- 1Inserm U1093, CAPS Université de Bourgogne, Faculté des Sciences du Sport, Dijon, France
- 2Institute of Sport Sciences, Faculty of Biology and Medicine, University of Lausanne, Lausanne, Switzerland
- 3Department of Biomedical Sciences, Faculty of Biology and Medicine, University of Lausanne, Lausanne, Switzerland
- 4Department of Sports Medicine, Swiss Olympic Medical Center, Lausanne University Hospital, Lausanne, Switzerland
Purpose: This study examined the physiological effects of 12 weeks of detraining and retraining in a highly trained master triathlete (age 53.8 years).
Methods: Variables associated with swimming, cycling, and running performance, including
Results: Detraining resulted in a 9.1% and 10.9% decrease in relative
Conclusion: After 12 weeks of detraining, a lifelong master triathlete can regain his cardiorespiratory fitness (i.e.,
Introduction
The time needed to return to a fitness level close to the initial state after a prolonged training cessation (e.g., more than a month) is an important issue for many athletes. Detraining is characterized by marked alterations in the cardiorespiratory system (e.g., reduction in maximal oxygen uptake -
Aging is associated with progressive reductions in cardiovascular and neuromuscular function, contributing to the decline in the physiological determinants of endurance performance with advancing age. For example, maximum cardiorespiratory fitness, measured by maximal oxygen uptake, decreases by 0.5%–1% per year starting at age 30, primarily due to lower cardiac output resulting from a reduction in maximum heart rate (0.7 beats per minute per year) and a decrease in muscle mass (Trappe et al., 1996). Running economy at a fixed running speed has also been reported to decrease with age in some studies (e.g., dos Anjos Souza et al., 2023), though not all (e.g., Quinn et al., 2011), with this decline partially attributed to reductions in tendon stiffness (Karamanidis and Arampatzis, 2006), which, in turn, decreases the mechanical efficiency of the muscle (Bohm et al., 2019). Finally, the metabolic rate at which the lactate threshold occurs has been reported to decrease (Wiswell et al., 2000) or remain unchanged with age (Allen et al., 1985). While master athletes experience notable declines in cardiovascular function and endurance capacity after periods of detraining, they may maintain certain physiological adaptations acquired through lifelong training, potentially mitigating the effects of detraining (Barbieri et al., 2024).
Endurance exercise training induces, among other adaptations, increases in stroke volume and muscle oxidative capacity, resulting in an increase in
Conducting studies to examine the effects of detraining and retraining in competitive, well-trained athletes is difficult, as these athletes are often reluctant to voluntarily stop their training for a long period (e.g., >4 weeks). Consequently, even case reports represent valuable information on changes in physical fitness after periods of detraining and retraining.
In a previous case report, we demonstrated that a 12-week detraining program could benefit a well-trained master athlete by enhancing their muscle metabolic phenotype and aerobic capacity following a retraining protocol (Zanou et al., 2024). At the muscle level, detraining was associated with reduced levels of mitochondrial biogenesis and oxidative phosphorylation (OXPHOS) proteins. Conversely, it led to an increase in the levels of the ryanodine receptor type 1 (RyR1) protein, myosin heavy chain fast-twitch (fast MHC), and Sarco/Endoplasmic reticulum Ca2+ ATPase type 1 (SERCA) protein. In contrast, retraining elevated not only mitochondrial proteins but also glycolytic proteins beyond baseline levels, likely improving skeletal muscle metabolism. In this companion article, we describe the effects of a 12-week detraining period followed by a retraining period on cardiorespiratory fitness, muscular strength, and body composition in the same highly trained male master triathlete.
Methods
Subject
At the first evaluation date, the subject was a 53.8-year-old French Caucasian. He had been racing triathlons since the age of 20. At the peak of his amateur career, he twice finished in the top 150 at the Hawaii Ironman triathlon championship. Since entering the master category (>40 years old), he has regularly placed in the top 5 of his categories in Ironman 70.3 events. For the past 20 years, he has maintained a regular training program, with no break of more than 2 weeks in a row each year. His annual training volume has remained relatively constant at around 500 h per year (120 h swimming, 270 h cycling, and 110 h running). In the summer of 2022, he voluntarily decided to take a 3-month break after his last scheduled competition on 5 September 2022 (ÖTILLÖ, Swimrun World Championship in Sweden, consisting of a 10 km open-water swim and 65 km trail run, where he placed 42nd in men’s team category in 10 h 01 min). In the 3 months leading up to his last competition, he trained an average of 10.8 h per week, consisting of 32% swimming, 38% cycling, and 30% running.
