- Department of Cardiovascular Medicine, Tokushima University Graduate School of Biomedical Sciences, Tokushima, Japan
Lifestyle-related diseases, such as atherosclerosis and diabetes, are now considered to be a series of diseases caused by chronic inflammation. Adipose tissue is considered to be an endocrine organ that not only plays a role in lipid storage, heat production, and buffering, but also produces physiologically active substances and is involved in chronic inflammation. Perivascular adipose tissue (PVAT) surrounding blood vessels similarly produces inflammatory and anti-inflammatory physiologically active substances that act on blood vessels either directly or via the bloodstream. Epicardial adipose tissue (EAT), which is in direct contact with the coronary arteries inside the pericardium, is thought to have a direct effect on the coronary arteries as well. The presence and inflammatory status of these adipose tissues can be evaluated by imaging tests, and has been shown to be associated with the presence of current cardiovascular disease (CVD) and to be a prognostic factor. It is also expected to become a new diagnostic and therapeutic target for CVD.
1 Introduction
Cardiovascular disease (CVD), diabetes, and chronic kidney disease are recognized to be based on chronic inflammation (Furman et al., 2019). In addition, adipose tissue has recently been shown to not only play a role in energy storage, heat production, and cushioning between tissues, but also as an endocrine organ, secreting inflammatory and anti-inflammatory physiologically active substances that are involved in chronic inflammation (Matsuzawa et al., 2004). Recently, several reports have suggested a relationship between epicardial adipose tissue (EAT) and CVD (Tanaka et al., 2020; Wang et al., 2022). In 2023, the European Society of Cardiology reported a statement on the use of EAT as a therapeutic indicator and biomarker in clinical practice (Antoniades et al., 2023). This article mainly reviews the relationship between CVD and EAT and its potential as a therapeutic target.
2 Terminological definition of perivascular fat
A proposal by the European Society of Cardiology defined the names of each adipose tissue in 2023 (Antoniades et al., 2023) (Left side of Figure 1). Thoracic visceral adipose tissue (VAT) includes the EAT, which is enclosed between the cardiac surface and the visceral pericardium, the pericardial adipose tissue (external to the pericardium and surrounding the cardiac silhouette), as well as the non-pericardial thoracic adipose tissue (located anywhere inside the thoracic cavity, but outside the pericardium) (Antonopoulos and Antoniades, 2017). Perivascular adipose tissue (PVAT) around coronary arteries has distinct biological properties compared to the rest of the EAT far from the arterial wall (Antonopoulos et al., 2017; Costa et al., 2018; Antoniades et al., 2020; Turaihi et al., 2020). However, within EAT there is a gradual transition between PVAT and non-PVAT, with no anatomical structures separating the two, and a definition must be agreed upon. Indeed, in recent literature, PVAT has been defined as a layer of adipose tissue located within a distance equal to the luminal diameter of the artery, and this definition was adopted by the working group (Antoniades et al., 2020). This definition applies to PVAT surrounding human arteries with a lumen diameter of up to 2 cm. For arteries with a lumen diameter greater than 2 cm (such as the aorta), PVAT extends up to 2 cm from the external surface of the vessel.
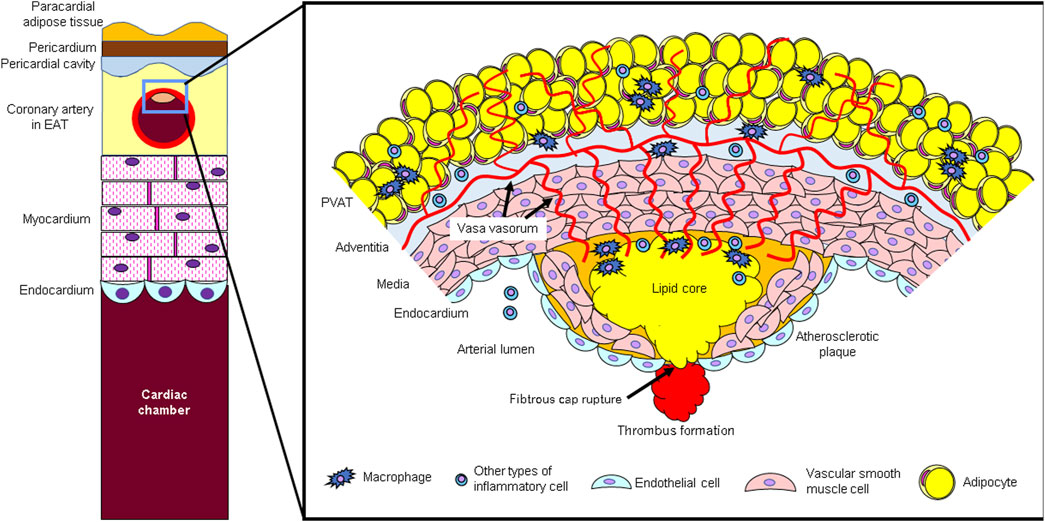
Figure 1. (Left side) Schematic diagram of epicardial adipose tissue (EAT) and coronary arteries. Epicardial adipose tissue (EAT) is located in the pericardial cavity, directly in contact with the coronary arteries, and adjacent to the myocardium. (Right side) Atherosclerotic lesion and perivascular adipose tissue (PVAT). PVAT secretes inflammatory cytokines. Bioactive agents secreted from PVAT act not only directly on blood vessels but also through adventitial vasa vasorum (VV).
3 Adventitial environment of blood vessels with atherosclerotic lesions
Atherosclerosis is caused when the homeostatic function of vascular endothelial cells is impaired and inflammatory cells infiltrate under the endothelium (Ross, 1999) (Figure 2A). Therefore, it has been thought that inflammation occurs from the luminal side and spreads to the adventitial side. However, more recent findings suggest a pathway through which inflammation on the adventitial side spreads to the lumen side (Figures 2B, C).
