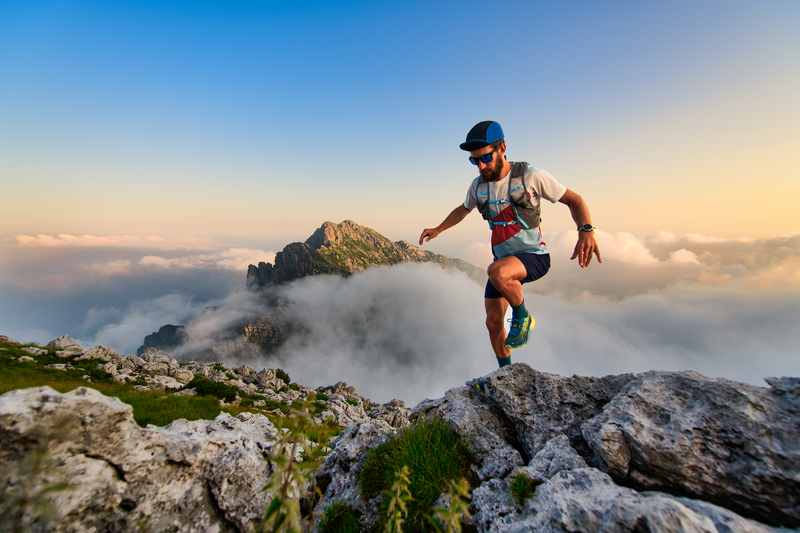
95% of researchers rate our articles as excellent or good
Learn more about the work of our research integrity team to safeguard the quality of each article we publish.
Find out more
REVIEW article
Front. Physiol. , 11 November 2024
Sec. Metabolic Physiology
Volume 15 - 2024 | https://doi.org/10.3389/fphys.2024.1491815
This article is part of the Research Topic Emerging Applications of Targeted and Non-Targeted Metabolomics to Physiology and Pathophysiology View all 8 articles
Abnormal lipid accumulation is a fundamental contributor to obesity and metabolic disorders. Lipid droplets (LDs) and mitochondria (MT) serve as organelle chaperones in lipid metabolism and energy balance. LDs play a crucial role in lipid storage and mobilization, working in conjunction with MT to regulate lipid metabolism within the liver, brown adipose tissue, and skeletal muscle, thereby maintaining metabolic homeostasis. The novelty of our review is the comprehensive description of LD and MT interaction mechanisms. We also focus on the current drugs that target this metabolism, which provide novel approaches for obesity and related metabolism disorder treatment.
Obesity has escalated to epidemic proportions worldwide and constitutes a grave threat to public health (Seidell and Halberstadt, 2015). There is also a marked increasing in these conditions among children. The primary cause of obesity is the accumulation of abnormal body fat, which significantly increases the risk of metabolic syndromes that include non-alcoholic fatty liver disease (NAFLD) (Younossi et al., 2016), high blood cholesterol, and diabetes (Kaze et al., 2021). These conditions severely affect health and substantially increase the risk of mortality (Chen et al., 2019). Research into the mechanisms underlying abnormal fat metabolism may be the new perspective for treating obesity and metabolic syndromes.
LDs and MT work cooperatively in lipid metabolism and energy maintenance. LDs-associated MT reveals the existence of various MT groups in cell structures carrying unique protein sets and exhibiting diverse capabilities in fatty acid oxidation (FAO). As mitochondrial motility is a key parameter in mitochondrial fusion, peridroplet mitochondria (PDM) segregate from cytoplasmic mitochondria (CM) due to the distinct mechanisms of mitochondrial adhesion to LDs. Aerobic exercise diminishes the interactions between hepatic LDs and MT, alongside reducing LD size, which correlates with a less severe manifestation of NAFLD (Bórquez et al., 2024). Perilipin 5 (PLIN5) is a lipid droplet-associated protein can regulate lipid metabolism through protein kinase A (PKA) phosphorylation (Gao et al., 2017; Keenan et al., 2021). In this paper, we have summarized the mechanism of LD-MT interaction in abnormal lipid deposition, which might be an influential factor in the etiology of obesity and metabolic syndrome. We also mention the current drugs that target LD-MT interaction which provide novel approaches for obesity treatment.
LDs originate in the endoplasmic reticulum (ER) and are associated with general organelles through membrane contact sites. Generally distributed in both prokaryotic and eukaryotic cells (Ibayashi et al., 2024), LDs are lipid and phospholipid storage organelles that serve as centers of lipid metabolism (Zadoorian et al., 2023). Almost all types of cells have the ability to store excess energy in the form of triacylglycerol (TAG) in LDs. LDs store neutral lipids (NLs) and proteins involved in lipid metabolism and cell membrane synthesis, serving as hubs for metabolic processes (Gross and Silver, 2014). LDs consist of a core of NL surrounded by a phospholipid monolayer, which is modified by specific proteins called LDs-associated proteins (LDAP) (Jarc and Petan, 2020), Figure1B.
Figure 1. The processes of production and metabolism of lipid droplets. (A) Lipid droplets-Mitochondria have receptor mechanisms in different tissues. (B) LDs synthesis in endoplasmic reticulum. (C) LDs produce free fatty acids (FFAs), which are finally metabolized under the action of mitochondria.
