extent to maintain THR than to maintain target RPE during HIIT in a hot environment, 2) greater thermal and cardiovascular strain would result from maintaining target RPE compared to THR during a HIIT workout in the heat, and 3)
2 Materials and methods
2.1 Experimental design
Participants visited the laboratory on 5 separate days (1 control trial, two 15-min experimental trials, and two 43-min experimental trials). At each visit, they completed an exercise bout on a cycle ergometer (LC6 Novo, Monark Exercise, Vansbro, Sweden).
The first visit was a control trial; participants completed a graded exercise test (GXT) to measure maximal HR (HRmax) and
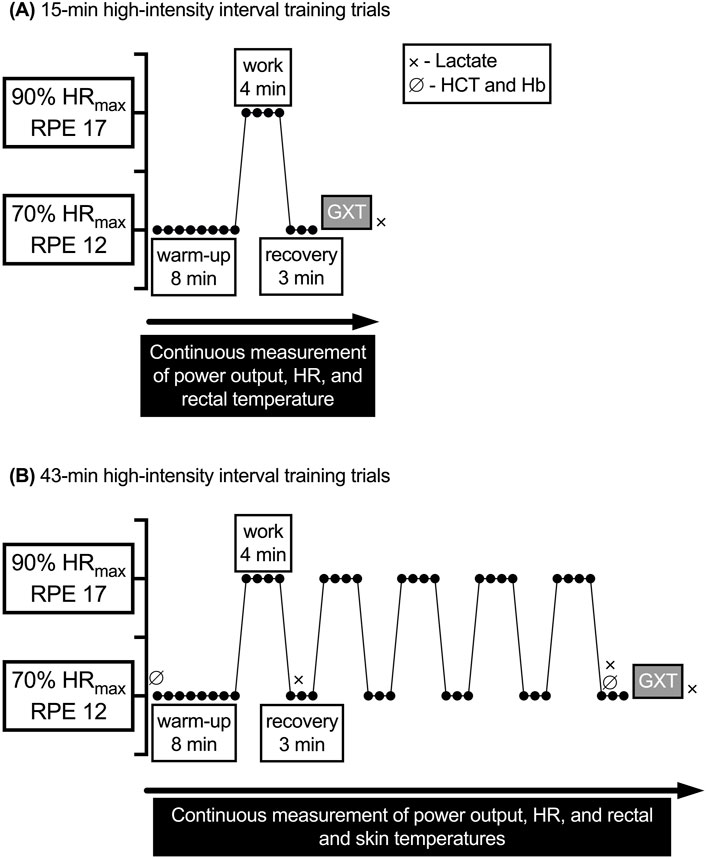
Figure 1. General exercise protocols for the 15- and 43-min experimental trials. GXT, graded exercise test; Hb, hemoglobin: HCT, hematocrit; HR, heart rate; HRmax, maximal heart rate; RPE, rating of perceived exertion;
An a priori power analysis (G*power 3.1.9.6) revealed a sample size of 7 would be sufficient to detect a 25-W difference between the change score in power output from the first to the final work interval in 43HR versus 43RPE, assuming α = 0.05 and power ≈0.80 (Faul et al., 2009; Faul et al., 2007). Eight healthy adults (4 men and 4 women; 18–38 y) free of disease participated. Seven were recreationally active as defined by the American College of Sports Medicine (2022) (i.e., exercising at a moderate intensity aerobically ≥30 min per day, ≥3 times per week, for the past ≥3 months) and 1 male was a competitive endurance athlete. Physical characteristics of participants were age (mean ± SD) = 25 ± 7 y, body mass = 74.1 ± 8.3 kg, height = 181 ± 10 cm, percent body fat = 21.4% ± 8.4%,
Women with a regular menstrual cycle lasting 21–35 days were included (Elliott-Sale et al., 2021). They were asked to self-report the first and last day of previous menses and contraceptive use for data analysis and scheduling. All experimental trials were scheduled during the same phase of their menstrual cycle (luteal phase or follicular phase), although the specific phase was not expected to affect study outcomes (Stone et al., 2021). Two of the 4 women completed the experimental trials in the luteal phase of their menstrual cycle. Although phase of menstrual cycle was not confirmed via hormonal assay, based on cycle reporting and rectal temperature (Tre) it is likely 1 woman completed the 43-min trials in the follicular phase and the remaining trials in the luteal phase. One woman who was using an oral contraceptive [norgestimate (0.25 mg) and ethinyl estradiol (0.035 mg)] was tested in her follicular phase.
For each of the 5 trials, participants were instructed to abstain from consuming alcohol or participating in strenuous exercise during the 24 h before testing. Additionally, participants were asked to report to the laboratory well rested, euhydrated, and having refrained from ingesting non-prescription drugs and caffeine on the day of testing. Pre-testing instructions have been successfully used by our lab previously and adherence was confirmed using a 24-h history questionnaire (Yoder et al., 2023; Mulholland et al., 2023).
Upon arrival, participants provided a urine sample that was analyzed for urine specific gravity (USG) using a digital refractometer (ATAGO PAL-10S digital refractometer, Tokyo, Japan). USG had to be ≤1.020 for a participant to be considered adequately hydrated (Sawka et al., 2007). Participants whose USG values were >1.020 were given water to ingest for 20–30 min and then reevaluated. Then participants dressed in padded cycling shorts and a mesh tank top and were equipped with chest strap HR monitor (H10, Polar Electro, Kempele, Finland) that paired with a smartphone application (Polar Beat, version 3.5.0, Polar Electro, Kempele, Finland). Prior to beginning exercise, the Borg 6–20 RPE scale was explained using standardized instructions (Borg and Noble, 1974).
A minimum of 24 h separated control trials from subsequent experimental trials and at least 48 h separated experimental trials from one another. All trials for a given participant took place over ≤8 weeks and each was completed at a similar time of day to control for fluctuations in core body temperature associated with circadian rhythm (Moore-Ede et al., 1983).