Design
Baseline data were collected in the 2 weeks leading up to the cessation of training. The day after his last competition, the athlete stopped all structured training and only engaged in minimal physical activity for 12 weeks. He walked twice a week for 30 min at a slow pace and completed 15 min of core training twice a week (e.g., forearm plank, side bend, hip dip). Re-testing was carried out during the week following the 12-week detraining period. The athlete then began a structured retraining program for 12 weeks, gradually progressing from approximately 50% of his usual training volume to 100% over the following 6 weeks. By the seventh week of retraining, he had reached his previous regular training regimen (10–12 h per week). During the retraining period, his training volume was divided as follows: 28% swimming, 47% cycling, and 25% running. The athlete showed no abnormal symptoms or injuries when he resumed training and did not change his diet.
Measurements
The master triathlete carried out swimming, cycling, and running tests.
Swimming test
The participant performed a 400 m freestyle test in a 50 m length pool.
Cycling test
The participant performed an incremental exercise test on an electrically braked cycle ergometer (Lode Excalibur, Groningen, Netherlands) equipped with automatic pedals. This test was used to determine gross cycling efficiency (CE),
Running test
The subject performed a continuous incremental exercise on a Pulsar 3p® treadmill (h/p/cosmos sports and medical gmbh, Traunstein, Germany). This test allowed us to determine running economy (RE), VO2max,
For the calculation of CE and RE, the power output and running velocity were based on the levels the athlete could maintain throughout the 180 km cycling and 42 km running sections of an Ironman triathlon. During the stages where CE and RE were calculated, the RER values were below 1.0, ranging between 0.81 and 0.94.
Cardiorespiratory responses
Heart rate and
Knee extensor muscle strength
The participant performed maximal isometric and isokinetic voluntary knee extensor contractions with his right leg in concentric mode at 60°.s−1, 120°.s−1, and 180°.s−1. He was seated on an isokinetic dynamometer (System pro 4, Biodex Medical System, New York) with a 90° hip flexion and the knee joint axis in line with the rotation axis of the dynamometer. At least two maximal voluntary contractions of each contraction mode and angular velocity were performed (more if the difference between the peak torque of two consecutive contractions was greater than 5%). The trial with the greatest peak torque was analyzed. The participant also performed a Thorstensson Test (Thorstensson and Karlsson, 1976) to assess muscular endurance. Briefly, 50 maximal isokinetic voluntary knee extensor contractions were repeated at 180°.s−1, and a fatigue index over 50 contractions was calculated as: (last 5-first 5)/first 5 × 100. The participant was instructed to give an all-out effort during each concentric action and to relax his leg during flexion back to the starting position.
Finally, three squat jumps (SJ) and three countermovement jumps (CMJ), each separated by 1-min recovery, were performed. The trial with the greatest height was analyzed. The participant had to keep his hands on his hips, starting from a static standing position, leg straight for CMJ and leg fold at 90° for SJ. The subject was instructed to jump as high as possible. Jump performance was assessed using the My Jump app on an iPhone (Gençoğlu et al., 2023).
Body composition
Body composition was measured via dual-energy x-ray absorptiometry (Lunar iDXA ME + 210430). There were no specific instructions regarding hydration and diet prior to the DXA or performance tests, but participants were asked to maintain the same dietary routine before each testing session.