LDs formation is the process of synthesizing and assembling NL and phospholipid monolayers in the ER. This process involves the synthesis of NL within the ER, followed by nucleation, cytoplasmic outgrowth, and growth (Thiam and Ikonen, 2021). The NL stored in LDs are TAG and sterol esters (SE), with TAG being the predominant form. During LDs formation, diacylglycerol acyltransferases (DGAT), including DGAT1 and DGAT2, catalyze the covalent addition of a fatty acyl chain to diacylglycerol, resulting in the esterification and synthesis of TAGs (Wang et al., 2024). TAG is synthesized in the bilayers of the ER, and once a level of 5%–10% is reached, the proteins Fat Storage Inducing Transmembrane Protein 2 (FIT2) and Perilipin3 promote the development of TAG accumulates, LDs precursors (LDP) form “lens-like structures” (Walther et al., 2017). LDP is recruited to the site of LD biogenesis through interactions with LD markers, and at this site LDP bud from the ER into the cytoplasm (Cottier and Schneiter, 2022). LDP originate from the ER and subsequently mature within the cytoplasm (Wang et al., 2016; Demirel-Yalciner et al., 2024), Figure 1B.
The process of lipolysis entails the sequential hydrolysis of TAGs to form diacylglycerols (DAGs) and monoacylglycerols (MAGs), with the liberation of a fatty acids (FAs) at each stage. The final FAs is released upon hydrolysis of the MAG, accompanied by the generation of glycerol (G). Enzymes associated with lipolysis process include adipose triglyceride lipase (ATGL), hormone-sensitive lipase (HSL), and monoacylglycerol lipase/α/β hydrolase domain-6 (MGL/ABHD6) (Ding et al., 2024). Lipophagy mediates the transfer and degradation of triglyceride-containing LDs through the lysosomal pathway (Kaushik and Cuervo, 2015; Wei et al., 2024). The resulting fatty acids undergo β-oxidation in MT. While the regulatory mechanisms of lipophagy remain unclear, this process significantly impacts hepatic metabolic disorders. Further research is needed to elucidate its precise role in liver metabolism (Kaushik and Cuervo, 2015). During the lipolysis process, ATGL breaks down TAG into DAG and FAs. Subsequently, HSL hydrolyzes DAG into MAG and FAs. Finally, MAG is converted into G and FAs by monoacylglycerol lipase (MGL). For a long time, HSL was believed as the rate-limiting enzyme in TAG breakdown. But currently ATGL is the rate-limiting enzyme that catalyzes the first step of TG breakdown to G and FAs (Xie et al., 2024). Mutations in the ATGL gene cause neutral lipid storage disease and myopathy, and reduced ATGL expression has been found in NAFLD (Ghosh et al., 2016). Disruptions in LD metabolism is associated with various metabolic disorders (Carotti et al., 2020), Figure 1B. LDs also response to inflammation, combined with MT, ER, and peroxisomes. These complexes undergo changes in inflammatory stimuli, can supply FAs for LD growth, and support FAs efflux from LDs. Macrophages utilize LDs for inflammatory lipid transport and influence inflammatory lipid mediators, indicating the importance of organelles in the regulation of inflammatory lipid metabolism (Zimmermann et al., 2024). Multi-spectral organelle imaging can comprehensively display the mapping of metabolic organelles including catalase, MT, Golgi apparatus and lysosomes, LD and ER, and their interactions with macrophages. This method can be applied in the study of metabolic changes in macrophages especially lipids which are rapidly responsive, opening up new avenues for potentially targeting the treatment of pathological conditions characterized by dysregulated lipid metabolism.
LDs proteomics examined more than 200 proteins and functions that collaboratively regulate the droplets’ formation, stability, metabolism, and growth. The aforementioned proteins can be broadly classified into these categories: membrane-associated structures of LDs that protect LDs and regulate their function, e.g., PLIN (Perilipin) and LSD2 (Lysine-Specific Histone Demethylase 2); lipid-metabolising enzymes on the surface of the ER and LDs that synthesise and degrade neutral lipids, e.g., DGAT1 and HSL. Membrane transporter proteins on the surface of the ER and LDs that regulate the interaction of LDs with other cellular structures, e.g., RAB18 (Ras-Related Protein Rab-18, Member RAS Oncogene Family); signalling proteins involved in inflammation and signal transduction, e.g., MAPK (Mitogen-Activated Protein Kinase); and degradation-associated proteins that degrade LD-related proteins, e.g., UBXD8 (UBX Domain-Containing Protein 8) also known as FAF2 (Fas Associated Factor Family Member 2). Furthermore, additional proteins, including ribosomal proteins, histones and actin, are implicated in protein translation, chromosome assembly under stress and LD motility, respectively. Among which perilipins are ubiquitously found in the cytoplasmic LDs of mammalian cells, all play roles in LD function under differing conditions (Cinato et al., 2024). Five genes encode the five main perilipin (PLIN) family proteins (Sztalryd and Brasaemle, 2017), namely, PAT family including Perilipin A (PLIN A), Adipose Differentiation-Related Protein (ADRP), Tail-Interacting Protein 47 (TIP47), Adipocyte Protein S3-12(S3-12), and Oxidative Tissue-Enriched PAT Protein (OXPAT). Their function are various. PAT proteins regulate the lipases into LDs which promote LDs generation. Besides, PAT proteins can also control the lipolysis of stored NL via cytoplasmic lipases, maintain the morphology of LDs, and regulate the movement of LDs (Bickel et al., 2009). PLIN A is a surface protein on LDs that is identical to PLIN 1. PLIN A is predominantly expressed in WAT, and to a lesser extent is present in BAT, cardiac muscle liposarcoma, where its action functions in hormone-induced lipolysis large LD stabilization. PLIN one is a surface protein on LDs that facilitates the stabilization of LDs and lipolysis, which is mediated by lipases and cofactors. The PKA signaling is activated by PLIN one phosphorylation, then inducing the catabolism of TAG. This