2.2 Control O2max trial
At the first visit, participants completed a questionnaire about their readiness to participate in exercise and a general health history form. Next, height was measured using a stadiometer (SECA 213, Seca Ltd., Hamburg, Germany) and body mass was measured with a digital scale (Tanita WB-800S, Tanita Corp., Tokyo Japan). Body fat percentage was calculated from the sum of 3 skinfolds (Jackson and Pollock, 1985).
Participants then began a self-selected warm-up for 5–10 min on the cycle ergometer. Next, the GXT started and every 2 min the power output on the cycle ergometer was increased by 25 W until volitional exhaustion was reached or pedal cadence fell below 30 rev⋅min–1.
Twenty min following the GXT, participants completed a
2.3 Experimental trials
At least 24 h following the control trial, participants returned to the laboratory for the first experimental trial. In addition to the procedures outlined under “all trials,” for the experimental trials, participants measured nude body mass and inserted a flexible rectal thermistor 10 cm beyond the anal sphincter for measurement of Tre. The thermistor was integrated with wireless amplifiers (BioNomadix Wireless SKT Transmitter, Biopac Systems, Inc., Goleta, CA, United States) set to a sampling frequency of 1,000 Hz. Tre and ambient temperature were recorded continuously using a data acquisition system (MP150, Biopac Systems, Inc., Goleta, CA, United States) powered by data analysis software (AcqKnowledge 4.2, Biopac Systems, Inc., Goleta, CA, United States). During the trials based on HR, a member of the research team monitored HR and adjusted the workload to maintain HR within 5 b⋅min–1 of THR during the entire workout or at 30 W during the recovery intervals if THR was not achievable. During trials based on RPE, the participant adjusted the power output (with the power output concealed) to match the target RPE. The RPE scale was continuously visible to participants throughout the exercise sessions and participants were frequently reminded to adjust resistance to remain at the prescribed intensity. During the HR-based trials, participants were instructed to point to a value on the chart that matched their RPE at the end of the first and fifth work and recovery intervals; the value was verbally confirmed by a member of the research team.
All blood samples taken before, during, and after the experimental trials were drawn from a superficial forearm vein into a Vacutainer containing EDTA for measurement of either lactate concentration, hematocrit (HCT) and hemoglobin (Hb) concentration, or both, as specified in Figure 1. HCT was assessed in triplicate using a microcapillary reader (Model 3201, International Equipment Co., Boston, MA, United States); Hb concentration was assessed in duplicate using a Hb analyzer (HemoPoint H2, EKF Diagnostics, Inc., Boerne, TX, United States). HCT and Hb were then used to calculate plasma volume change (Dill and Costill, 1974). After the last round of HIIT recovery, participants immediately began a GXT in the same manner as during the control trial with no cessation of exercise to determine
Even though the plateau verification procedure for
2.3.1 15-min trials
Participants entered the environmental chamber and mounted the cycle ergometer. Next, instrumentation was connected and baseline measurements were taken (∼15 min). After baseline measurements, participants completed 1 of the 15-min trials, which included a warm-up and 1 round of HIIT followed by a GXT to determine
2.3.2 43-min trials
For the 43HR and 43RPE trials, skin temperature was measured using 4 iButtons (model no. DS1921H, Embedded Data Systems, KY, United States) taped to each participant’s right upper chest, lateral deltoid, anterior thigh, and lateral calf with elastic therapeutic tape. Skin temperatures from these sites were then used to calculate mean skin temperature (
where Tchest, Tdelt, Tthigh, and Tcalf are the skin temperatures at the chest, deltoid, thigh, and calf, respectively. Mean body temperature (
The core-to-skin thermal gradient was calculated as the difference between Tre and
where
Next, a flexible catheter was placed into a forearm vein for 2-mL blood sample collection before, during the trial at time points corresponding to the end of the high-intensity bouts (min 12 and 40), and after exercise. Blood lactate concentration was measured at the end of the first and fifth work intervals and upon completion of the GXT. HCT and Hb were measured at baseline and the end of the fifth work interval.
Following the placement of the catheter, participants entered the environmental chamber and mounted the cycle ergometer. The remaining instrumentation was then connected, a 2-mL blood sample was drawn, and other baseline measurements were taken (∼15 min). Participants then began one of the 43-min trials (warm-up, 5 rounds of HIIT, and GXT). At min 12 and 40 participants were asked to report their thermal sensation on a numerical scale (Young et al., 1987). Approximately 20 min after the exercise session, participants were asked to rate the session RPE (Foster et al., 2001).
2.4 Data analysis
Mean data were generated on the indicated outcome measures. To test the significance of mean differences in power output, a 2-way [condition × time (work intervals 1 and 5)] repeated measures analysis of variance (ANOVA) was used. Power output was also assessed by comparing the change in power output from the first work interval (min 9–11) to the fifth work interval (min 37–40) between the 43HR and 43RPE trials using a paired samples t-test. Paired samples t-tests were also used to evaluate the difference in Tre,
Baseline data for control and experimental trials were analyzed using a 1-way repeated measures ANOVA. Planned contrasts were performed to compare
For hematological variables, 2-way repeated measures ANOVAs [condition × time (work intervals 1 and 5)] were conducted. For other variables, such as HR, Tre,
where, SD1 and SD2 are the standard deviations of the respective time points or conditions and n is the number of pairs. ES were interpreted as: 0.20 = small, 0.50 = medium, and 0.80 = large, respectively (Caldwell and Cheuvront, 2019; Fritz et al., 2012).
For select variables, the 95% confidence interval (CI) was calculated for the mean difference between conditions (for pairwise comparisons of interest) using a critical t (adjusted, if applicable, to keep the family-wise error rate α at 0.05) in the following formula (Weir and Vincent, 2020):
where tcv is the critical t value (adjusted for multiple comparisons, if applicable) and SEd is the standard error of the differences.