Results
Figure 1 shows that absolute
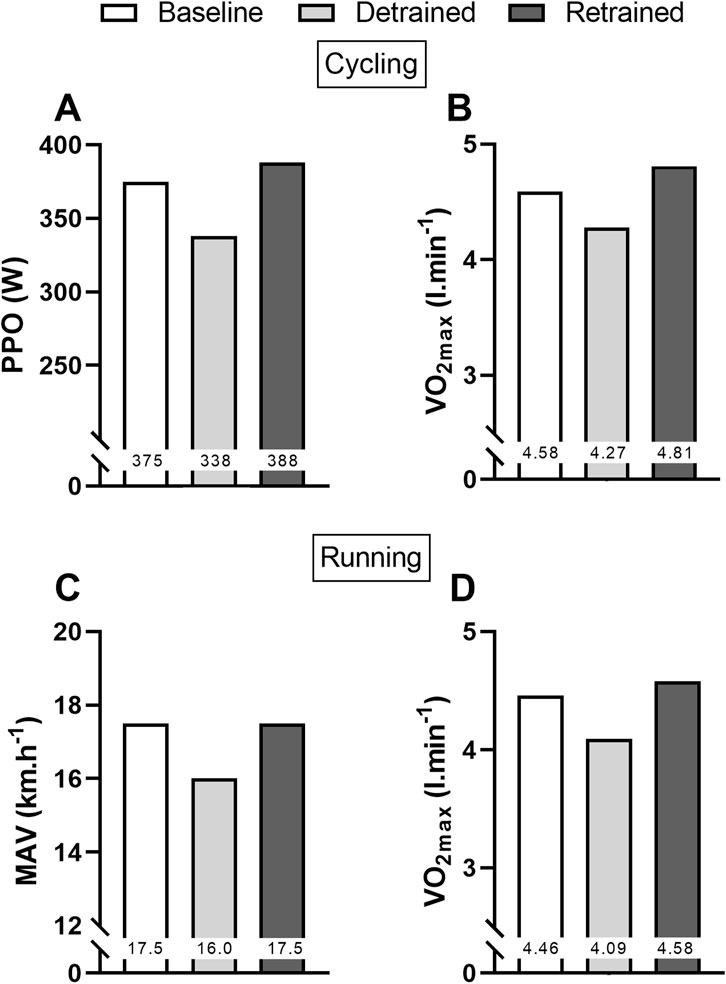
Figure 1. Values of peak power output (PPO) (A) and absolute maximal oxygen uptake (VO2max) (B) for cycling, and maximal aerobic velocity (MAV) (C) and absolute VO2max (D) for running at baseline, after 12 weeks of training cessation (detrained), and after 12 weeks of retraining (retrained).
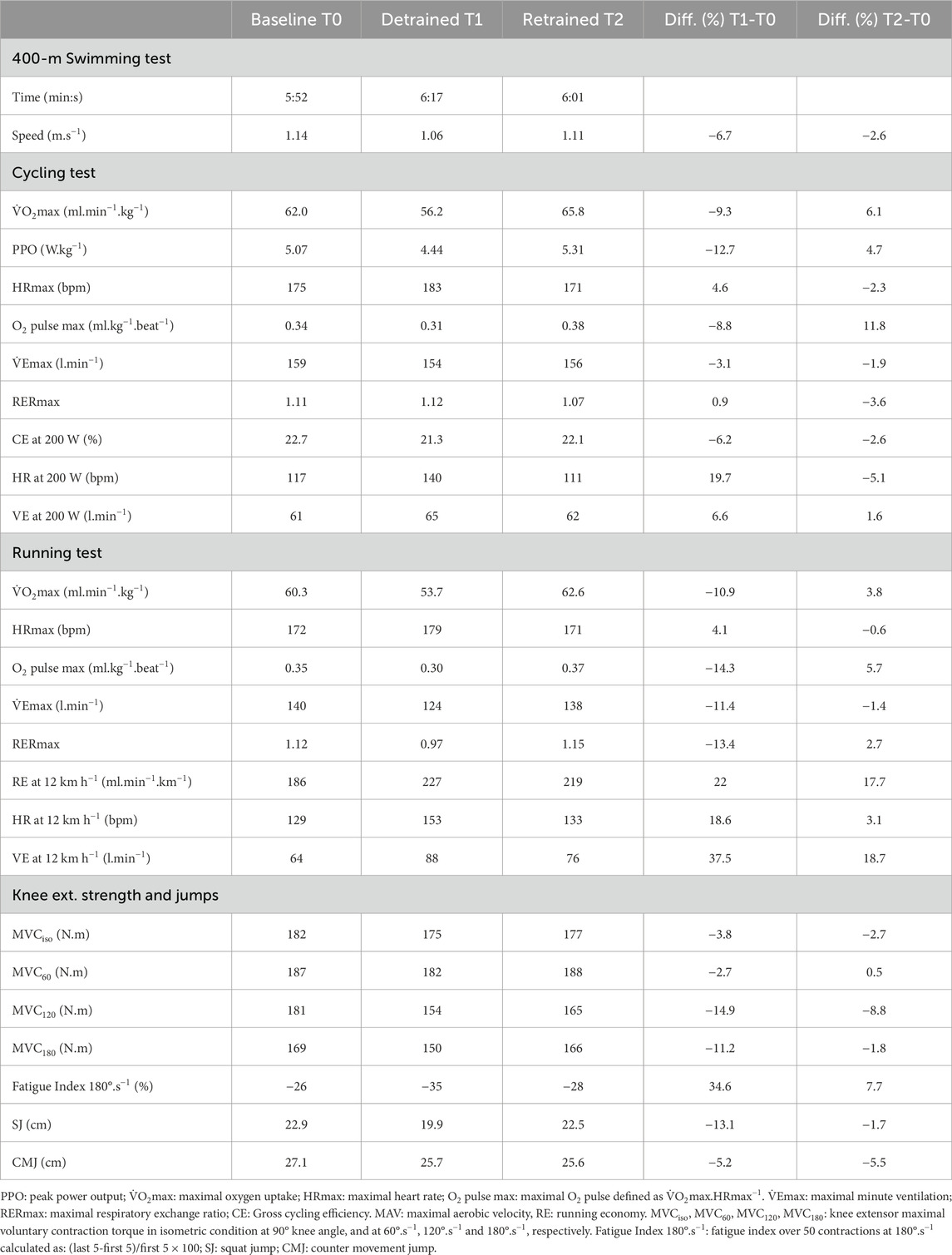
Table 1. Swimming performance, physiological variables during the cycling and running incremental tests, and knee extensor muscle strength and jump performances at Baseline, after 3 months of detraining (Detrained), and after 3 months of retraining (Retrained). Body mass of the participant at the testing time: Baseline, 74.0 kg; Detrained, 76.1 kg; Retrained, 73.1 kg.
Analysis at submaximal exercise intensities revealed that after 12 weeks of detraining, CE (at an intensity corresponding to 53% of baseline PPO) decreased by 6.2% and remained 2.6% below baseline after retraining (Table 1). Similarly, running economy (at an intensity corresponding to 68% of baseline MAV) was 22% higher after detraining and remained 17% higher after retraining compared to baseline. Heart rate and ventilation at these submaximal intensities were higher after detraining than baseline values for cycling and running. After retraining, these variables returned to baseline values for cycling but not for running.