process has been linked to the development of metabolic diseases such as obesity, diabetes, endocrine disorders, and hypertension (Desgrouas et al., 2024). Furthermore, PLIN one was positively related to disease-free survival (DFS) in lung squamous cell carcinoma (Kim et al., 2023). The depletion of B-cell receptor-associated protein 31 (BAP31) inhibited adipogenesis and lipolysis, but promoted the aberrant enlargement of LDs by reducing the proteasomal degradation of PLIN1 (Wei et al., 2023). ADRP is known as PLIN two that mainly expressed in liver, followed by premature adipocytes, macrophages, sebocytes, mary gland epithelia, choriocaricinoma cells. The functions of PLIN2 include the differentiation of adipocytes, the generation of small LDs, and the stabilization of LDs. ADRP can target adenosine monophosphate-activated protein kinase alpha (AMPKα)-dependent lipophagy, mediated by the Perilipin 2-lysosomal acid lipase (PLIN2-LIPA) axis which ameliorates NAFLD (Fang et al., 2024). ADRP knockout inhibits the induction of platelet-derived growth factor (PDGF) and suppresses vascular smooth muscle cell (VSMC) proliferation in atherosclerotic lesions, as evidenced by a decline in extracellular signal-regulated kinase (ERK) activity and protein kinase B signaling pathways (Zhao et al., 2017). TIP47 is called PLIN three that mainly expressed in ubiquitous but also in skeletal muscle neutrophils, mast cells retinal pigment epithelium sebocytes and its action function is LD stabilization (compensation of PLIN2) PGE2 production intracellular trafficking. PLIN-3 induced apoptosis in CD8+ T lymphocytes and promoted Programmed Cell Death 1 Ligand 1 (PD-L1) and B7 Homologue 2 (B7-H2) expression in oral squamous cell carcinoma (OSCC). PLIN-3 knockdown in tumors reduces LDs and tumor migration (He et al., 2024). S3-12 is known as PLIN 4, predominantly expressed in WAT and secondarily occurs in hMSC (Musculin), which is induced during differentiation of skeletal muscle. PLIN four plays a major role in human adipocyte differentiation. Moreover, S3-12 binds to the surface of nascent LDs promoting TAG synthesis in a time-substrate- insulin-dependent manner in LDs generation (Wolins et al., 2003). OXPAT is named of PLIN 5, which is expressed predominantly in cardiac muscle BAT skeletal muscle and to a lesser extent in slet β-cells hepatic stellate cells, where it primarily plays a role in LD stabilisation FA supply to MT. Through augmentation of FAs uptake and elevation of the expression levels of enzymes associated with oxidative catabolism, OXPAT facilitates mitochondrial FAO (Wolins et al., 2006). The heterogeneity of LDAP in different cells and tissues is the result of a combination of factors, including differences in cell type and function, differences in the regulation of protein expression, differences in the intracellular environment, and differences in the interaction of LDs with other organelles. These differences enable lipid droplet proteins to fulfil specific roles in different physiological and pathological states. The proper functioning of LADP is essential for maintaining cellular energy balance and metabolic health. However, disruptions in LADP function are increasingly being recognized as significant contributors to various diseases.
The outer membrane (OM) separates the MT from the cytoplasm While the inner membrane (IM) forms the mitochondrial matrix. The IM is divided into an inner boundary membrane, parallel to the OM, and cristae. Mic60, a component of the MT’s contact site and cristae organizing system, is systematically distributed at cristae junctions to support the mitochondrial structure (Stoldt et al., 2019). Carnitine palmitoyltransferase, a crucial enzyme in lipid β-oxidation, is located on the OM and catalyzes the conversion of palmitoyl CoA to palmitoyl carnitine, a process that is essential for the complete oxidative catabolism of FAs.
MT supply energy for the body, while liver, skeletal muscle, cardiovascular and brown adipocytes are the most energetic organs. In LDs metabolism, MT is involved not only in lipogenesis but also in FAO, processes that are intricately linked to mitochondrial dynamics, including movement, fusion, and fission. Microtubule stabilizers induce mitochondrial fusion, activate the mechanistic target of rapamycin (mTOR) signaling pathway and enhance ATP production (Cho et al., 2021; de Mello et al., 2018). Based on the specific proteins of MT and their roles in FAs and pyruvate oxidation, these organelles are categorized into PDM and CM, both of which coexist within the same cell to facilitate lipid oxidation and synthesis (Benador et al., 2018). The functions of PDM and CM differ mainly because the different membrane surface proteins of each play different roles and are closely linked to the interaction with the microenvironment and other organelles. The growth and degradation processes of LDs, the concentration of surrounding FAs and the local redox state are bound to affect PDM (Mahdaviani et al., 2017). In BAT, PDM has stronger pyruvate oxidation, electron transfer and ATP synthesis capabilities. It can also support LD amplification by esterifying FAs into triglycerides with the provision of ATP. CM shows stronger FAO ability (Benador et al., 2018). In WAT, PDM has stronger attachment to LDs through specific protein-protein or protein-lipid interactions, but has lower respiratory and ATP synthesis capabilities than CM. Moreover, the heterogeneity of PDM function can be determined by the size of LDs. The respiratory capacity of PDM is negatively correlated with the size of LDs (Brownstein et al., 2024). The reason for the gap between PDM attached to smaller LDs in WAT having higher respiratory capacity under pyruvate and PDM attached to larger LDs in BAT having higher ATP synthesis ability is that BAT has greater de novo lipogenesis and TAG turnover capacity, which is closely related to ATP synthesis and respiration, Figure 2. Naturally, PDM is not exclusive to adipose tissue, and it is likewise found in cardiomyocytes.