For power output,
3 Results
3.1 Hydration
Participants were adequately hydrated prior to all trials (mean ± SD, USG control = 1.005 ± 0.002, 15HR = 1.007 ± 0.005, 15RPE = 1.005 ± 0.004, 43HR = 1.006 ± 0.006, 43RPE = 1.006 ± 0.003; p = 0.82). Additionally, pre-exercise body mass was comparable among trials (control = 73.9 ± 7.9 kg, 15HR = 73.9 ± 7.6 kg, 15RPE = 74.4 ± 8.8 kg, 43HR = 74.3 ± 8.9 kg, 43RPE = 74.0 ± 8.0 kg; p = 0.68). Percent change in body mass from before to after exercise for each experimental trial was greater in the 45-min vs. 15-min trials (15HR = −0.7% ± 0.4%, 15RPE = −0.6% ± 0.4%, 43HR = −1.3% ± 0.8%, and 43RPE = −1.3% ± 0.8%; p = 0.004 for main effect of time). Additionally, percent change in plasma volume pre-to post-HIIT exercise was not different between 43-min trials (43HR = −9.0% ± 3.3%, 43RPE = −9.5% ± 3.9%, p = 0.67).
3.2 Cardiovascular, work rate, metabolic, and perceptual responses during HIIT exercise
3.2.1 Cardiovascular
As designed, during the work intervals of the HR-based trial, HR during the final min did not increase from the first to fifth interval and THR was achieved (Table 1; Figure 2). In contrast, during 43RPE, HR increased by 12 b⋅min–1 from the first to fifth work interval. During the final min of recovery intervals across both 43-min trials, HR increased by 11 b⋅min–1 from the first to fifth recovery interval and HR was 13 b⋅min–1 higher during the RPE-based trial. HR during the final min of the first and fifth recovery intervals increased from 72% to 78% HRmax in the HR trial and from 79% to 85% HRmax in the RPE trial (p < 0.001 for main effect of time; p = 0.006 for main effect of condition). Similar patterns were observed for %HRmax averaged over the entire work and recovery intervals and are shown in Figure 2A.
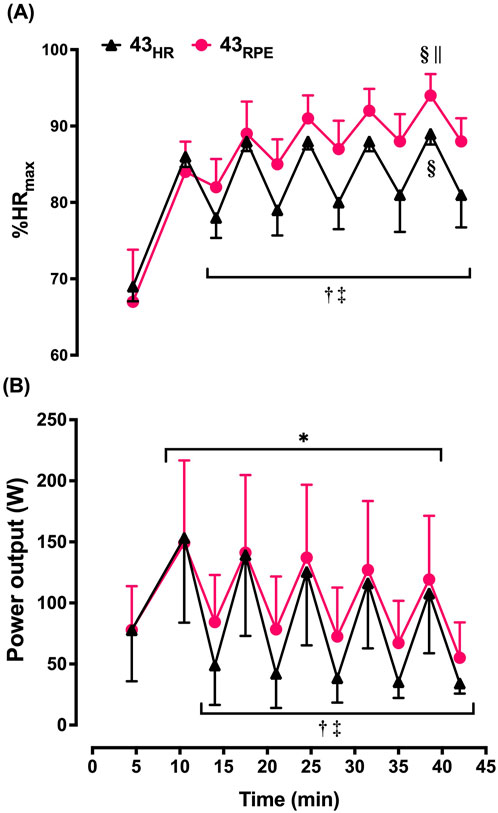
Figure 2. Mean ± SD heart rate (expressed as percentage of maximum [%HRmax]; (A) and power output (B) averaged over each interval during the 43-min trials. 43HR = 43-min trial based on target heart rate; 43RPE = 43-min trial based on target rating of perceived exertion. *p < 0.05 main effect of time during work intervals; †p < 0.05 main effect of time during recovery intervals; ‡p < 0.05 main effect of condition during recovery intervals; §p < 0.05 compared with work interval 1 of the given condition; ||p < 0.05 compared with heart rate-based trial during the same work interval.
During 43HR, 4 participants were able to reach THR during the first recovery interval, 3 in the second, and the same 2 participants for the final 3 recovery intervals. During the 43RPE trial, 2 participants cycled at the minimum 30 W for the final 2 intervals. Two participants reached or surpassed the HRmax observed in the control trial during the HIIT portion of the 43RPE trial.
3.2.2 Power output
Across both 43-min trials, power output had to be lowered by 38 W (ES = 0.59) between the first and fifth work intervals to maintain the target intensity, but conditions were not statistically different (Table 1; Figure 2B). Likewise, the t-test comparing the change score between the first and fifth work interval for 43HR (−46 ± 29 W) and the change score between the first and fifth work interval for 43RPE (−30 ± 28 W) was not statistically significant [mean difference (MD) = 16 ± 36 W; 95% CI for MD = −45, 15; ES = 0.53 ], but the magnitude of difference between these change scores was moderate. During the recovery intervals power output was 22 W lower during the fifth interval across both conditions (ES = 0.79) and 28 W (ES = 1.00) lower in the HR-based trial across both time points.