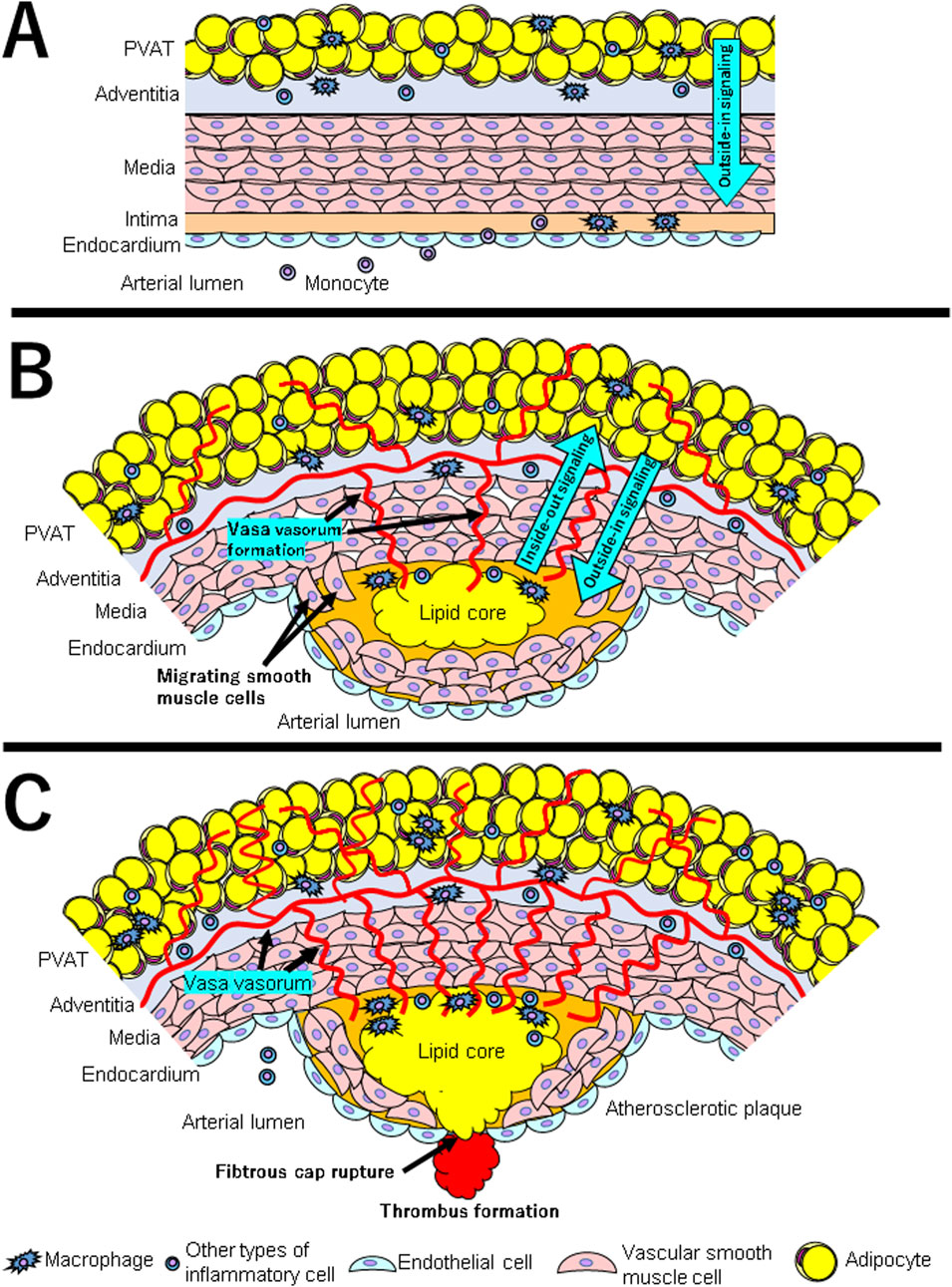
Figure 2. (A–C) show the interaction between PVAT and vascular wall at each stage in the development of atherosclerosis. (A) The first steps include adhesion of blood leukocytes to a monolayer of activated endothelial cells, migration of bound leukocytes to the intima, maturation of monocytes into macrophages and their lipid uptake to generate foam cells. Under normal circumstances, PVAT induces vasodilation and anti-inflammatory effects through the release of adipokines such as adiponectin and vasodilators such as nitric oxide (displayed as “Outside-in Signaling” with blue arrow). (B) Under inflammatory conditions such as obesity, PVAT produces adipokines such as leptin, which induces vasoconstriction, smooth muscle cell migration, and endothelial dysfunction. Pro-inflammatory cytokines are produced by PVAT macrophages and other inflammatory cells. At the same time, vasa vasorum is formed and invaded into intima. Inflammatory cytokines and chemokines produced by PVAT either infiltrate directly into the intima or enter through vasa vasorum (displayed as “Outside-in Signaling” with blue arrow). The inflammatory condition of vessel wall influences PVAT through paracrine signals that cause changes in the PVAT secretion phenotype (displayed as “Inside-out signaling” with blue arrow). (C) As a result of the interaction between chronic inflammatory condition in both PVAT and vascular wall, the atherosclerotic plaques gradually become unstable. Inflammation is a major factor in the thinning of the fibrous cap and the rupture of atherosclerotic plaques. Thrombosis complicates physical disruption of the atherosclerotic plaque. Fracture of the cap exposes blood coagulant components to tissue factors in the plaque, triggering occlusive thrombus formation that limits blood flow.
Most arteries, except cerebral arteries and microvessels, are surrounded by perivascular adipose tissue (PVAT) (Szasz et al., 2013). Although PVAT has been thought to act as a mechanical cushion for supporting tissues and the vasculature, recent studies have shown that PVAT secretes adipokines, including inflammatory cytokines and chemokines. Under normal circumstances, PVAT induces vasodilation and anti-inflammatory effects through the release of adipokines (such as adiponectin) and vasodilators (such as nitric oxide) (Akoumianakis et al., 2017) (Figure 2A). Under inflammatory conditions such as obesity, PVAT produces adipokines such as leptin, which induces vasoconstriction, SMC migration, and endothelial dysfunction (Kim et al., 2020; Ichikawa et al., 2023). Pro-inflammatory cytokines are produced by PVAT macrophages and other inflammatory cells. Therefore, they cause endothelial dysfunction and atherosclerotic plaque formation (Chen et al., 2010) (Figure 2B). Recent evidence also supports that the relationship between PVAT and vessel walls is bidirectional. This is because the inflammatory condition of vessel wall influences PVAT through paracrine signals that cause changes in the PVAT secretion phenotype (Oikonomou and Antoniades, 2019).
Accumulating evidence highlights the role of adipocytes as secreting cells of exosomes that convey miRNAs with either pro-atherosclerotic or anti-atherosclerotic effects. The expression of MiR-133, miR-21, and miR-143 is significantly decreased in PVAT around segments with occlusive coronary plaques (Marketou et al., 2023). The expression of pro-inflammatory miR-103-3p was higher in coronary PVAT of CAD patients, while PVAT-derived miR-382-5p suppressed foam cell formation (Vacca et al., 2016; Liu et al., 2022).
Microvessels present in the adventitia of blood vessels (vasa vasorum; VV) develop in the adventitia of atherosclerotic lesions due to hypoxic stimulation, destroy the tunica media, invade into the plaque, and play a role in inflammatory cell infiltration (Moreno et al., 2004; Phillippi, 2022). These newly proliferated blood vessels within the plaque lack pericytes and are prone to rupture, resulting in intraplaque hemorrhage (Sluimer et al., 2009; Phillippi, 2022). In this way, inflammation from the adventitia side spreads to the luminal side, promoting plaque progression and instability. Adipocytokines secreted by perivascular adipose tissue (PVAT) are thought to infiltrate directly into the vascular wall and also enter the plaque using the VV as a conduit (Sacks and Fain, 2007; Tanaka et al., 2020; Wang et al., 2022) (Right side of Figure 1).
4 Relationship between inflammation in EAT and CVD
It is reported that EAT in patients undergoing coronary artery bypass graft (CABG) highly expressed several inflammatory cytokines and chemokines, such as interleukin (IL)-6 and tumor necrosis factor (TNF)-α compared with their subcutaneous fat (Mazurek et al., 2003) or compared with EAT in non-CAD patients (Hirata et al., 2011b). Our observational studies revealed that EAT volume, macrophage and inflammatory cytokine content in EAT, and the decrease in adiponectin expression in EAT were risk factors for coronary artery disease requiring CABG (Shimabukuro et al., 2013).
Some clinical studies examining the relationship between EAT levels and blood biomarkers have found that there was an inverse correlation with the blood concentration of apeline, which has a vasodilatory effect (Babapour et al., 2024), and a positive correlation with the blood concentration of branched-chain amino acids (Zhao et al., 2023). Recent clinical research has shown that EAT thickness and/or volume, including those calculated using AI-based deep learning, are predictive factors for increased coronary artery lipid plaque volume (Amangurbanova et al., 2024), the onset of CVD such as coronary artery disease (Mahabadi et al., 2013; Christensen et al., 2019b; Commandeur et al., 2020; Eisenberg et al., 2020; Filtz et al., 2024; Gaborit et al., 2024; Rämö et al., 2024), and MACE (Guglielmo et al., 2024).