Table 1 showed that, after detraining, maximal strength capacity of the knee extensor muscles decreased by an average of 8.2%, ranging from 2.7% to 14.9%, depending on the contraction mode. After retraining, maximal strength capacity remained slightly below baseline values (−3.2% on average). The fatigue index over 50 repeated dynamic contractions increased by 34.6% after detraining and remained higher by 7.7% after retraining compared to baseline values. After detraining, jumping performances decreased by an average of 9.2% (−5.2% for the CMJ and −13.1% for the SJ), respectively. After retraining, jumping performances remained slightly below baseline values (−3.6% on average).
Body fat percentage increased from 10.1% to 13.3% during detraining (Table 2). The participant gained 2.5 kg of fat and lost 2.2 kg of fat-free mass. The loss of fat-free mass was more pronounced in the trunk (−5.7%) than in the arms (−1%) and legs (−1.5%). After 12 weeks of retraining, the participant was lighter than at baseline, and his percentage of body fat had dropped to 8.7%. His body fat mass was 1.6 kg lower than the baseline value, and his free-fat mass was 1 kg lower. Bone mineral content remained unchanged during the detraining and retraining periods (Table 2).
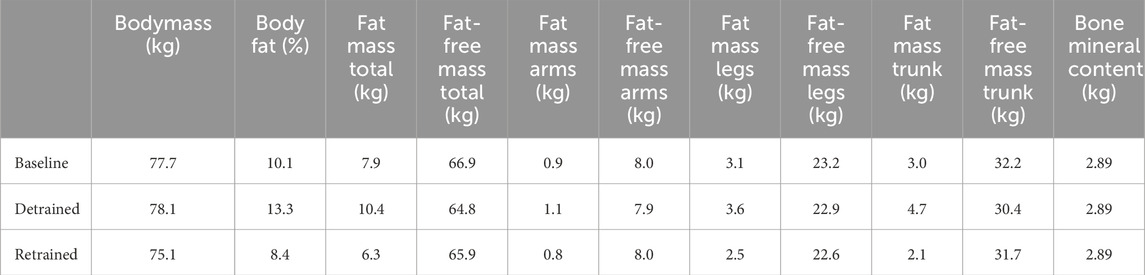
Table 2. Body composition calculated by DEXA at Baseline, after 12 weeks of detraining (Detrained) and after 12 weeks of retraining (Retrained).