3.2.3 Metabolic and perceptual responses
In the work intervals, absolute
3.3 Thermoregulatory responses to HIIT exercise
Baseline Tre was not different among the 4 experimental trials (15HR = 37.3°C ± 0.3°C, 15RPE = 37.2°C ± 0.3°C, 43HR = 37.1°C ± 0.3°C, 43RPE = 37.1°C ± 0.3°C, p = 0.19). M–W increased over time in 43RPE and decreased over time in 43HR. Nonetheless, Tre increased by a comparable amount between experimental conditions so that Tre at the end of the final recovery interval was not different between conditions (MD = 0.2 ± 0.2; 95% CI for MD = −0.05, 0.46; ES = 0.36; Figure 3). Tre was also similar between conditions at the end of the first recovery interval. By the end of the GXT in the 43-min trials, Tre was higher following the RPE trial compared to the HR trial (p = 0.03). Across both experimental conditions,
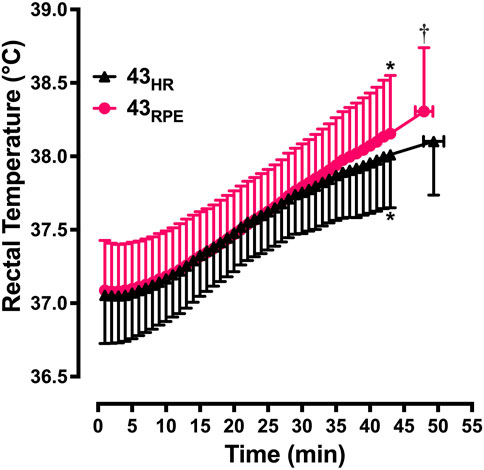
Figure 3. Mean ± SD rectal temperature from the start of exercise to the end of the graded exercise test. 43HR = 43-min trial based on target heart rate; 43RPE = 43-min trial based on target rating of perceived exertion. *p < 0.05 compared to min 15 of the same condition; †p < 0.05 compared to 43HR at maximum effort.
3.4 Maximal responses
Maximal responses are shown in Table 2 and Figure 4. Planned comparisons between control
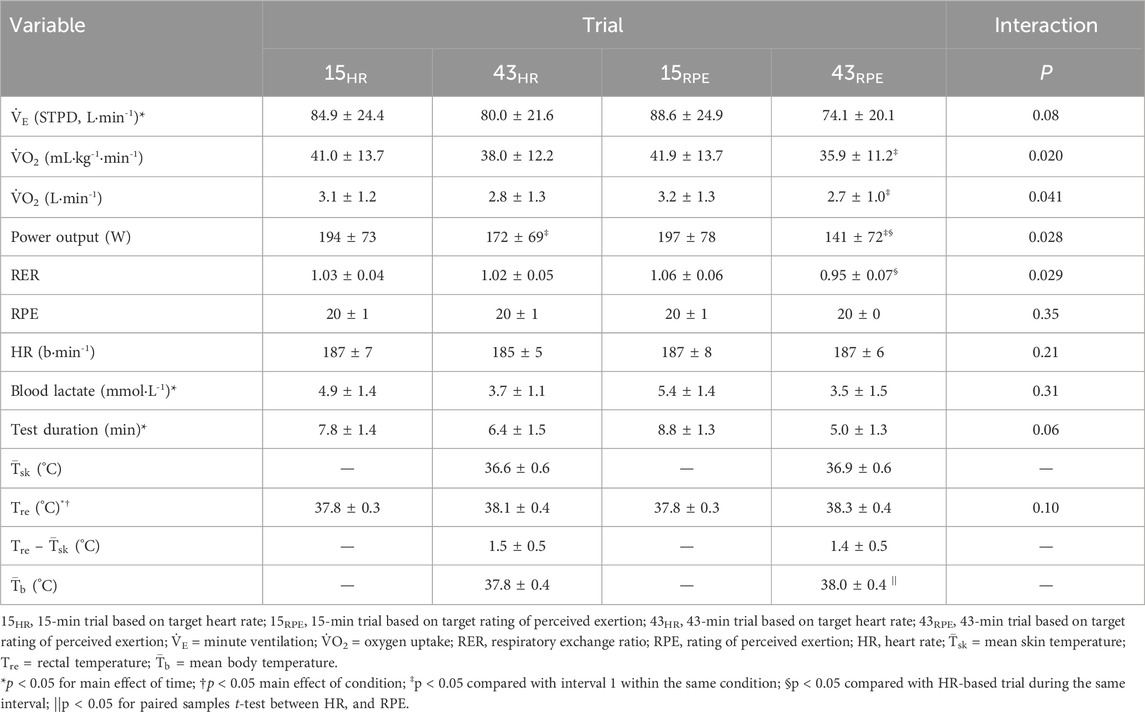
Table 2. Maximal responses during a graded exercise test following 15 min (after 1 work and recovery interval) and 43 min (after 5 work and recovery intervals) of high-intensity interval training exercise in a hot environment using heart rate or rating of perceived exertion to prescribe exercise intensity.
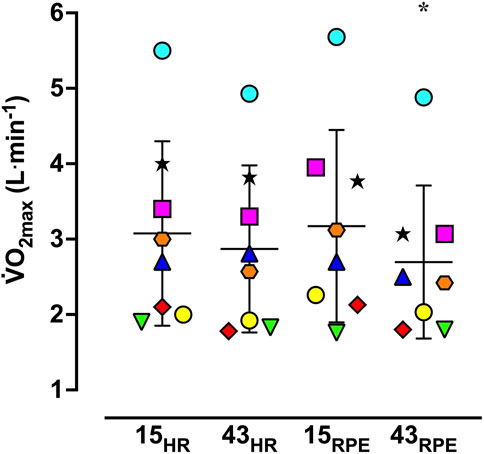
Figure 4. Vertical scattergram of maximal oxygen uptake (
For the 43-min trials, the maximal power output achieved during the GXT was lower compared to the respective 15-min trial. Additionally, the maximal power output during the GXT was 31 W lower in the 43RPE trial versus the 43HR trial. Tre and
4 Discussion
The purpose of this study was to evaluate work rate adjustments and thermal and cardiovascular strain using two simple methods of exercise prescription, THR and target RPE, to prescribe HIIT in the heat. A secondary purpose was to evaluate changes in aerobic capacity (
The range of decreases in work rate from the first to final work interval (43RPE = 18% and 43HR = 30%) were comparable to what others have observed during HIIT in a temperate environment using THR (21%) (Morales-Palomo et al., 2017), during 45 min of continuous exercise in the heat using THR (37%) (Wingo and Cureton, 2006b), and during 30 min of continuous exercise in the heat using target RPE (≈27%) (Tucker et al., 2006). While work rate was not statistically different during the work intervals, the 16 W (ES = 0.53) greater reduction in work rate during 43HR may be practically meaningful. However, unlike our results where work rate was lower during recovery intervals of 43HR, no differences in running speed were observed during work or recovery intervals when using RPE compared to THR to prescribe exercise intensity during a 20-min treadmill walking/running HIIT session in a temperate environment (Ciolac et al., 2015). Still others have found lower intensities using RPE compared to THR during interval training (Aamot et al., 2014) and during continuous exercise (Shea et al., 2022) in cardiac rehabilitation patients in temperate environments. Taken together, it appears that findings related to work rate adjustments during HIIT exercise based on THR and target RPE are equivocal. It is likely the heat stress in the current study contributed to the variability of findings in the literature. Differences could also be attributed to variations in exercise protocol, mode and duration, or participant characteristics.