In recent basic and translational research on EAT, pan-genomic microarray analysis has shown that EAT have a profile similar to that of beige adipocytes (Doukbi et al., 2024). In mouse models, it has been confirmed that EAT accumulation affects the inflammatory phenotype of cardiac macrophages and induces microvascular occlusion (MVO) (Zhao et al., 2024). Recently, single-cell transcriptome analysis of human normal and pathological EAT tissues was performed, which might provide further detailed mechanisms serve as future therapeutic targets (Liu X. et al., 2024).
Furthermore, our previous studies evaluated the relationship of local inflammation in EAT, intraplaque microluminal structure (determined by optical coherence tomography; OCT) and coronary plaque characteristics (determined by integrated backscatter intravascular ultrasound; IB-IVUS) in fresh cadavers. The results showed that coronary arteries with intraplaque microluminal structure had relatively lipid-rich plaques and increased expression of the inflammatory molecules in EAT compared to coronary arteries without microluminal structure (Ito et al., 2020; Kawabata et al., 2023), suggesting the presence of luminal structures within plaques contribute to plaque instability.
5 Quantification of inflammation in coronary artery PVAT
In 2017, anti-inflammatory therapy targeting the IL-1β immune pathway with canakinumab for secondary prevention of myocardial infarction significantly reduced the recurrence rate of cardiovascular events, independent of lower lipid levels (CANTOS study), suggesting the relationship between chronic inflammation and CVD (Ridker et al., 2017). Also in 2017, it was reported that the state of inflammation in coronary artery PVAT can be evaluated using the fatty line attenuation coefficient (FAI), which can be obtained from coronary artery CT images (Antonopoulos et al., 2017). Computed tomography is useful for characterization as morphological changes associated with inflammation can be detected by the gradient of CT signal attenuation in PVAT [-190 to −30 Hounsfield units (HU)] (Antonopoulos et al., 2017; Theofilis et al., 2022). Indeed, lipolysis in PVAT adipocytes and adipocyte dedifferentiation induced by proinflammatory cytokines lead to an increase in the water/lipid ratio in PVAT close to the inflamed vessel wall. Consequently, these morphological changes increase the PVAT mean CT attenuation towards −30 Hounsfield units (HU) (Antonopoulos et al., 2017). The FAI of coronary PVAT in patients with CAD was consciously higher than in patients without CAD. Currently, FAI is considered to be an imaging index that reflects changes in the size of perivascular adipocytes during inflammation, and its clinical application is expected (Antonopoulos et al., 2017).
6 Association between perivascular FAI of coronary artery and CVD
The group above mentioned conducted two independent prospective cohort studies of symptomatic patients who underwent coronary artery CT examination to investigate the association between FAI at the root of the right coronary artery, which was most strongly associated with event onset, and all-cause mortality and cardiac death (CRISP-CT study) (Oikonomou et al., 2018). In both cohorts, FAI at the root of the right coronary artery was associated with all-cause and cardiac death, independent of conventional risk factors such as age, sex, and cardiovascular risk factors; The cutoff value was −70.1 Hounsfield units (HU), which was confirmed in the validation cohort. This group also proposed a new method using artificial intelligence (AI) to predict cardiovascular risk by analyzing the radiomic profile obtained from radiographic images of coronary artery PVAT (Oikonomou et al., 2019).
Accumulating recent clinical research have suggested a correlation between coronary artery FAI and control level of diabetes (Liu Y. et al., 2024), control level of dyslipidemia (Feng et al., 2024), coronary flow reserve (CFR) (Chen et al., 2024), plaque instability (Sagris et al., 2022; Antonopoulos and Simantiris, 2023; Kuneman et al., 2023; Wang et al., 2024), future percutaneous coronary intervention (He et al., 2024), incidence of coronary restenosis (Qin et al., 2022), and coronary bypass graft occlusion (Han et al., 2024; Huang et al., 2024). It should be noted that there may be differences between men and women regarding the characteristics of FAI (Kinoshita et al., 2024). Although many retrospective and prospective observational studies have suggested a correlation between coronary FAI and future incidence of MACE (Ichikawa et al., 2022; Kato et al., 2022; Chan et al., 2024; Choi et al., 2024; Zhan et al., 2024), there are also negative reports (van Rosendael et al., 2024; Yang et al., 2024). Alternatively, there is also literature showing that lesion-specific pericoronary FAI is a predictive factor for MACE (Liu M. et al., 2024). Currently, a large-scale prospective cohort study is underway in a multiethnic and multinational country in Asia, and will evaluate the association between AI-based coronary artery FAI and clinical endpoints such as cardiovascular events, hospitalization, and mortality (Baskaran et al., 2024). The 2023 European Society of Cardiology Recommendation document summarizes imaging methods for EAT and coronary PVAT, including FAI (Antoniades et al., 2023).
7 Treatments targeting EAT and coronary PVAT
If accumulation of EAT, coronary PVAT, is associated with cardiovascular events, treatment targeting PVAT resuction may potentially suppress the onset of CVD. We summarize treatments in which EAT, including coronary PVAT, is described as the potential therapeutic target.
7.1 Aerobic exercise
In a larger, single-center, double-blind, randomized exercise intervention study, 52 abdominally obese but otherwise healthy participants randomly assigned to moderate/high-intensity aerobic exercise (3 sessions of 45 min per week reaching 70%–85% of Vo2max) or no exercise with or without monthly infusions of the IL-6 receptor antagonist tocilizumab in the clinical standard dose of 8 mg/kg or saline (placebo). When comparing the EAT volume evaluated by MRI, a clear decrease in EAT volume was observed in the aerobic exercise group, but this effect was not observed in the tocilizumab administration group, suggesting the decrease in EAT volume due to aerobic exercise was mediated by IL-6 (Christensen et al., 2019a). A meta-analysis of five randomized controlled trials that observed whether exercise reduced EAT found that exercise significantly reduced EAT and waist circumference (Colonetti et al., 2021). The exercises used in these studies included in the meta-analysis were aerobic exercise and/or resistance circuit training, and the exercise frequency was 2–3 times a week, so exercises of such kind, intensity, and frequency might be incorporated into clinical recommendations to manage CVD risks associated with EAT. However, this report has limitations, such as insufficient blinding in the original study, and further research needs to be conducted with a more appropriate design and method to clarify the relationship between exercise and EAT. Specifically, further comparative verifications are required, including randomized controlled trials with a larger number of subjects, comparisons of exercise intensity and frequency, evaluation of the volume and feature of EAT using various imaging tests, and long-term cardiovascular prognosis. As an underlying mechanism, single-nucleus transcriptomics of epicardial adipose tissue from female pigs revealed the effects of exercise training on resident innate and adaptive immune cells (Ahmad et al., 2024).
7.2 Statin
In a sub-analysis of the BELLES study, which investigated whether moderate to high-dose statin administration improves coronary artery calcification in postmenopausal women, EAT volume was measured using CT. In the high-dose (atorvastatin 80 mg) group, a statistically significant decrease in EAT volume was observed after 1 year, and this was independent of the lipid-lowering effect (Alexopoulos et al., 2013). It was also reported that the FAI of PVAT in coronary arteries with non-calcified plaques was significantly reduced by 1 year of statin administration (Dai et al., 2020). Based on these results, the effects of statins on CVD may also include reducing EAT volume and suppressing inflammation.
7.3 SGLT2 inhibitor
Our research group compared the thickness of the EAT in type 2 diabetic patients by using echocardiography before and after treatment with canagliflozin, a SGLT2 (sodium-glucose co-transporter 2) inhibitor. The results showed that EAT thickness was significantly reduced, independent of the reduction in HbA1c levels (Yagi et al., 2017). Similar effects have been reported with ipragliflozin (Fukuda et al., 2017), luseoflozin (Bouchi et al., 2017), and dapagliflozin (Sato et al., 2018). Although the scale of individual observational studies and randomized controlled trials was small and some results were controversial, recent meta-analyses have shown that SGLT2 inhibitors significantly reduce EAT volumes in patients with type 2 diabetes and obesity (Cinti et al., 2023; Myasoedova et al., 2023; Bao et al., 2024). As for the more basic pathophysiology, recent basic research has revealed that empagliflozin suppresses the differentiation and maturation of human epicardial adipocytes, and this mechanism is at least partially responsible for the reduction in EAT levels induced by SGLT2 inhibitors (Takano et al., 2023).