Transmission electron microscopy (TEM) can directly identify PDM, allowing observation of its morphological features (Benador et al., 2018; Freyre et al., 2019; Tarnopolsky et al., 2007), including the arrangement of cristae in PDM towards LDs (Benador et al., 2018; Herms et al., 2015). Confocal imaging microscopy allows real-time observation of the dynamics of LD-MT (Stone et al., 2009; Wang et al., 2011) by double staining LD and MT markers in tissues marker (Benador et al., 2018). Besides, protein blot analysis and silver staining assay are applied to LD-MT mutual studies (Freyre et al., 2019; Cui et al., 2019; Yu et al., 2015), such as detecting PLIN5, mitochondrial proteins, and PLIN2. In addition, proximity ligation assay (PLA) and subcellular fractionation can also assess the status of mitochondria-associated endoplasmic ER membranes (Yang et al., 2019). Differential centrifugation and proteases could successfully separate PDM from LDs (Benador et al., 2018). However, centrifugation alone may not fully eliminate MT from LDs completely, proteases are required for efficient separation (Cui et al., 2019). In WAT, treating PDM with Proteinase K (Prot-K) before differential centrifugation could improve separation efficacy (Brownstein et al., 2024).
MT produces ATP and participates in lipid metabolism within LDs. When the cell requires energy, TAG is hydrolyzed to FAs, serving as energy carriers, Figure 1B. FAs are transported intracellularly to various cellular compartments by binding to the cytoplasmic lipid-binding protein, which maintains the solubility of FAs. MT convert FAs to lipoyl-coenzyme A (acyl-CoA) through the carnitine palmitoyltransferase (CPT1/2) system. Firstly, FFAs are catalyzed by acyl-CoA synthase and carnitine acyl transferase I (CPT1) on the OM of MT to form acyl-CoA and acyl-carnitine (AC). Carnitine-acylcarnitine translocase facilitates the transfer AC across the IM into the mitochondrial matrix. AC and CoA are enzymatically reconverted to acyl-CoA by carnitine acyl transferase II (CPT2) on the IM for β-oxidation (Talari et al., 2023), Figure 1C. FFAs are β-oxidized by highly FAO-capable CM to generate acetyl-CoA. Acetyl-CoA serves as a substrate in the tricarboxylic acid cycle (TCA) and oxidative phosphorylation to produce ATP for cells. The translocation of FAs from the LDs to the MT, which necessitates close contact between the two for lipid transfer, reduces FAs exposure and cellular lipotoxicity. In contrast, the enhanced ATP synthesis capacity of PDM facilitates the esterification of FAs to TAG and encourages LDs amplification. Additionally, PDM plays a pivotal role in the conversion of pyruvate to acetyl-CoA, which subsequently undergoes carboxylation by acetyl-CoA carboxylase (ACC) to form malonyl-CoA, an essential precursor for FA biosynthesis (Benador et al., 2018). When pyruvate oxidation through PDM is enhanced, acetyl-CoA levels significantly increase in the mitochondrial matrix. This elevation also impacted malonyl-CoA levels (Benador et al., 2018). At this juncture, malonyl-CoA acts as an inhibitory factor for CPT1, thus effectively blocking the entry of FAs into the MT (McGarry et al., 1978), Figure 2.
The interactions between LDs and MT are facilitated by specific contact sites, Table 1. The expression levels and activities of proteins involved in the interaction between LDs and MT exhibit significant heterogeneity across different tissues. This heterogeneity can be attributed to the fact that the gene expression patterns of different tissue cell types are tailored (Elmentaite et al., 2022) to meet the unique energy metabolism and cellular function requirements of their respective tissues (Ouyang et al., 2023; Ferreira et al., 2018). In liver, PLIN5 enhanced LDs formation and increased contacts between LDs and MT (Tan et al., 2019). When high fat diet (HFD), Rab32 localizes to LDs and MT, targeting LDAP-associated proteins Atgl and Plin5 to promote LDs accumulation and inhibition MT biosynthesis, fusion and oxidation. Inhibition of the Creb-Pgc1α pathway blocked Rab32 localization to LDs and MT, suggesting that HFD may target LD-MT and then regulate hepatic LDs lipolysis (Song et al., 2020). Synaptosome-associated protein 23 (SNAP23) and long-chain acyl-CoA synthetase 1 (ACSL1) are situated on the OM of MT, which promote FAs β-oxidation (Che et al., 2023). SNAP23 may be crucial for the LD-MT complex (Jägerström et al., 2009), when ablation would inhibit the complex and β-oxidation. In adipose tissue, mitoguardin 2 (MIGA2) located on OM of MT, facilitate the synthesis of TAG from non-lipid precursors (Freyre et al., 2019). The interaction between PLIN1 and mitofusin 2 (MFN2) also facilitated the association of MT with LDs (Boutant et al., 2017). MT typically accumulate around LDs in adipocytes. Caveolin-1 is involved in LDs and MT interaction, when knockout in adipocytes display the disappearance of MT around LDs and alters the spatial structure between the LDs surface and the cytoplasm, resulting in reduced lipolysis (Cohen et al., 2004). In skeletal muscle, LD-associated PLIN5 established a binding complex with the mitochondrial receptor Rab8a at the surface of LDs, facilitating LDs hydrolysis and delivery of FAs to MT for β-oxidation (Ouyang et al., 2023), Figure 1A.