Discussion
This study reports on changes in cardiorespiratory fitness, body composition and knee extensor maximal strength capacity of a highly trained 54-year-old master triathlete following 12 weeks of detraining and retraining. At baseline, his
The decrease in
When the participant was 40, his
After detraining, RE assessed at 12 km h−1 was much more altered than
After detraining, the decline in maximum strength capacity was more pronounced for the concentric contractions at high contraction speeds (11%–15% at 120°.s−1 and 180°.s−1) compared with those at lower speeds (60°.s−1) or under isometric conditions (3%–4%). The ability to maintain a level of muscle torque over 50 dynamic contractions (estimated by the fatigue index) was much more diminished (35%) than the maximum strength capacity, suggesting that the subject was more fatigable after detraining. These results are consistent with the increase in fast MHC observed in the participant.6 The decline in strength capacity could be explained by the reduction in leg fat-free mass, which was reduced by 1.5% during the detraining period. The leg fat-free mass did not return to baseline after retraining, nor did strength capabilities completely return to baseline. It should be noted that total mass and body fat percentage were lower after retraining than at baseline, which contributed to the increased relative
One of the limitations of this study is that the lactate threshold was not assessed. Lactate threshold has been identified as one of the main determinants of endurance, in addition to
Practical applications
The results of this study suggest that after a detraining period of up to 12 weeks, well-trained endurance master athletes can regain their cardiorespiratory fitness (i.e.,
Conclusion
This case report illustrates the changes in cardiorespiratory fitness following 12 weeks of detraining and retraining in a lifelong endurance athlete following his competitive season. After a retraining period of the same duration as the detraining one, the master athlete reached and even exceeded his baseline level of cardiorespiratory fitness, but his running economy and lean mass remained slightly depressed.
Data availability statement
The raw data supporting the conclusions of this article will be made available by the authors, without undue reservation.
Ethics statement
The studies involving humans were approved by the Committee on the Protection of Human Subjects (CER-VD 2022-01614) of Lausanne. The studies were conducted in accordance with the local legislation and institutional requirements. The participants provided their written informed consent to participate in this study. Written informed consent was obtained from the individual(s) for the publication of any potentially identifiable images or data included in this article.
Author contributions
RL: Writing–original draft, Writing–review and editing. AM: Writing–original draft, Writing–review and editing. HA: Writing–original draft, Writing–review and editing. NZ: Writing–original draft, Writing–review and editing. VG: Writing–original draft, Writing–review and editing. NP: Writing–original draft, Writing–review and editing.
Funding
The author(s) declare that no financial support was received for the research, authorship, and/or publication of this article.
Conflict of interest
The authors declare that the research was conducted in the absence of any commercial or financial relationships that could be construed as a potential conflict of interest.
Generative AI statement
The author(s) declare that no Generative AI was used in the creation of this manuscript.
Publisher’s note
All claims expressed in this article are solely those of the authors and do not necessarily represent those of their affiliated organizations, or those of the publisher, the editors and the reviewers. Any product that may be evaluated in this article, or claim that may be made by its manufacturer, is not guaranteed or endorsed by the publisher.
References
ACSM’s Guidelines for Exercise Testing and Prescription (2021). Editors G. Liguori, Y. Feito, C. Fountaine, and B. A. Roy 11th ed. (Philadelphia (PA: Lippincott Williams & Wilkins).