7.4 GLP-1 receptor agonist
Recently, the efficacy and safety of glucagon-like peptide (GLP)-1 receptor agonists for the treatment of type 2 diabetes have been reported (Karagiannis et al., 2024). Some reports have shown that liraglutide, a GLP-1 receptor agonist, reduced EAT volume in type 2 diabetes (Dutour et al., 2016; Iacobellis et al., 2017), although others have shown no significant changes (van Eyk et al., 2019; Bizino et al., 2020). In recent years, many meta-analyses integrating observational studies and RCTs have been conducted, and it has been shown that GLP-1 receptor agonists significantly reduce the amount of EAT in obese patients and type 2 diabetic patients (Berg et al., 2022; Akoumianakis et al., 2023; Myasoedova et al., 2023; Bao et al., 2024). Furthermore, a recent retrospective observational study revealed an independent association between coronary FAI and semaglutide treatment, which is a widely used GLP-1 analogue (Li et al., 2024). The substudy of the randomized controlled trial (SUMMIT trial) also demonstrated that tirzepatide therapy, a commonly used GLP-1 receptor agonist, in obesity-related HFpEF led to reduced LV mass and EAT as compared to placebo (Kramer et al., 2024).
7.5 Anti-inflammatory drug]
Recently, it has been reported that anti-inflammatory drugs such as canakinumab (CANTOS trial) (Ridker et al., 2017) or small doses of colchicine (Nidorf et al., 2020) reduced coronary events in patients with chronic CAD. The former, the CANTOS trial, is a randomized controlled trial of canakinumab, an anti-IL-1β therapy, versus placebo for the secondary prevention of patients with myocardial infarction. Canakinumab significantly reduced the recurrence rate of cardiovascular events, independent of lower lipid levels, although canakinumab was associated with a higher incidence of fatal infection than was placebo (Ridker et al., 2017). The latter study of low-dose colchicine (0.5 mg once daily) was a randomized controlled trial versus placebo for major cardiovascular events in patients with chronic coronary artery disease. In this trial, the risk of cardiovascular events was significantly lower in patients who received colchicine than in patients who received placebo, whereas the incidence of death from non-cardiovascular causes was significantly lower than in patients who received placebo (Nidorf et al., 2020). Inflammation in EAT may be a target for such anti-inflammatory drugs. It is expected that the relationship between anti-inflammatory drugs and FAI will be elucidated through randomized clinical trials in the future. However, they also need to be carefully evaluated, including concerns about potential adverse effects such as infections due to their anti-inflammatory properties.
8 Discussion
In this review, we have summarized recent findings regarding the role of EAT and PVAT in the pathogenesis of atherosclerosis. Adipose tissue serves not only as an energy storage or mechanical cushion, but also as an endocrine organ. Recent evidence has revealed that perivascular adipose tissue is involved in vascular homeostasis and adjacent arterial pathophysiology by producing various adipokines (Soltis and Cassis, 1991; Löhn et al., 2002). EAT is located between the surface of the heart and the visceral layer of the pericardium and surrounds the coronary arteries. Many clinical studies suggest that an increase in EAT volume is associated with CAD (Dagvasumberel et al., 2012; Shimabukuro et al., 2013; Hirata et al., 2015; Yamada and Sata, 2015). We also reported that inflammation is enhanced in the EAT of patients with CAD (Hirata et al., 2011a; Hirata et al., 2011b), suggesting that EAT plays a crucial role in the pathogenesis of coronary atherosclerosis (Tanaka and Sata, 2018). It has been reported that exercise and some antidiabetic drugs can reduce EAT volume. Although this article mainly discussed coronary artery disease, EAT has also been reported to be associated with atrial fibrillation and heart failure (Tanaka and Sata, 2018; Elsanhoury et al., 2021). EAT and coronary PVAT may become new therapeutic targets or part of existing therapeutic targets. In particular, the CVD suppressing effects of anti-inflammatory drugs are attracting attention, and elucidation of the relationship between EAT and inflammation is expected in the future.
9 Conclusion
Accumulating evidence suggests that EAT, coronary PVAT, may represent new prognostic factors, new therapeutic targets, or part of existing therapeutic targets. In particular, the CVD suppressing effects of anti-inflammatory drugs are attracting attention, and elucidation of the relationship between EAT and inflammation is expected in the future.
Author contributions
TH: Writing–original draft, Writing–review and editing. MS: Supervision, Writing–review and editing.
Funding
The author(s) declare that financial support was received for the research, authorship, and/or publication of this article. This work was partially supported by JSPS Kakenhi Grants (Number 23K15132 to TH, 22H03069 to MS), Bayer Vascular Frontiers Research Grant (MS), and Japan Agency for Medical Research and Development (MS). The funders had no role in the study design, data collection and analysis, or manuscript preparation.
Acknowledgments
The authors thank Etsuko Uematsu for technical assistance.
Conflict of interest
The authors declare that the research was conducted in the absence of any commercial or financial relationships that could be construed as a potential conflict of interest.
Generative AI statement
The author(s) declare that no Generative AI was used in the creation of this manuscript.
Publisher’s note
All claims expressed in this article are solely those of the authors and do not necessarily represent those of their affiliated organizations, or those of the publisher, the editors and the reviewers. Any product that may be evaluated in this article, or claim that may be made by its manufacturer, is not guaranteed or endorsed by the publisher.