The contacts between LDs and MT are displayed dynamic and stable, which could not be completely separated during ultracentrifugation. Morphological studies reveal that the interaction between LDs and MT are highly dynamic, consistent with the 'kiss and run’ model. Electron microscope observed that the interaction between LDs and MT in skeletal muscle increases with exercise (Tarnopolsky et al., 2007). Furthermore, there is experimental evidence from electron microscopy that contact between the two varies with experimental conditions (Valm et al., 2017). Despite the publication of a limited number of precise LD-MT interaction protein sites, the molecular biology of how target proteins regulate the binding or separation of the two remains unclear. This represents a significant avenue for future research.
The attachment of regulatory proteins associated with LDs and MT interactions represents a complex biological process. In addition to being influenced by the localization and expression of proteins, the spatial distance and nourishment are also significant factors. This section is dedicated to the non-protein factors, and how specific proteins regulate LDs and MT binding and segregation is detailed in subsequent sections.
MT surrounding LDs were observed in adipocytes, with similar findings in liver cells (Kalashnikova and Fadeeva, 2006). TEM revealed direct contact between LDs and MT. Studies show an association between the size of LDs and their proximity to MT, indicating that larger LDs were more likely to be closely associated with MT, and more efficient FAs transportation. The spatial distance between LDs and MT may affect FAs transportation and lipid metabolism.
Insufficient nutrients activate autophagy to release FAs, which convert to acylcarnitines on the OM of MT by binding to the coenzyme A (COA) moiety. FAs are then transported into the MT matrix via the CPT1/2 system and participate in FAO and oxidative phosphorylation to generate ATP (Nguyen et al., 2017). When overnutrition, overproduced pyruvate, the high pyruvate oxidation capacity in PDM generates substantial malonyl-CoA, which inhibits CPT1 and negatively regulates the transfer of FAs between LDs and MT, thus reducing the rate of lipid metabolism and energy production (McGarry et al., 1978). Conversely, diminished pyruvate oxidation capacity in the CM results in decreased levels of citrate and malonyl-CoA, thereby promoting the translocation of FAs to MT by CPT1 for β-oxidation, Figure 2.
The LD-MT interaction sites known from current studies in the liver are Rab32, ACSL1-SNAP23, p53-PLIN2, and PLIN5. The liver is indispensable in the regulation of lipid metabolism. Rab proteins (Nguyen et al., 2016) are associated with intracellular LDs, and Rab32 is the Rab GTPase associated with MT (Bui et al., 2010). The knockdown of Rab32 promotes lipolysis by indirectly increasing the expression of adipose ATGL (Li et al., 2016). Hepatic FAs significantly upregulate Pgc1α and induce Rab32 localization to LD and MT (Song et al., 2020). NAFLD is one of the most common liver diseases worldwide, characterized by elevated levels of reactive oxygen species (ROS), and Bailey (Bailey et al., 2015) demonstrated that LDs can act as antioxidant organelles. Overexpression of PLIN5 promotes the formation of LDs and LD-MT contacts, reduces cellular levels of ROS and upregulates genes related to mitochondrial function. This represents a survival strategy adopted by cells in response to stress and is a promising new target (Tan et al., 2019). Moreover, oleic acid (OA) increases PLIN5 via the PI3K/PPAR(Peroxisome Proliferator-Activated Receptor) α pathway (Zhong et al., 2019), and IL-6 increases PLIN5 through the JAK/STAT3 axis (Krizanac et al., 2023). The increase in PLIN5 promotes the contact between LD and MT, reduces the cellular levels of ROS. In contrast, Chaperone-mediated autophagy (CMA) induces Plin5 degradation (Ma et al., 2020). In addition, lipid metabolism defects not only cause NAFLD but also insulin resistance (IR). Abnormal lipid metabolism in hepatocytes leads to increased ROS. These responses in turn affect insulin signalling in the liver, resulting in decreased liver sensitivity to insulin. This leads to a reduction in hepatic uptake and utilisation of glucose and exacerbates IR (Tilg et al., 2021). The livers of Plin5-deficient mice exhibited activation of c-Jun N-terminal kinase, impaired insulin signal transduction, and IR, which impaired systemic insulin action and glycemic control. The re-expression of Plin5 reversed these effects (Keenan et al., 2019). Similarly, 17β-HSD13 functions as a hepatocyte-specific LDAP, and its expression is upregulated in patients with NAFLD (s). Besides, comparative proteomic study reveals 17β-HSD13 as a pathogenic protein in NAFLD (Su et al., 2014). Inadequate dietary choline intake is associated with choline deficiency (CD), which induces NAFLD (Michel et al., 2011). Knockdown of serine/threonine kinase (STK) on the surface of hepatic LDs increases lipolysis and protects hepatocytes (Kim et al., 2023). Another new entry point for the treatment of NAFLD is the NR4A1 (nuclear receptor subfamily four group A member 1)/DNA-PKcs (DNA-dependent protein kinase catalytic subunit)/p53 pathway. The regulation of p53 is bidirectional, driving MT fission on the one hand, and stalling MT autophagy on the other to maintain MT homeostasis. Furthermore, melatonin blocks the NR4A1/DNA-PKcs/p53 pathway, promotes mitochondrial autophagy, and enhances NAFLD (Zhou et al., 2018a). ACSL1 is situated in the mitochondrial OM. The direct interaction with CPT - one leads FAs from LDs to the MT for oxidation, while the tethering of SNAP23 is not required (Young et al., 2018). The liver injury results in increased energy expenditure and weight loss, with ACSL1 content being linearly correlated to body weight (Khamoui et al., 2020). Elevated ACSL1 expression decreases β-oxidation (Li et al., 2020). Furthermore, miR-34c promotes liver fibrosis by inhibiting ACSL1 expression (Li et al., 2021). PLIN2 deficiency attenuates hepatic steatosis. The lack of Cannabinoid receptor 1 (CB1) signaling results in a decrease in PLIN2 levels through the CB1-perilipin2 axis, which opens up ideas for the treatment of steatosis (Irungbam et al., 2020). In alcoholic fatty liver disease (AFLD), p53 interacts directly with ALDH2, inhibiting the formation of reactive tetramers and indirectly limiting pyruvate production (Yao et al., 2023). PLIN2 inhibits the adenosine 5′-AMPK/ULK1 (human) recombinant protein-lysosome pathway and promotes cell proliferation in hepatocellular carcinoma (HCC) (Liu et al., 2022). Additionally, RAB32 is a crucial target of miR - 30c - 5p in HCC. miR - 30c - 5p inhibits the growth and invasion of HCC cells via the miR - 30c - 5p - RAB32 axis (He et al., 2021).