Allen W. K., Seals D. R., Hurley B. F., Ehsani A. A., Hagberg J. M. (1985). Lactate threshold and distance-running performance in young and older endurance athletes. J. Appl. Physiol. 58 (4), 1281–1284. doi:10.1152/jappl.1985.58.4.1281
Barbieri A., Fuk A., Gallo G., Gotti D., Meloni A., La Torre A., et al. (2024). Cardiorespiratory and metabolic consequences of detraining in endurance athletes. Front. Physiol. 14, 1334766. doi:10.3389/fphys.2023.1334766
Bohm S., Mersmann F., Santuz A., Arampatzis A. (2019). The force-length-velocity potential of the human soleus muscle is related to the energetic cost of running. Proc. Biol. Sci. 286 (1917), 20192560. doi:10.1098/rspb.2019.2560
Burtscher J., Strasser B., Burtscher M., Millet G. P. (2022). The impact of training on the loss of cardiorespiratory fitness in aging masters endurance athletes. Int. J. Environ. Res. Public Health 19, 11050. doi:10.3390/ijerph191711050
Coyle E. F., Martin W. H. 3rd, Sinacore D. R., Joyner M. J., Hagberg J. M., Holloszy J. O. (1984). Time course of loss of adaptations after stopping prolonged intense endurance training. J. Appl. Physiol. Respir. Environ. Exerc Physiol. 57, 1857–1864. doi:10.1152/jappl.1984.57.6.1857
Coyle E. F., Sidossis L. S., Horowitz J. F., Beltz J. D. (1992). Cycling efficiency is related to the percentage of type I muscle fibers. Med. Sci. Sports Exerc 24 (7), 782–788. doi:10.1249/00005768-199207000-00008
di Prampero P. E. (1986). The energy cost of human locomotion on land and in water. Int. J. Sports Med. 7 (2), 55–72. doi:10.1055/s-2008-1025736
dos Anjos Souza V. R., Seffrin A., da Cunha R. A., Vivan L., de Lira C. A. B., Vancini R. L., et al. (2023). Running economy in long-distance runners is positively affected by running experience and negatively by aging affected by running experience and negatively by aging. Physiol. Behav. 258, 114032. doi:10.1016/j.physbeh.2022.114032
Gençoğlu C., Ulupınar S., Özbay S., Turan M., Savaş B. Ç., Asan S., et al. (2023). Validity and reliability of “My Jump app” to assess vertical jump performance: a meta-analytic review. Sci. Rep. 13, 20137. doi:10.1038/s41598-023-46935-x
Hagberg J. M., Allen W. K., Seals D. R., Hurley B. F., Ehsani A. A., Holloszy J. O. (1985). A hemodynamic comparison of young and older endurance athletes during exercise. J. Appl. Physiol. 58, 2041–2046. doi:10.1152/jappl.1985.58.6.2041
Henriksson J., Reitman J. S. (1977). Time course of changes in human skeletal muscle succinate dehydrogenase and cytochrome oxidase activities and maximal oxygen uptake with physical activity and inactivity. Acta Physiol. Scand. 99, 91–97. doi:10.1111/j.1748-1716.1977.tb10356.x
Hopkins W. G., Schabort E. J., Hawley J. A. (2001). Reliability of power in physical performance tests. Sports Med. 31 (3), 211–234. doi:10.2165/00007256-200131030-00005
Jaén-Carrillo D., Cartón-Llorente A., Lozano-Jarque D., Rubio-Peirotén A., Roche- Seruendo L. E., García-Pinillos F. (2021). Relationship between reactive strength and leg stiffness at submaximal velocity: effects of age on distance runners. Int. J. Environ. Res. Public Health 18 (13), 6866. doi:10.3390/ijerph18136866
Jones A. M. (2006). The physiology of the World record holder for the women's marathon. Int. J. Sports Sci. & Coach. 1, 101–116. doi:10.1260/174795406777641258
Joyner M. J., Coyle E. F. (2008). Endurance exercise performance: the physiology of champions. J. Physiol. 1 (586), 35–44. doi:10.1113/jphysiol.2007.143834
Karamanidis K., Arampatzis A. (2006). Mechanical and morphological properties of human quadriceps femoris and triceps surae muscle-tendon unit in relation to aging and running properties of human quadriceps femoris and triceps surae muscle-tendon unit in relation to aging and running. J. Biomech. 39 (3), 406–417. doi:10.1016/j.jbiomech.2004.12.017