References
Ahmad I., Gupta S., Faulkner P., Mullens D., Thomas M., Sytha S. P., et al. (2024). Single-nucleus transcriptomics of epicardial adipose tissue from female pigs reveals effects of exercise training on resident innate and adaptive immune cells. Cell Commun. Signal 22 (1), 243. doi:10.1186/s12964-024-01587-w
Akoumianakis I., Tarun A., Antoniades C. (2017). Perivascular adipose tissue as a regulator of vascular disease pathogenesis: identifying novel therapeutic targets. Br. J. Pharmacol. 174 (20), 3411–3424. doi:10.1111/bph.13666
Akoumianakis I., Zagaliotis A., Konstantaraki M., Filippatos T. D. (2023). GLP-1 analogs and regional adiposity: a systematic review and meta-analysis. Obes. Rev. 24 (8), e13574. doi:10.1111/obr.13574
Alexopoulos N., Melek B. H., Arepalli C. D., Hartlage G. R., Chen Z., Kim S., et al. (2013). Effect of intensive versus moderate lipid-lowering therapy on epicardial adipose tissue in hyperlipidemic post-menopausal women: a substudy of the BELLES trial (Beyond Endorsed Lipid Lowering with EBT Scanning). J. Am. Coll. Cardiol. 61 (19), 1956–1961. doi:10.1016/j.jacc.2012.12.051
Amangurbanova M., Daher R., Asbeutah A. A., Vemuri B., Mirza H., Waseem S., et al. (2024). Higher epicardial adipose tissue volume is associated with higher coronary fatty plaque volume and is regulated by waist circumference but not EPA+DHA supplementation. J. Clin. Lipidol. 18 (5), e773–e786. doi:10.1016/j.jacl.2024.06.006
Antoniades C., Antonopoulos A. S., Deanfield J. (2020). Imaging residual inflammatory cardiovascular risk. Eur. Heart J. 41 (6), 748–758. doi:10.1093/eurheartj/ehz474
Antoniades C., Tousoulis D., Vavlukis M., Fleming I., Duncker D. J., Eringa E., et al. (2023). Perivascular adipose tissue as a source of therapeutic targets and clinical biomarkers. Eur. Heart J. 44 (38), 3827–3844. doi:10.1093/eurheartj/ehad484
Antonopoulos A. S., Antoniades C. (2017). The role of epicardial adipose tissue in cardiac biology: classic concepts and emerging roles. J. Physiol. 595 (12), 3907–3917. doi:10.1113/jp273049
Antonopoulos A. S., Sanna F., Sabharwal N., Thomas S., Oikonomou E. K., Herdman L., et al. (2017). Detecting human coronary inflammation by imaging perivascular fat. Sci. Transl. Med. 9 (398), eaal2658. doi:10.1126/scitranslmed.aal2658
Antonopoulos A. S., Simantiris S. (2023). Detecting the vulnerable patient: toward preventive imaging by coronary computed tomography angiography. Circ. Cardiovasc Imaging 16 (2), e015135. doi:10.1161/circimaging.122.015135
Babapour B., Doustkami H., Avesta L., Kiamehr P., Aslani M. R. (2024). Negative association of apelin plasma levels with epicardial fat thickness in patients with stable angina and acute myocardial infarction: a case-control study. J. Res. Med. Sci. 29, 26. doi:10.4103/jrms.jrms_478_22
Bao Y., Hu Y., Shi M., Zhao Z. (2024). SGLT2 inhibitors reduce epicardial adipose tissue more than GLP-1 agonists or exercise interventions in patients with type 2 diabetes mellitus and/or obesity: a systematic review and network meta-analysis. Diabetes Obes. Metab. doi:10.1111/dom.16107
Baskaran L., Leng S., Dutta U., Teo L., Yew M. S., Sia C. H., et al. (2024). Cohort profile: AI-driven national Platform for CCTA for clinicaL and industriaL applicatiOns (APOLLO). BMJ Open 14 (12), e089047. doi:10.1136/bmjopen-2024-089047
Berg G., Barchuk M., Lobo M., Nogueira J. P. (2022). Effect of glucagon-like peptide-1 (GLP-1) analogues on epicardial adipose tissue: a meta-analysis. Diabetes Metab. Syndr. 16 (7), 102562. doi:10.1016/j.dsx.2022.102562
Bizino M. B., Jazet I. M., de Heer P., van Eyk H. J., Dekkers I. A., Rensen P. C. N., et al. (2020). Placebo-controlled randomised trial with liraglutide on magnetic resonance endpoints in individuals with type 2 diabetes: a pre-specified secondary study on ectopic fat accumulation. Diabetologia 63 (1), 65–74. doi:10.1007/s00125-019-05021-6
Bouchi R., Terashima M., Sasahara Y., Asakawa M., Fukuda T., Takeuchi T., et al. (2017). Luseogliflozin reduces epicardial fat accumulation in patients with type 2 diabetes: a pilot study. Cardiovasc Diabetol. 16 (1), 32. doi:10.1186/s12933-017-0516-8
Chan K., Wahome E., Tsiachristas A., Antonopoulos A. S., Patel P., Lyasheva M., et al. (2024). Inflammatory risk and cardiovascular events in patients without obstructive coronary artery disease: the ORFAN multicentre, longitudinal cohort study. Lancet 403 (10444), 2606–2618. doi:10.1016/s0140-6736(24)00596-8
Chen C., Jiang J., Lü J. M., Chai H., Wang X., Lin P. H., et al. (2010). Resistin decreases expression of endothelial nitric oxide synthase through oxidative stress in human coronary artery endothelial cells. Am. J. Physiol. Heart Circ. Physiol. 299 (1), H193–H201. doi:10.1152/ajpheart.00431.2009
Chen M., Liu B., Li X., Li D., Fan L. (2024). Relationship between peri-coronary inflammation and coronary vascular function in patients with suspected coronary artery disease. Front. Cardiovasc Med. 11, 1303529. doi:10.3389/fcvm.2024.1303529
Choi Y. J., Yang S., West H., Tomlins P., Hoshino M., Murai T., et al. (2024). Association of coronary inflammation with plaque vulnerability and fractional flow reserve in coronary artery disease. J. Cardiovasc Comput. Tomogr. doi:10.1016/j.jcct.2024.10.013
Christensen R. H., Lehrskov L. L., Wedell-Neergaard A. S., Legaard G. E., Ried-Larsen M., Karstoft K., et al. (2019a). Aerobic exercise induces cardiac fat loss and alters cardiac muscle mass through an interleukin-6 receptor-dependent mechanism: cardiac analysis of a double-blind randomized controlled clinical trial in abdominally obese humans. Circulation 140 (20), 1684–1686. doi:10.1161/circulationaha.119.042287
Christensen R. H., von Scholten B. J., Hansen C. S., Jensen M. T., Vilsbøll T., Rossing P., et al. (2019b). Epicardial adipose tissue predicts incident cardiovascular disease and mortality in patients with type 2 diabetes. Cardiovasc Diabetol. 18 (1), 114. doi:10.1186/s12933-019-0917-y
Cinti F., Leccisotti L., Sorice G. P., Capece U., D'Amario D., Lorusso M., et al. (2023). Dapagliflozin treatment is associated with a reduction of epicardial adipose tissue thickness and epicardial glucose uptake in human type 2 diabetes. Cardiovasc Diabetol. 22 (1), 349. doi:10.1186/s12933-023-02091-0
Colonetti T., Grande A. J., Amaral M. C., Colonetti L., Uggioni M. L., da Rosa M. I., et al. (2021). Effect of exercise on epicardial adipose tissue in adults: a systematic review and meta-analyses. Heart Fail Rev. 26 (6), 1399–1411. doi:10.1007/s10741-020-09965-5
Commandeur F., Slomka P. J., Goeller M., Chen X., Cadet S., Razipour A., et al. (2020). Machine learning to predict the long-term risk of myocardial infarction and cardiac death based on clinical risk, coronary calcium, and epicardial adipose tissue: a prospective study. Cardiovasc Res. 116 (14), 2216–2225. doi:10.1093/cvr/cvz321