We predicted work rate and thermal strain would be greater during 43RPE versus 43HR, but statistically higher work rates were only observed during the recovery intervals of 43RPE, and did not result in increased thermal strain during the HIIT protocol. During the HIIT sessions, Tre,
Although the elevated work rate during the recovery intervals of the RPE-based trial did not result in increased thermal strain, cardiovascular strain was greater during 43RPE as indicated by an ≈ 5% higher HR averaged over the fifth work interval and in the final min of the fifth work interval. An increase in HR over time during interval training was observed in temperate environments when work rate was held constant (Thomas et al., 2020), when work rate was self-selected during intervals of 4 or 8 min (Fennell and Hopker, 2021), and in temperate and hot environments when maximal sprint intervals were performed (Maxwell et al., 2008). Fennell and Hopker (2021) manipulated recovery intensity during a similar HIIT protocol (6 × 4 min with 2 min recovery in a temperate environment) where participants recovered at 80% or 110% of the power output corresponding to their lactate threshold. Unlike our results, the different recovery intensities did not affect HR during the work intervals, but during the recovery intervals HR was 7 b⋅min–1 higher during the 110% compared to 80% power output of their lactate threshold, respectively. Similarly, the difference in HR between conditions was unlike the findings of Ciolac et al. (2015) and Johnson et al. (2017) who observed similar HR when using THR and RPE to prescribe running intensity in temperate indoor and outdoor environments at varying exercise intensities. It appears that heat stress may alter the relationship between HR and RPE that is observed in temperate environments.
The progressive increase in HR (and accompanying decrease in stroke volume) during continuous exercise in the heat has been shown to be associated with decreased maximal aerobic capacity (Wingo and Cureton, 2006b; Lafrenz et al., 2008). As such, the ∼2.5 times greater decrease in
Although work rate and
As mentioned, aerobic capacity decreased over twice as much following the RPE trial. We speculate the greater M–W in this trial resulted in a larger peripheral displacement of blood volume to the skin for heat dissipation. This peripheral displacement of blood volume, combined with the higher HR at the end of the final round of HIIT, could have corresponded to a lower stroke volume (Coyle and Gonzalez-Alonso, 2001; Turkevich et al., 1988; Rowell et al., 1966; Rowell et al., 1969; Wingo et al., 2012). If this lower stroke volume persisted during maximal exercise, it could explain the decrease in
Although both conditions resulted in large declines in work rate, participants were able to complete the entire HIIT protocol followed by a GXT in the heat. Exploring other methods or strategies for intensity prescription of HIIT in the heat may be beneficial for maintaining work interval intensity. Manipulating recovery intensity (such as using passive recovery instead of active recovery) may be one way to preserve work rate during the work intervals based on RPE.
4.1 Limitations
A limitation of using RPE to prescribe exercise intensity is the different interpretations of the scale. The RPE scale was explained to participants using standardized instructions and they were instructed to complete the work intervals at an RPE of 17 which meant adjusting the resistance up or down to elicit the prescribed RPE. Nonetheless, it appears 1 participant paced themselves and increased power output from the first to final work interval in the 43RPE trial. This participant preserved their
Another challenge with using RPE to prescribe intensity during exercise in the heat is the disassociation between the prescribed intensity and the HR response observed during recovery intervals. For instance, participants were instructed to cycle at an RPE of 12 during recovery, which is considered a moderate intensity corresponding to 64%–74% HRmax (Garber et al., 2011). However, based on %HRmax, participants exercised at a vigorous intensity (85% HRmax) during the final recovery interval (Garber et al., 2011). Even during the first interval of recovery, based on HR, participants were at a vigorous intensity (79% HRmax). HR was elevated to such an extent that the %HRmax during the last 3 recovery intervals of 43RPE were about the same as the work intervals of the 43HR trial (Figure 2A). Furthermore, 2 participants achieved or surpassed the HRmax observed during the control trial during the submaximal HIIT portion of 43RPE. Using THR in the heat also proved problematic for prescribing intensity during recovery intervals because most participants were unable to achieve the THR and instead cycled at 30 W.
Despite the limitations of using RPE and THR to prescribe intensity in the present study, both resulted in the exercise intensity being attainable across the varying fitness levels of the participants.
4.2 Conclusion
Using target RPE and THR to prescribe HIIT exercise in the heat resulted in considerable declines in work rate during the work intervals, and lower work rates were needed to maintain THR compared to target RPE during the recovery intervals. The higher power output sustained in 43RPE recovery intervals corresponded to elevated cardiovascular strain during both work and recovery intervals, as well as a greater decline in
Data availability statement
The raw data supporting the conclusions of this article will be made available by the authors, without undue reservation.
Ethics statement
The studies involving humans were approved by University of Alabama Institutional Review Board. The studies were conducted in accordance with the local legislation and institutional requirements. The participants provided their written informed consent to participate in this study.