Klausen K., Andersen L. B., Pelle I. (1981). Adaptive changes in work capacity, skeletal muscle capillarization and enzyme levels during training and detraining. Acta Physiol. Scand. 113, 9–16. doi:10.1111/j.1748-1716.1981.tb06854.x
Lepers R., Bontemps B., Louis J. (2020). Physiological profile of a 59-year-old male World record holder marathoner. Med. Sci. Sports Exerc 52, 623–626. doi:10.1249/MSS.0000000000002181
Lepers R., Stapley P. J. (2016). Master athletes are extending the limits of human endurance. Front. Physiol. 12 (7), 613. doi:10.3389/fphys.2016.00613
Li F., Newton R. U., Shi Y., Sutton D., Ding H. (2021). Correlation of eccentric strength, reactive strength, and leg stiffness with running economy in well-trained distance runners. J. Strength Cond. Res. 35 (6), 1491–1499. doi:10.1519/JSC.0000000000003446
Liu B., Wu J., Shi Q., Hao F., Xiao W., Yu J., et al. (2022). Running economy and lower extremity stiffness in endurance runners: a systematic review and meta-analysis. Front. Physiol. 13, 1059221. doi:10.3389/fphys.2022.1059221
Mujika I., Padilla S. (2000). Detraining: loss of training-induced physiological and performance adaptations. Part I: short term insufficient training stimulus. Sports Med. 30, 79–87. doi:10.2165/00007256-200030020-00002
Nichols J. F., Robinson D., Douglass D., Anthony J. (2000). Retraining of a competitive master athlete following traumatic injury: a case study. Med. Sci. Sports Exerc 32, 1037–1042. doi:10.1097/00005768-200006000-00001
Quinn T. J., Manley M. J., Aziz J., Padham J. L., MacKenzie A. M. (2011). Aging and factors related to running economy. J. Strength Cond. Res. 25 (11), 2971–2979. doi:10.1519/JSC.0b013e318212dd0e
Thorstensson A., Karlsson J. (1976). Fatiguability and fibre composition of human skeletal muscle. Acta Physiol. Scand. 98, 318–322. doi:10.1111/j.1748-1716.1976.tb10316.x
Trappe S. W., Costill D. L., Vukovich M. D., Jones J., andMelham T. (1996). Aging among elite distance runners: a 22-yr longitudinal study among elite distance runners: a 22-yr longitudinal study. J. Appl. Physiol. 80 (1), 285–290. doi:10.1152/jappl.1996.80.1.285
Valenzuela P. L., Maffiuletti N. A., Joyner M. J., Lucia A., Lepers R. (2020). Lifelong endurance exercise as a countermeasure against age-related VO2max decline: physiological overview and insights from masters athletes. Sports Med. 50, 703–716. doi:10.1007/s40279-019-01252-0
Wiswell R. A., Jaque S. V., Marcell T. J., Hawkins S. A., Tarpenning K. M., Constantino N., et al. (2000). Maximal aerobic power, lactate threshold, and running performance in master athletes. Med. Sci. Sports Exerc 32 (6), 1165–1170. doi:10.1097/00005768-200006000-00021
Keywords: endurance, triathlon, running, cycling, reconditioning, VO2max, peak power output, running economy
Citation: Lepers R, Mater A, Assadi H, Zanou N, Gremeaux V and Place N (2024) Effect of 12 weeks of detraining and retraining on the cardiorespiratory fitness in a competitive master athlete: a case study. Front. Physiol. 15:1508642. doi: 10.3389/fphys.2024.1508642
Received: 09 October 2024; Accepted: 08 November 2024;
Published: 22 November 2024.
Edited by:
Mathieu Gruet, Université de Toulon, FranceReviewed by:
Leonardo Alexandre Peyré-Tartaruga, University of Pavia, ItalyPedro L. Valenzuela, Research Institute Hospital 12 de Octubre, Spain
Copyright © 2024 Lepers, Mater, Assadi, Zanou, Gremeaux and Place. This is an open-access article distributed under the terms of the Creative Commons Attribution License (CC BY). The use, distribution or reproduction in other forums is permitted, provided the original author(s) and the copyright owner(s) are credited and that the original publication in this journal is cited, in accordance with accepted academic practice. No use, distribution or reproduction is permitted which does not comply with these terms.
*Correspondence: Romuald Lepers, cm9tdWFsZC5sZXBlcnNAdS1ib3VyZ29nbmUuZnI=