Costa R. M., Neves K. B., Tostes R. C., Lobato N. S. (2018). Perivascular adipose tissue as a relevant fat depot for cardiovascular risk in obesity. Front. Physiol. 9, 253. doi:10.3389/fphys.2018.00253
Dagvasumberel M., Shimabukuro M., Nishiuchi T., Ueno J., Takao S., Fukuda D., et al. (2012). Gender disparities in the association between epicardial adipose tissue volume and coronary atherosclerosis: a 3-dimensional cardiac computed tomography imaging study in Japanese subjects. Cardiovasc Diabetol. 11, 106. doi:10.1186/1475-2840-11-106
Dai X., Yu L., Lu Z., Shen C., Tao X., Zhang J. (2020). Serial change of perivascular fat attenuation index after statin treatment: insights from a coronary CT angiography follow-up study. Int. J. Cardiol. 319, 144–149. doi:10.1016/j.ijcard.2020.06.008
Doukbi E., Ancel P., Dutour A., Soghomonian A., Ahmed S., Castejon V., et al. (2024). Human epicardial fat has a beige profile and contains higher type 2 innate lymphoid cells than subcutaneous fat. Obes. (Silver Spring) 32 (7), 1302–1314. doi:10.1002/oby.24023
Dutour A., Abdesselam I., Ancel P., Kober F., Mrad G., Darmon P., et al. (2016). Exenatide decreases liver fat content and epicardial adipose tissue in patients with obesity and type 2 diabetes: a prospective randomized clinical trial using magnetic resonance imaging and spectroscopy. Diabetes Obes. Metab. 18 (9), 882–891. doi:10.1111/dom.12680
Eisenberg E., McElhinney P. A., Commandeur F., Chen X., Cadet S., Goeller M., et al. (2020). Deep learning-based quantification of epicardial adipose tissue volume and attenuation predicts major adverse cardiovascular events in asymptomatic subjects. Circ. Cardiovasc Imaging 13 (2), e009829. doi:10.1161/circimaging.119.009829
Elsanhoury A., Nelki V., Kelle S., Van Linthout S., Tschöpe C. (2021). Epicardial fat expansion in diabetic and obese patients with heart failure and preserved ejection fraction-A specific HFpEF phenotype. Front. Cardiovasc Med. 8, 720690. doi:10.3389/fcvm.2021.720690
Feng Y. S., Sun Z. Y., Jiang F., Ma P. C., Liu X. R., Meng Y. Y., et al. (2024). Association between pericoronary fat attenuation index as evaluated by coronary artery CT angiography and clinical interventions in lipid management among patients with coronary artery disease. Int. J. Gen. Med. 17, 4937–4946. doi:10.2147/ijgm.S468768
Filtz A., Lorenzatti D., Scotti A., Piña P., Fernandez-Hazim C., Huang D., et al. (2024). Relationship between epicardial adipose tissue and coronary atherosclerosis by CCTA in young adults (18-45). Am. J. Prev. Cardiol. 19, 100711. doi:10.1016/j.ajpc.2024.100711
Fukuda T., Bouchi R., Terashima M., Sasahara Y., Asakawa M., Takeuchi T., et al. (2017). Ipragliflozin reduces epicardial fat accumulation in non-obese type 2 diabetic patients with visceral obesity: a pilot study. Diabetes Ther. 8 (4), 851–861. doi:10.1007/s13300-017-0279-y
Furman D., Campisi J., Verdin E., Carrera-Bastos P., Targ S., Franceschi C., et al. (2019). Chronic inflammation in the etiology of disease across the life span. Nat. Med. 25 (12), 1822–1832. doi:10.1038/s41591-019-0675-0
Gaborit B., Julla J. B., Fournel J., Ancel P., Soghomonian A., Deprade C., et al. (2024). Fully automated epicardial adipose tissue volume quantification with deep learning and relationship with CAC score and micro/macrovascular complications in people living with type 2 diabetes: the multicenter EPIDIAB study. Cardiovasc Diabetol. 23 (1), 328. doi:10.1186/s12933-024-02411-y
Guglielmo M., Penso M., Carerj M. L., Giacari C. M., Volpe A., Fusini L., et al. (2024). DEep LearnIng-based QuaNtification of epicardial adipose tissue predicts MACE in patients undergoing stress CMR. Atherosclerosis 397, 117549. doi:10.1016/j.atherosclerosis.2024.117549
Han L., Like L., Wang M., Zhou M., Xu Z., Yan F., et al. (2024). Investigating the peri-saphenous vein graft fat attenuation index on computed tomography angiography: relationship with progression of venous coronary artery bypass graft disease and temporal trends. BMC Cardiovasc Disord. 24 (1), 597. doi:10.1186/s12872-024-04257-4
He W., Lu Y., Yin J., He F., Zhang Y., Qiao G., et al. (2024). Coronary computed tomography angiography-derived fat attenuation index predict future percutaneous coronary intervention. Br. J. Radiol. 97 (1163), 1782–1790. doi:10.1093/bjr/tqae135
Hirata Y., Kurobe H., Akaike M., Chikugo F., Hori T., Bando Y., et al. (2011a). Enhanced inflammation in epicardial fat in patients with coronary artery disease. Int. Heart J. 52 (3), 139–142. doi:10.1536/ihj.52.139
Hirata Y., Tabata M., Kurobe H., Motoki T., Akaike M., Nishio C., et al. (2011b). Coronary atherosclerosis is associated with macrophage polarization in epicardial adipose tissue. J. Am. Coll. Cardiol. 58 (3), 248–255. doi:10.1016/j.jacc.2011.01.048
Hirata Y., Yamada H., Kusunose K., Iwase T., Nishio S., Hayashi S., et al. (2015). Clinical utility of measuring epicardial adipose tissue thickness with echocardiography using a high-frequency linear probe in patients with coronary artery disease. J. Am. Soc. Echocardiogr. 28 (10), 1240–1246. doi:10.1016/j.echo.2015.07.006
Huang S., Yu X., Yang B., Xu T., Gu H., Wang X. (2024). Predictive value of pericoronary fat attenuation index for graft occlusion after coronary artery bypass grafting. Jpn. J. Radiol. doi:10.1007/s11604-024-01709-x
Iacobellis G., Mohseni M., Bianco S. D., Banga P. K. (2017). Liraglutide causes large and rapid epicardial fat reduction. Obes. (Silver Spring) 25 (2), 311–316. doi:10.1002/oby.21718
Ichikawa K., Miyoshi T., Ohno Y., Osawa K., Nakashima M., Nishihara T., et al. (2023). Association between high pericoronary adipose tissue computed tomography attenuation and impaired flow-mediated dilation of the brachial artery. J. Atheroscler. Thromb. 30 (4), 364–376. doi:10.5551/jat.63580
Ichikawa K., Miyoshi T., Osawa K., Nakashima M., Miki T., Nishihara T., et al. (2022). High pericoronary adipose tissue attenuation on computed tomography angiography predicts cardiovascular events in patients with type 2 diabetes mellitus: post-hoc analysis from a prospective cohort study. Cardiovasc Diabetol. 21 (1), 44. doi:10.1186/s12933-022-01478-9
Ito H., Wakatsuki T., Yamaguchi K., Fukuda D., Kawabata Y., Matsuura T., et al. (2020). Atherosclerotic coronary plaque is associated with adventitial vasa vasorum and local inflammation in adjacent epicardial adipose tissue in fresh cadavers. Circ. J. 84 (5), 769–775. doi:10.1253/circj.CJ-19-0914
Karagiannis T., Tsapas A., Bekiari E., Toulis K. A., Nauck M. A. (2024). A methodological framework for meta-analysis and clinical interpretation of subgroup data: the case of major adverse cardiovascular events with GLP-1 receptor agonists and SGLT2 inhibitors in type 2 diabetes. Diabetes Care 47 (2), 184–192. doi:10.2337/dc23-0925