Author contributions
HY: Conceptualization, Data curation, Formal Analysis, Investigation, Methodology, Project administration, Software, Validation, Visualization, Writing–original draft, Writing–review and editing. AM: Investigation, Writing–review and editing. HM: Conceptualization, Project administration, Supervision, Writing–review and editing. JW: Conceptualization, Formal Analysis, Methodology, Resources, Supervision, Writing–original draft, Writing–review and editing.
Funding
The author(s) declare that no financial support was received for the research, authorship, and/or publication of this article.
Acknowledgments
A preprint version of this study was posted online as part of a dissertation (Yoder et al., 2022).
Conflict of interest
The authors declare that the research was conducted in the absence of any commercial or financial relationships that could be construed as a potential conflict of interest.
Generative AI statement
The author(s) declare that no Generative AI was used in the creation of this manuscript.
Publisher’s note
All claims expressed in this article are solely those of the authors and do not necessarily represent those of their affiliated organizations, or those of the publisher, the editors and the reviewers. Any product that may be evaluated in this article, or claim that may be made by its manufacturer, is not guaranteed or endorsed by the publisher.
References
Aamot I. L., Forbord S. H., Karlsen T., Støylen A. (2014). Does rating of perceived exertion result in target exercise intensity during interval training in cardiac rehabilitation? A study of the Borg scale versus a heart rate monitor. J. Sci. Med. Sport 17 (5), 541–545. doi:10.1016/j.jsams.2013.07.019
American College of Sports Medicine (2022). ACSM's guidelines for exercise testing and prescription. 11th edn. Philadelphia, PA: Wolters Kluwer Health.
Arazi H., Keihaniyan A., EatemadyBoroujeni A., Oftade A., Takhsha S., Asadi A., et al. (2017). Effects of heart rate vs. Speed-based high intensity interval training on aerobic and anaerobic capacity of female soccer players. Sports 5 (3), 57. doi:10.3390/sports5030057
Bolter C. P., Atkinson K. J. (1988). Influence of temperature and adrenergic stimulation on rat sinoatrial frequency. Am. J. Physiol. 254 (5), R840–R844. doi:10.1152/ajpregu.1988.254.5.R840
Borg G. A. (1982). Psychophysical bases of perceived exertion. Med. Sci. Sports Exerc 14 (5), 377–381. doi:10.1249/00005768-198205000-00012
Borg G. A., Noble B. J. (1974). Perceived exertion. Exerc Sport Sci. Rev. 2, 131–154. doi:10.1249/00003677-197400020-00006
Buchheit M., Laursen P. B. (2013). High-intensity interval training, solutions to the programming puzzle: Part I: cardiopulmonary emphasis. Sports Med. 43 (5), 313–338. doi:10.1007/s40279-013-0029-x
Caldwell A. R., Cheuvront S. N. (2019). Basic statistical considerations for physiology: the journal Temperature toolbox. Temperature 6 (3), 181–210. doi:10.1080/23328940.2019.1624131
Cheuvront S. N., Kenefick R. W., Montain S. J., Sawka M. N. (2010). Mechanisms of aerobic performance impairment with heat stress and dehydration. J. Appl. Physiol. 109 (6), 1989–1995. doi:10.1152/japplphysiol.00367.2010
Ciolac E. G., Mantuani S. S., Neiva C. M., Verardi C., Pessôa-Filho D. M., Pimenta L. (2015). Rating of perceived exertion as a tool for prescribing and self regulating interval training: a pilot study. Biol. Sport 32 (2), 103–108. doi:10.5604/20831862.1134312
Cohen J. (1988). Statistical power analysis for the behavioral sciences. Hillsdale, NJ: Lawrence Erlbaum.
Coyle E. F., Gonzalez-Alonso J. (2001). Cardiovascular drift during prolonged exercise: new perspectives. Exerc Sport Sci. Rev. 29 (2), 88–92. doi:10.1097/00003677-200104000-00009
Dill D. B., Costill D. L. (1974). Calculation of percentage changes in volumes of blood, plasma, and red cells in dehydration. J. Appl. Physiol. 37 (2), 247–248. doi:10.1152/jappl.1974.37.2.247
Dunbar C. C., Robertson R. J., Baun R., Blandin M. F., Metz K., Burdett R. A. Y., et al. (1992). The validity of regulating exercise intensity by ratings of perceived exertion. Med. Sci. Sports Exerc 24 (1), 94–99. doi:10.1249/00005768-199201000-00016
Edwards R. H., Melcher A., Hesser C. M., Wigertz O., Ekelund L. G. (1972). Physiological correlates of perceived exertion in continuous and intermittent exercise with the same average power output. Eur. J. Clin. Investig. 2, 108–114. doi:10.1111/j.1365-2362.1972.tb00578.x
Elliott-Sale K. J., Minahan C. L., Janse de Jonge X. A. K., Ackerman K. E., Sipilä S., Constantini N. W., et al. (2021). Methodological considerations for studies in sport and exercise science with women as participants: a working guide for standards of practice for research on women. Sports Med. 51 (5), 843–861. doi:10.1007/s40279-021-01435-8