The LD-MT sites are mainly MIGA2, PLIN1-MFN2. High MIGA2 expression not only promotes LD-MT contact but also effectively activates adipocytes to synthesize TAG from non-lipid precursors (Freyre et al., 2019). The direct interaction between Mfn2 and PLIN1 is conducive to the entry of FAs into MT and FAO. Mfn2 knockout would impair the fat utilization in mice even under a low-fat diet (LFD), increasing the incidence of obesity (Boutant et al., 2017).
Skeletal muscle activity requires a large supply of mitochondrial energy, and the known LD-MT sites are PLIN5-Rab8A and PLIN2. PLIN5 mediates LD-MT coupling (LDMC) to enhance mitochondrial respiratory capacity, and its abundance correlates with mitochondrial respiration rates (Bosma et al., 2012; Kien et al., 2022). Vitamin D upregulates PLIN2 levels. In myotubes, calcitriol (the active form of vitamin D) increases the mRNA of triglyceride synthesis genes DGAT1 and DGAT2, partially mediated by PLIN2 for mitochondrial oxidative function (Schnell et al., 2019). Interestingly, overfeeding upregulates PLIN2 expression, but has no effect on ROS release in vivo (Toledo et al., 2018). Vascular endothelial growth factor B (VEGFB) upregulates fatty acid transporter protein 4 (FATP4) and FATP1, inhibiting FASN production (Li et al., 2019). Caffeine promotes FAs utilization and FAO (Enyart et al., 2020).
Myocardial disease is the most common cause of mortality and disability globally (Christiansen et al., 2017; Wende et al., 2017). A certain degree of LDs can alleviate oxidative stress in cells (Jarc and Petan, 2019). Oxidative stress is an imbalance between ROS and antioxidants in cells (Burgoyne et al., 2012). Under physiological conditions, ROS regulates numerous cellular processes at low concentrations (Lennicke and Cochemé, 2021); however, overproduction of ROS results in impaired cellular components and function (Ong et al., 2015; Humeres and Frangogiannis, 2019), triggering adverse cardiac remodeling and progression to heart failure (De Geest and Mishra, 2022; D'Oria et al., 2020). ROS induces LDs accumulation by increasing PLIN2 expression, and PLIN2 modulates LDs formation via PPAR and CREBBP (CREB Binding Protein) signaling pathways (Jin et al., 2018a). In mouse hearts, Plin2 is upregulated during fasting-induced steatosis (Suzuki et al., 2009; Ueno et al., 2017). Moreover, Sato demonstrated that Plin2-induced cardiac steatosis leads to an increased incidence of atrial fibrillation in aged mice (Sato et al., 2019). PLIN5 expression inhibits ROS (Zheng et al., 2017). LDs prevent excess ROS production by decreasing FAO (Kuramoto et al., 2012). Plin5 knockout mice exhibit significantly reduced TAG accumulation in cardiomyocytes (Drevinge et al., 2016), increased cardiac hypertrophy, and elevated myocardial oxidative stress following transaortic constriction (Wang et al., 2019). Thus, Plin5-deficient myocardium elevates levels of ROS (Zheng et al., 2017), suggesting that Plin5 deficiency reduces cardiac function.
There are few drug studies showing intervention at the LD-MT interaction site, and to better provide new insights into the treatment and prevention of obesity, we have collected a wide range of drug studies related to LD synthetic catabolism and LD-MT interactions.
PPAR-γ, SREBP-1 and C/EBPα/β targets such as FASN, HSL, ATGL, fatty acid binding protein (FABP) and LDAP regulating LDs synthesis and catabolism. PPARs promote LDs accumulation in the liver. Octyl gallate (OG) (Lima et al., 2020) and p-coumaric acid (p-CA) (Yuan et al., 2023) upregulate PPAR-γ expression. Ochratoxin A (OTA) upregulates PPAR γ levels, and induces hepatic steatosis through the PPAR γ-CD36 axis (Zheng et al., 2021). Similarly, the extract from Syzygium simile leaves (SSLE) decreases CD36 expression, hindering cellular LDs accumulation (Yen et al., 2018). Additionally, p-AMPK reduces LD accumulation. The TF3-PK-AMPK regulatory axis is a novel mechanism to alleviate lipid deposition. The theaflavin monomer theaflavin-3,3′-digallate (TF3) acts on the TF3-PK-AMPK regulatory axis and activates AMPK (Zhang et al., 2020a). Conversely, DHA inhibits AMPK phosphorylation (Xia et al., 2021). Additionally, oroxylin A inhibits HIF-1α expression in vivo, suppressing LDs accumulation (Jin et al., 2018b). Furthermore, Quercetin induces NAFLD by inhibiting AKT via the PI3K/AKT pathway and promoting FAs synthesis (Li et al., 2023a).