Kato S., Utsunomiya D., Horita N., Hoshino M., Kakuta T. (2022). Prognostic significance of the perivascular fat attenuation index derived by coronary computed tomography: a meta-analysis. Hell. J. Cardiol. 67, 73–75. doi:10.1016/j.hjc.2022.07.004
Kawabata Y., Wakatsuki T., Yamaguchi K., Fukuda D., Ito H., Matsuura T., et al. (2023). Association of microluminal structures assessed by optical coherence tomography with local inflammation in adjacent epicardial adipose tissue and coronary plaque characteristics in fresh cadavers. Circ. J. 87 (2), 329–335. doi:10.1253/circj.CJ-22-0299
Kim H. W., Shi H., Winkler M. A., Lee R., Weintraub N. L. (2020). Perivascular adipose tissue and vascular perturbation/atherosclerosis. Arterioscler. Thromb. Vasc. Biol. 40 (11), 2569–2576. doi:10.1161/atvbaha.120.312470
Kinoshita D., Suzuki K., Yuki H., Niida T., Fujimoto D., Minami Y., et al. (2024). Sex-specific association between perivascular inflammation and plaque vulnerability. Circ. Cardiovasc Imaging 17 (2), e016178. doi:10.1161/circimaging.123.016178
Kramer C. M., Borlaug B. A., Zile M. M., Ruff D., DiMaria J. M., Menon V., et al. (2024). Tirzepatide reduces LV mass and paracardiac adipose tissue in obesity-related heart failure: SUMMIT CMR substudy. J. Am. Coll. Cardiol. doi:10.1016/j.jacc.2024.11.001
Kuneman J. H., van Rosendael S. E., van der Bijl P., van Rosendael A. R., Kitslaar P. H., Reiber J. H. C., et al. (2023). Pericoronary adipose tissue attenuation in patients with acute coronary syndrome versus stable coronary artery disease. Circ. Cardiovasc Imaging 16 (2), e014672. doi:10.1161/circimaging.122.014672
Li Y., Yao W., Wang T., Yang Q., Song K., Zhang F., et al. (2024). Association of semaglutide treatment with coronary artery inflammation in type 2 diabetes mellitus patients: a retrospective study based on pericoronary adipose tissue attenuation. Cardiovasc Diabetol. 23 (1), 348. doi:10.1186/s12933-024-02445-2
Liu M., Zhen Y., Shang J., Dang Y., Zhang Q., Ni W., et al. (2024a). The predictive value of lesion-specific pericoronary fat attenuation index for major adverse cardiovascular events in patients with type 2 diabetes. Cardiovasc Diabetol. 23 (1), 191. doi:10.1186/s12933-024-02272-5
Liu X., Yuan M., Zhao D., Zeng Q., Li W., Li T., et al. (2024b). Single-nucleus transcriptomic atlas of human pericoronary epicardial adipose tissue in normal and pathological conditions. Arterioscler. Thromb. Vasc. Biol. 44 (7), 1628–1645. doi:10.1161/atvbaha.124.320923
Liu Y., Dai L., Dong Y., Ma C., Cheng P., Jiang C., et al. (2024c). Coronary inflammation based on pericoronary adipose tissue attenuation in type 2 diabetic mellitus: effect of diabetes management. Cardiovasc Diabetol. 23 (1), 108. doi:10.1186/s12933-024-02199-x
Liu Y., Sun Y., Lin X., Zhang D., Hu C., Liu J., et al. (2022). Perivascular adipose-derived exosomes reduce macrophage foam cell formation through miR-382-5p and the BMP4-PPARγ-ABCA1/ABCG1 pathways. Vasc. Pharmacol. 143, 106968. doi:10.1016/j.vph.2022.106968
Löhn M., Dubrovska G., Lauterbach B., Luft F. C., Gollasch M., Sharma A. M. (2002). Periadventitial fat releases a vascular relaxing factor. Faseb J. 16 (9), 1057–1063. doi:10.1096/fj.02-0024com
Mahabadi A. A., Berg M. H., Lehmann N., Kälsch H., Bauer M., Kara K., et al. (2013). Association of epicardial fat with cardiovascular risk factors and incident myocardial infarction in the general population: the Heinz Nixdorf Recall Study. J. Am. Coll. Cardiol. 61 (13), 1388–1395. doi:10.1016/j.jacc.2012.11.062
Marketou M., Kontaraki J., Kalogerakos P., Plevritaki A., Chlouverakis G., Kassotakis S., et al. (2023). Differences in MicroRNA expression in pericoronary adipose tissue in coronary artery disease compared to severe valve dysfunction. Angiology 74 (1), 22–30. doi:10.1177/00033197221121617
Matsuzawa Y., Funahashi T., Kihara S., Shimomura I. (2004). Adiponectin and metabolic syndrome. Arterioscler. Thromb. Vasc. Biol. 24 (1), 29–33. doi:10.1161/01.Atv.0000099786.99623.Ef
Mazurek T., Zhang L., Zalewski A., Mannion J. D., Diehl J. T., Arafat H., et al. (2003). Human epicardial adipose tissue is a source of inflammatory mediators. Circulation 108 (20), 2460–2466. doi:10.1161/01.Cir.0000099542.57313.C5
Moreno P. R., Purushothaman K. R., Fuster V., Echeverri D., Truszczynska H., Sharma S. K., et al. (2004). Plaque neovascularization is increased in ruptured atherosclerotic lesions of human aorta: implications for plaque vulnerability. Circulation 110 (14), 2032–2038. doi:10.1161/01.Cir.0000143233.87854.23
Myasoedova V. A., Parisi V., Moschetta D., Valerio V., Conte M., Massaiu I., et al. (2023). Efficacy of cardiometabolic drugs in reduction of epicardial adipose tissue: a systematic review and meta-analysis. Cardiovasc Diabetol. 22 (1), 23. doi:10.1186/s12933-023-01738-2
Nidorf S. M., Fiolet A. T. L., Mosterd A., Eikelboom J. W., Schut A., Opstal T. S. J., et al. (2020). Colchicine in patients with chronic coronary disease. N. Engl. J. Med. 383 (19), 1838–1847. doi:10.1056/NEJMoa2021372
Oikonomou E. K., Antoniades C. (2019). The role of adipose tissue in cardiovascular health and disease. Nat. Rev. Cardiol. 16 (2), 83–99. doi:10.1038/s41569-018-0097-6
Oikonomou E. K., Marwan M., Desai M. Y., Mancio J., Alashi A., Hutt Centeno E., et al. (2018). Non-invasive detection of coronary inflammation using computed tomography and prediction of residual cardiovascular risk (the CRISP CT study): a post-hoc analysis of prospective outcome data. Lancet 392 (10151), 929–939. doi:10.1016/s0140-6736(18)31114-0
Oikonomou E. K., Williams M. C., Kotanidis C. P., Desai M. Y., Marwan M., Antonopoulos A. S., et al. (2019). A novel machine learning-derived radiotranscriptomic signature of perivascular fat improves cardiac risk prediction using coronary CT angiography. Eur. Heart J. 40 (43), 3529–3543. doi:10.1093/eurheartj/ehz592
Phillippi J. A. (2022). On vasa vasorum: a history of advances in understanding the vessels of vessels. Sci. Adv. 8 (16), eabl6364. doi:10.1126/sciadv.abl6364
Qin B., Li Z., Zhou H., Liu Y., Wu H., Wang Z. (2022). The predictive value of the perivascular adipose tissue CT fat attenuation index for coronary in-stent restenosis. Front. Cardiovasc Med. 9, 822308. doi:10.3389/fcvm.2022.822308
Rämö J. T., Kany S., Hou C. R., Friedman S. F., Roselli C., Nauffal V., et al. (2024). Cardiovascular significance and genetics of epicardial and pericardial adiposity. JAMA Cardiol. 9 (5), 418–427. doi:10.1001/jamacardio.2024.0080
Ridker P. M., Everett B. M., Thuren T., MacFadyen J. G., Chang W. H., Ballantyne C., et al. (2017). Antiinflammatory therapy with canakinumab for atherosclerotic disease. N. Engl. J. Med. 377 (12), 1119–1131. doi:10.1056/NEJMoa1707914
Ross R. (1999). Atherosclerosis-an inflammatory disease. N. Engl. J. Med. 340 (2), 115–126. doi:10.1056/nejm199901143400207
Sacks H. S., Fain J. N. (2007). Human epicardial adipose tissue: a review. Am. Heart J. 153 (6), 907–917. doi:10.1016/j.ahj.2007.03.019