Eston R. G., Williams J. G. (1988). Reliability of ratings of perceived effort regulation of exercise intensity. Br. J. Sports Med. 22 (4), 153–155. doi:10.1136/bjsm.22.4.153
Faul F., Erdfelder E., Buchner A., Lang A. G. (2009). Statistical power analyses using G*Power 3.1: tests for correlation and regression analyses. Behav. Res. Methods 41 (4), 1149–1160. doi:10.3758/BRM.41.4.1149
Faul F., Erdfelder E., Lang A. G., Buchner A. (2007). G*Power 3: a flexible statistical power analysis program for the social, behavioral, and biomedical sciences. Behav. Res. Methods 39 (2), 175–191. doi:10.3758/bf03193146
Fennell C. R. J., Hopker J. G. (2021). The acute physiological and perceptual effects of recovery interval intensity during cycling-based high-intensity interval training. Eur. J. Appl. Physiol. 121 (2), 425–434. doi:10.1007/s00421-020-04535-x
Foster C., Florhaug J. A., Franklin J., Gottschall L., Hrovatin L. A., Parker S., et al. (2001). A new approach to monitoring exercise training. J. Strength Cond. Res. 15 (1), 109–115. doi:10.1519/00124278-200102000-00019
Fritz C. O., Morris P. E., Richler J. J. (2012). Effect size estimates: current use, calculations, and interpretation. J. Exp. Psychol. 141 (1), 2–18. doi:10.1037/a0024338
Garber C. E., Blissmer B., Deschenes M. R., Franklin B. A., Lamonte M. J., Lee I. M., et al. (2011). American College of Sports Medicine position stand. Quantity and quality of exercise for developing and maintaining cardiorespiratory, musculoskeletal, and neuromotor fitness in apparently healthy adults: guidance for prescribing exercise. Med. Sci. Sports Exerc 43 (7), 1334–1359. doi:10.1249/MSS.0b013e318213fefb
Gorman A. J., Proppe D. W. (1984). Mechanisms producing tachycardia in conscious baboons during environmental heat stress. J. Appl. Physiol. 56 (2), 441–446. doi:10.1152/jappl.1984.56.2.441
Helgerud J., Høydal K., Wang E., Karlsen T., Berg P., Bjerkass M., et al. (2007). Aerobic high-intensity intervals improve
Jackson A. S., Pollock M. L. (1985). Practical assessment of body composition. Phys. Sportsmed. 13, 76–90. doi:10.1080/00913847.1985.11708790
Johnson E. C., Pryor R. R., Casa D. J., Ellis L. A., Maresh C. M., Pescatello L. S., et al. (2017). Precision, accuracy, and performance outcomes of perceived exertion vs. heart rate guided run-training. J. Strength Cond. Res. 31 (3), 630–637. doi:10.1519/JSC.0000000000001541
Kenny G. P., Jay O. (2013). Thermometry, calorimetry, and mean body temperature during heat stress. Compr. Physiol. 3 (4), 1689–1719. doi:10.1002/cphy.c130011
Kim Y. D., Lake R., Lees D. E., Schuette W. H., Bull J. M., Weise V., et al. (1979). Hemodynamic and plasma catecholamine responses to hyperthermic cancer therapy in humans. Am. J. Physiol. 237 (5), H570–H574. doi:10.1152/ajpheart.1979.237.5.H570
Lafrenz A. J., Wingo J. E., Ganio M. S., Cureton K. J. (2008). Effect of ambient temperature on cardiovascular drift and maximal oxygen uptake. Med. Sci. Sports Exerc 40 (6), 1065–1071. doi:10.1249/MSS.0b013e3181666ed7
Lakens D. (2013). Calculating and reporting effect sizes to facilitate cumulative science: a practical primer for t-tests and ANOVAs. Front. Psychol. 4 (863), 863. doi:10.3389/fpsyg.2013.00863
Maw G. J., Boutcher S. H., Taylor N. A. S. (1993). Ratings of perceived exertion and affect in hot and cool environments. Eur. J. Appl. Physiol. 67, 174–179. doi:10.1007/BF00376663
Maxwell N. S., Castle P. C., Spencer M. (2008). Effect of recovery intensity on peak power output and the development of heat strain during intermittent sprint exercise while under heat stress. J. Sci. Med. Sport 11 (5), 491–499. doi:10.1016/j.jsams.2007.05.008
Moore-Ede M. C., Czeisler C. A., Richardson G. S. (1983). Circadian timekeeping in health and disease. Part 1. Basic properties of circadian pacemakers. N. Engl. J. Med. 309, 469–476. doi:10.1056/NEJM198308253090806
Morales-Palomo F., Ramirez-Jimenez M., Ortega J. F., Pallares J. G., Mora-Rodriguez R. (2017). Cardiovascular drift during training for fitness in patients with metabolic syndrome. Med. Sci. Sports Exerc 49 (3), 518–526. doi:10.1249/MSS.0000000000001139
Mulholland A. M., Yoder H. A., Wingo J. E. (2023). Effect of work-to-rest cycles on cardiovascular strain and maximal oxygen uptake during heat stress. Int. J. Environ. Res. Public Health 20 (5), 4580. doi:10.3390/ijerph20054580
Nybo L., Jensen T., Nielsen B., Gonzalez-Alonso J. (2001). Effects of marked hyperthermia with and without dehydration on
Pandolf K. (1998). Perceived exertion during exercise in the heat, cold, or at high altitude. Natick, Massachusetts: U.S. Army Research Institute of Environmental Medicine USARIEM.