In skeletal muscle, vitamin D reduced PPAR-γ levels in vivo, subsequently lowering PLIN2 expression and decreasing LDs in skeletal muscle (Li et al., 2018). Glucagon-like peptide-1 receptor agonists (GLP-1RA) and semaglutide enhance the Sirtuin1 (SIRT1) signaling pathway, leading to a downregulation of the atrophy-associated factor Atrogin-1 and an increase in myogenic factor expression, which alleviating muscle atrophy and enhancing IR (Xiang et al., 2023).
Also in adipocytes, Polysaccharide CM1 diminishes PPAR-γ, DGAT1, and DGAT2 (Yu et al., 2021). Lemon extract (LE) (Carota et al., 2021) and triterpenoid cycloastragenol (CAG) (Kim et al., 2024) downregulate PPAR-γ in 3T3-L1 cells. Curcumin and Synthetic Curcumin Derivatives also downregulate PPAR-γ, suppress COX2, inhibit FASN (Moetlediwa et al., 2024). Mulberry and Hippophae-based solid beverages inhibit TGF-β and PPAR-γ signaling pathways, restoring WAT dysfunction (Zhou et al., 2024). Polychlorinated biphenyls (PCBs) upregulate PPAR-γ and induce fat-specific protein 27 (Fsp27) expression, resulting in IR (Kim et al., 2017). Other pathways that modulated the increase in adipocyte LDs included clozapine, which significantly and directly reduced leptin secretion in 3T3-L1 adipocytes (Tsubai et al., 2017). In fascia-derived stromal cells (FSC), suramin significantly increase PPAR-γ, consequently elevating the expression of PLIN2, causing LDs accumulation (Li et al., 2023b). Rutin directly activates the SIRT1/PGC-1α/mitochondrial transcription factor (Tfam) signaling pathway increasing MT and UCP1 in BAT, ultimately enhancing energy expenditure (Yuan et al., 2017). Olanzapine can increase PLIN1, PLIN2 and PLIN4 expression (Nimura et al., 2015). Deoxyschizandrin (DS) and DS-liposomes (DS-liposomes) in 3T3-L1 adipocytes reduce LDL in the cytoplasm, alleviating NAFLD (Liu et al., 2018).
Drugs have the potential to directly target LD-MT interaction proteins to modify the oxidative catabolic process of LDs, aiming to intervene in obesity. Statins reduce hepatocyte TAG content by inhibiting PLIN5 expression (Langhi et al., 2014) Atorvastatin ameliorates NAFLD by enhancing PLIN5 phosphorylation, and reducing TAG accumulation in the liver (Gao et al., 2017). Additionally, glycocoumarin (GCM) regulates the PLIN5-Sirt1 axis, alleviating hepatic lipotoxicity (Zhang et al., 2020b). Resveratrol (RES) inhibits the thioacetamide (TAA)-induced TNF-alpha (inflammatory)/NF-kB (nuclear factor-kappa B)/iNOS (nitrosative stress)/HIF-1α axis (Ebrahim et al., 2022), and ameliorates hepatic steatosis (Zhou et al., 2018b). Tumor suppressor protein p53 reduces LDs (Borude et al., 2018).
Drugs affect relevant LD-MT metabolites, which modulate lipid metabolism and influence obesity. Among them, CPT1/2, PGC-1α (PPAR coactivator), and AMPK on the MT membrane play a central role. PGC-1α serves as a transcriptional co-activator whose upregulation increases FAO. Pioglitazone upregulates PGC-1α and ameliorates IR (Tan et al., 2020). Conversely, Simvastatin disrupts the Akt/mTOR pathway, hampered insulin receptor and mTORC2 function, inducing IR (Sanvee et al., 2019; Bonifacio et al., 2015). Interestingly, vincristine impairs glycogen muscle reserve in normal mice but not in PGC-1α overexpressing mice, suggesting a role for PGC-1α in preventing simvastatin-associated myotoxicity (Panajatovic et al., 2021; Panajatovic et al., 2020). In addition, dagliflozin treatment for 5 weeks increased CPT1 (Op den Kamp et al., 2022), Table 2.
In lipid metabolism, LDs are involved in membrane synthesis and store neutral lipids and proteins, through interactions with membrane-contact sites in various organelles (Zadoorian et al., 2023; Gross and Silver, 2014). It also regulated functions such as lipase entry into the LDs and maintenance of LDs morphology and motility with the assistance of the LDAP protein family (Bickel et al., 2009). The pyruvate oxidation capacity of PDM in WAT was significantly greater than that of CM (Benador et al., 2018). However, the mechanism behind this enhanced pyruvate oxidation capacity remains unknown. Moreover, PDM and CM have been individually researched in BAT and WAT, but the interconnections need to go deep.
There is substantial scientific evidence to suggest that LD-MT interplay is of great significance to human health (Fan and Tan, 2024). Despite there is a large number of data on LD-MT interplay, the molecular composition and regulation of LD-MT tethering is still unknown. Consequently, future research endeavors should prioritize the identification of novel interacting proteins. LD-MT interacts on the surfaces of LDs and MT with surface proteins, e.g., PLIN5 playing a significant role. Future research can continue exploring contact proteins that interact with PLIN5 in the liver, aiming to enhance understanding of the underlying mechanisms in studies centered on the interplay between LDs and MT.