Sagris M., Antonopoulos A. S., Simantiris S., Oikonomou E., Siasos G., Tsioufis K., et al. (2022). Pericoronary fat attenuation index-a new imaging biomarker and its diagnostic and prognostic utility: a systematic review and meta-analysis. Eur. Heart J. Cardiovasc Imaging 23 (12), e526–e536. doi:10.1093/ehjci/jeac174
Sato T., Aizawa Y., Yuasa S., Kishi S., Fuse K., Fujita S., et al. (2018). The effect of dapagliflozin treatment on epicardial adipose tissue volume. Cardiovasc Diabetol. 17 (1), 6. doi:10.1186/s12933-017-0658-8
Shimabukuro M., Hirata Y., Tabata M., Dagvasumberel M., Sato H., Kurobe H., et al. (2013). Epicardial adipose tissue volume and adipocytokine imbalance are strongly linked to human coronary atherosclerosis. Arterioscler. Thromb. Vasc. Biol. 33 (5), 1077–1084. doi:10.1161/atvbaha.112.300829
Sluimer J. C., Kolodgie F. D., Bijnens A. P., Maxfield K., Pacheco E., Kutys B., et al. (2009). Thin-walled microvessels in human coronary atherosclerotic plaques show incomplete endothelial junctions relevance of compromised structural integrity for intraplaque microvascular leakage. J. Am. Coll. Cardiol. 53 (17), 1517–1527. doi:10.1016/j.jacc.2008.12.056
Soltis E. E., Cassis L. A. (1991). Influence of perivascular adipose tissue on rat aortic smooth muscle responsiveness. Clin. Exp. Hypertens. A 13 (2), 277–296. doi:10.3109/10641969109042063
Szasz T., Bomfim G. F., Webb R. C. (2013). The influence of perivascular adipose tissue on vascular homeostasis. Vasc. Health Risk Manag. 9, 105–116. doi:10.2147/vhrm.S33760
Takano M., Kondo H., Harada T., Takahashi M., Ishii Y., Yamasaki H., et al. (2023). Empagliflozin suppresses the differentiation/maturation of human epicardial preadipocytes and improves paracrine secretome profile. JACC Basic Transl. Sci. 8 (9), 1081–1097. doi:10.1016/j.jacbts.2023.05.007
Tanaka K., Fukuda D., Sata M. (2020). Roles of epicardial adipose tissue in the pathogenesis of coronary atherosclerosis - an update on recent findings. Circ. J. 85 (1), 2–8. doi:10.1253/circj.CJ-20-0935
Tanaka K., Sata M. (2018). Roles of perivascular adipose tissue in the pathogenesis of atherosclerosis. Front. Physiol. 9, 3. doi:10.3389/fphys.2018.00003
Theofilis P., Sagris M., Antonopoulos A. S., Oikonomou E., Tsioufis K., Tousoulis D. (2022). Non-Invasive modalities in the assessment of vulnerable coronary atherosclerotic plaques. Tomography 8 (4), 1742–1758. doi:10.3390/tomography8040147
Turaihi A. H., Serné E. H., Molthoff C. F. M., Koning J. J., Knol J., Niessen H. W., et al. (2020). Perivascular adipose tissue controls insulin-stimulated perfusion, mitochondrial protein expression, and glucose uptake in muscle through adipomuscular arterioles. Diabetes 69 (4), 603–613. doi:10.2337/db18-1066
Vacca M., Di Eusanio M., Cariello M., Graziano G., D'Amore S., Petridis F. D., et al. (2016). Integrative miRNA and whole-genome analyses of epicardial adipose tissue in patients with coronary atherosclerosis. Cardiovasc Res. 109 (2), 228–239. doi:10.1093/cvr/cvv266
van Eyk H. J., Paiman E. H. M., Bizino M. B., de Heer P., Geelhoed-Duijvestijn P. H., Kharagjitsingh A. V., et al. (2019). A double-blind, placebo-controlled, randomised trial to assess the effect of liraglutide on ectopic fat accumulation in South Asian type 2 diabetes patients. Cardiovasc Diabetol. 18 (1), 87. doi:10.1186/s12933-019-0890-5
van Rosendael S. E., Kamperidis V., Maaniitty T., de Graaf M. A., Saraste A., McKay-Goodall G. E., et al. (2024). Pericoronary adipose tissue for predicting long-term outcomes. Eur. Heart J. Cardiovasc Imaging 25 (10), 1351–1359. doi:10.1093/ehjci/jeae197
Wang J., Zhang H., Wang Z., Liu W., Cao D., Tong Q. (2024). Evaluating the role of pericoronary adipose tissue on coronary artery disease: insights from CCTA on risk assessment, vascular stenosis, and plaque characteristics. Front. Cardiovasc Med. 11, 1451807. doi:10.3389/fcvm.2024.1451807
Wang M., Pan W., Xu Y., Zhang J., Wan J., Jiang H. (2022). Microglia-mediated neuroinflammation: a potential target for the treatment of cardiovascular diseases. J. Inflamm. Res. 15, 3083–3094. doi:10.2147/jir.S350109
Yagi S., Hirata Y., Ise T., Kusunose K., Yamada H., Fukuda D., et al. (2017). Canagliflozin reduces epicardial fat in patients with type 2 diabetes mellitus. Diabetol. Metab. Syndr. 9, 78. doi:10.1186/s13098-017-0275-4
Yamada H., Sata M. (2015). Role of pericardial fat: the good, the bad and the ugly. J. Cardiol. 65 (1), 2–4. doi:10.1016/j.jjcc.2014.07.004
Yang W., Ding X., Yu Y., Lan Z., Yu L., Yuan J., et al. (2024). Long-term prognostic value of CT-based high-risk coronary lesion attributes and radiomic features of pericoronary adipose tissue in diabetic patients. Clin. Radiol. 79 (12), 931–940. doi:10.1016/j.crad.2024.08.018
Zhan W., Luo Y., Luo H., Zhou Z., Yin N., Li Y., et al. (2024). Predicting major adverse cardiovascular events in angina patients using radiomic features of pericoronary adipose tissue based on CCTA. Front. Cardiovasc Med. 11, 1462451. doi:10.3389/fcvm.2024.1462451
Zhao E., Giamberardino S. N., Pagidipati N. J., Voora D., Ginsburg G. S., Hoffmann U., et al. (2023). Branched-chain amino acids in computed tomography-defined adipose depots and coronary artery disease: a promise trial biomarker substudy. J. Am. Heart Assoc. 12 (11), e028410. doi:10.1161/jaha.122.028410
Zhao J., Cheng W., Dai Y., Li Y., Feng Y., Tan Y., et al. (2024). Excessive accumulation of epicardial adipose tissue promotes microvascular obstruction formation after myocardial ischemia/reperfusion through modulating macrophages polarization. Cardiovasc Diabetol. 23 (1), 236. doi:10.1186/s12933-024-02342-8
Keywords: perivascular adipose tissue (PVAT), epicardial adipose tissue (EAT), vasa vasorum (VV), chronic inflammation, atherosclerosis, cardiovascular disease (CVD)
Citation: Hara T and Sata M (2025) Roles of perivascular adipose tissue in the pathogenesis of atherosclerosis ― an update on recent findings. Front. Physiol. 15:1522471. doi: 10.3389/fphys.2024.1522471
Received: 04 November 2024; Accepted: 16 December 2024;
Published: 06 January 2025.
Edited by:
Maria Andreia Delbin, State University of Campinas, BrazilReviewed by:
Longhua Liu, Shanghai University of Sport, ChinaSonia Eiras, Health Research Institute of Santiago de Compostela (IDIS), Spain
Copyright © 2025 Hara and Sata. This is an open-access article distributed under the terms of the Creative Commons Attribution License (CC BY). The use, distribution or reproduction in other forums is permitted, provided the original author(s) and the copyright owner(s) are credited and that the original publication in this journal is cited, in accordance with accepted academic practice. No use, distribution or reproduction is permitted which does not comply with these terms.
*Correspondence: Tomoya Hara, aGFyYS50b21veWEuMkB0b2t1c2hpbWEtdS5hYy5qcA==