Périard J. D., Racinais S. (2015). Self-paced exercise in hot and cool conditions is associated with the maintenance of %
Ramanathan N. L. (1964). A new weighting system for mean surface temperature of the human body. J. Appl. Physiol. 19 (3), 531–533. doi:10.1152/jappl.1964.19.3.531
Roussey G., Gruet M., Vercruyssen F., Louis J., Vallier J.-M., Bernard T. (2018). Interactions between perceived exertion and thermal perception in the heat in endurance athletes. J. Therm. Biol. 76, 68–76. doi:10.1016/j.jtherbio.2018.07.006
Rowell L. B., Brengelmann G. L., Murray J. A., Kraning Ii K. K., Kusumi F. (1969). Human metabolic responses to hyperthermia during mild to maximal exercise. J. Appl. Physiol. 26, 395–402. doi:10.1152/jappl.1969.26.4.395
Rowell L. B., Marx H. J., Bruce R. J., Conn R. D., Kusumi F. (1966). Reductions in cardiac output, central blood volume, and stroke volume with thermal stress in normal men during exercise. J. Clin. Investig. 45, 1801–1816. doi:10.1172/JCI105484
Sawka M. N., Burke L. M., Eichner E. R., Maughan R. J., Montain S. J., Stachenfeld N. S., et al. (2007). American College of Sports Medicine position stand. Exercise and fluid replacement. Med. Sci. Sports Exerc 39 (2), 377–390. doi:10.1249/mss.0b013e31802ca597
Shea M. G., Headley S., Mullin E. M., Brawner C. A., Schilling P., Pack Q. R. (2022). Comparison of ratings of perceived exertion and target heart rate-based exercise prescription in cardiac rehabilitation: a randomized controlled pilot study. J. Cardiopulm. Rehabilitation Prev. 42, 352–358. doi:10.1097/HCR.0000000000000682
Stolwijk A. J., Hardy J. D. (1966). Partitional calorimetric studies of responses of man to thermal transients. J. Appl. Physiol. 21 (3), 967–977. doi:10.1152/jappl.1966.21.3.967
Stone T., Earley R. L., Burnash S. G., Wingo J. E. (2021). Menstrual cycle effects on cardiovascular drift and maximal oxygen uptake during exercise heat stress. Eur. J. Appl. Physiol. 121 (2), 561–572. doi:10.1007/s00421-020-04542-y
Swain D. P., Abernathy K. S., Smith C. S., Lee S. J., Bunn S. A. (1994). Target heart rates for the development of cardiorespiratory fitness. Med. Sci. Sports Exerc 26 (1), 112–116. doi:10.1249/00005768-199401000-00019
Thomas E. J., Pettitt R. W., Kramer M. (2020). High-intensity interval training prescribed within the secondary severe-intensity domain improves critical speed but not finite distance capacity. J. Sci. Med. Sport 2 (2), 154–166. doi:10.1007/s42978-020-00053-6
Tucker R., Marle T., Lambert E. V., Noakes T. D. (2006). The rate of heat storage mediates an anticipatory reduction in exercise intensity during cycling at a fixed rating of perceived exertion. J. Physiol. 574 (Pt 3), 905–915. doi:10.1113/jphysiol.2005.101733
Turkevich D., Micco A., Reeves J. T. (1988). Noninvasive measurement of the decrease in left ventricular filling time during maximal exercise in normal subjects. Am. J. Cardiol. 62, 650–652. doi:10.1016/0002-9149(88)90676-5
Ulmer H. V. (1996). Concept of an extracellular regulation of muscular metabolic rate during heavy exercise in humans by psychophysiological feedback. Experientia 52 (5), 416–420. doi:10.1007/BF01919309
Wingo J. E. (2015). Exercise intensity prescription during heat stress: a brief review. Scand. J. Med. Sci. Sports 25, 90–95. doi:10.1111/sms.12381
Wingo J. E., Cureton K. J. (2006a). Body cooling attenuates the decrease in maximal oxygen uptake associated with cardiovascular drift during heat stress. Eur. J. Appl. Physiol. 98 (1), 97–104. doi:10.1007/s00421-006-0249-y
Wingo J. E., Cureton K. J. (2006b). Maximal oxygen uptake after attenuation of cardiovascular drift during heat stress. Aviat. Space Environ. Med. 77, 687–694.
Wingo J. E., Ganio M. S., Cureton K. J. (2012). Cardiovascular drift during heat stress: implications for exercise prescription. Exerc Sport Sci. Rev. 40 (2), 88–94. doi:10.1097/JES.0b013e31824c43af
Wingo J. E., Lafrenz A. J., Ganio M. S., Edwards G. L., Cureton K. J. (2005). Cardiovascular drift is related to reduced maximal oxygen uptake during heat stress. Med. Sci. Sports Exerc 37 (2), 248–255. doi:10.1249/01.mss.0000152731.33450.95
Wingo J. E., Stone T., Ng J. (2020). Cardiovascular drift and maximal oxygen uptake during running and cycling in the heat. Med. Sci. Sports Exerc 52 (9), 1924–1932. doi:10.1249/MSS.0000000000002324
Yoder H. A., Mulholland A. M., MacDonald H. V., Wingo J. E. (2023). Acute work rate adjustments during high-intensity interval training in a hot and temperate environment. Appl. Physiol. Nutr. Metab. 48, 962–973. doi:10.1139/apnm-2023-0144
Yoder H. A., Wingo J. E., Leeper J., MacDonald H. V., Mota J., Richardson M. (2022). High-intenstiy interval training in the heat. Tuscaloosa, AL: The University of Alabama.
Keywords: exercise prescription, HIIT, cardiovascular drift, power output, heat stress, target heart rate, rating of perceived exertion
Citation: Yoder HA, Mulholland AM, MacDonald HV and Wingo JE (2025) Work rate adjustments needed to maintain heart rate and RPE during high-intensity interval training in the heat. Front. Physiol. 16:1506325. doi: 10.3389/fphys.2025.1506325
Received: 04 October 2024; Accepted: 16 January 2025;
Published: 06 February 2025.
Edited by:
Courteney Leigh Benjamin, Samford University, United StatesReviewed by:
Paulo A. S. Armada-da-Silva, University of Lisbon, PortugalSamuel Honório, Polytechnic Institute of Castelo Branco, Portugal
Copyright © 2025 Yoder, Mulholland, MacDonald and Wingo. This is an open-access article distributed under the terms of the Creative Commons Attribution License (CC BY). The use, distribution or reproduction in other forums is permitted, provided the original author(s) and the copyright owner(s) are credited and that the original publication in this journal is cited, in accordance with accepted academic practice. No use, distribution or reproduction is permitted which does not comply with these terms.
*Correspondence: Hillary A. Yoder, aHlvZGVyQG5tc3UuZWR1