Medications that target the LDs sites to regulate cellular lipid synthesis and lipolysis. Regarding LD-MT interaction sites, medications can directly impact the oxidative catabolic process of LDs. For instance, statins reduce hepatocyte TAG content by inhibiting PLIN5 expression (Langhi et al., 2014). There is limited research on drugs targeting the LD-MT target, and additional studies are necessary to investigate whether other drugs that promote fat reduction and metabolic enhancement inhibit or reduce body weight through this mechanism. We anticipate the development of potent and side-effect-free medications targeting alternative pathways for treating obesity-related conditions. These advancements will offer novel approaches for managing metabolic disorders associated with obesity.
CZ: Conceptualization, Software, Supervision, Writing–original draft, Writing–review and editing. MZ: Conceptualization, Methodology, Resources, Software, Writing–review and editing. RB: Conceptualization, Resources, Software, Validation, Writing–review and editing. JC: Software, Visualization, Writing–review and editing. HY: Project administration, Resources, Validation, Writing–review and editing. GL: Resources, Validation, Writing–review and editing.
The author(s) declare that financial support was received for the research, authorship, and/or publication of this article. This research was funded by “Xinglin Scholars” Discipline Talent Research Enhancement Program of Chengdu University of TCM (No. MPRC2022023), Chengdu Health Commission Research Project (No. 202317033303). The APC was supported by the National Natural Science Foundation of China (NO. 82405158).
The authors declare that the research was conducted in the absence of any commercial or financial relationships that could be construed as a potential conflict of interest.
All claims expressed in this article are solely those of the authors and do not necessarily represent those of their affiliated organizations, or those of the publisher, the editors and the reviewers. Any product that may be evaluated in this article, or claim that may be made by its manufacturer, is not guaranteed or endorsed by the publisher.
Bailey A. P., Koster G., Guillermier C., Hirst E. M., MacRae J. I., Lechene C. P., et al. (2015). Antioxidant role for lipid droplets in a stem cell niche of Drosophila. Cell 163 (2), 340–353. doi:10.1016/j.cell.2015.09.020
Benador I. Y., Veliova M., Mahdaviani K., Petcherski A., Wikstrom J. D., Assali E. A., et al. (2018). Mitochondria bound to lipid droplets have unique bioenergetics, composition, and dynamics that support lipid droplet expansion. Cell Metab. 27 (4), 869–885. doi:10.1016/j.cmet.2018.03.003
Bickel P. E., Tansey J. T., Welte M. A. (2009). PAT proteins, an ancient family of lipid droplet proteins that regulate cellular lipid stores. Biochim. Biophys. Acta 1791 (6), 419–440. doi:10.1016/j.bbalip.2009.04.002
Bonifacio A., Sanvee G. M., Bouitbir J., Krähenbühl S. (2015). The AKT/mTOR signaling pathway plays a key role in statin-induced myotoxicity. Biochim. Biophys. Acta 1853 (8), 1841–1849. doi:10.1016/j.bbamcr.2015.04.010
Bórquez J. C., Díaz-Castro F., La Fuente F. P., Espinoza K., Figueroa A. M., Martínez-Ruíz I., et al. (2024). Mitofusin-2 induced by exercise modifies lipid droplet-mitochondria communication, promoting fatty acid oxidation in male mice with NAFLD. Metabolism 152, 155765. doi:10.1016/j.metabol.2023.155765
Borude P., Bhushan B., Gunewardena S., Akakpo J., Jaeschke H., Apte U. (2018). Pleiotropic role of p53 in injury and liver regeneration after acetaminophen overdose. Am. J. Pathol. 188 (6), 1406–1418. doi:10.1016/j.ajpath.2018.03.006
Bosma M., Minnaard R., Sparks L. M., Schaart G., Losen M., de Baets M. H., et al. (2012). The lipid droplet coat protein perilipin 5 also localizes to muscle mitochondria. Histochem Cell Biol. 137 (2), 205–216. doi:10.1007/s00418-011-0888-x
Boutant M., Kulkarni S. S., Joffraud M., Ratajczak J., Valera-Alberni M., Combe R., et al. (2017). Mfn2 is critical for brown adipose tissue thermogenic function. Embo J. 36 (11), 1543–1558. doi:10.15252/embj.201694914
Brownstein A. J., Veliova M., Acin-Perez R., Villalobos F., Petcherski A., Tombolato A., et al. (2024). Mitochondria isolated from lipid droplets of white adipose tissue reveal functional differences based on lipid droplet size. Life Sci. Alliance 7 (2), e202301934. doi:10.26508/lsa.202301934
Bui M., Gilady S. Y., Fitzsimmons R. E., Benson M. D., Lynes E. M., Gesson K., et al. (2010). Rab32 modulates apoptosis onset and mitochondria-associated membrane (MAM) properties. J. Biol. Chem. 285 (41), 31590–31602. doi:10.1074/jbc.M110.101584
Burgoyne J. R., Mongue-Din H., Eaton P., Shah A. M. (2012). Redox signaling in cardiac physiology and pathology. Circ. Res. 111 (8), 1091–1106. doi:10.1161/circresaha.111.255216
Carota G., Raffaele M., Amenta M., Ballistreri G., Fabroni S., Rapisarda P., et al. (2021). In vitro effects of bioflavonoids rich lemon extract on pre-adipocyte differentiation. Nat. Prod. Res. 35 (22), 4774–4778. doi:10.1080/14786419.2020.1721493
Carotti S., Aquilano K., Zalfa F., Ruggiero S., Valentini F., Zingariello M., et al. (2020). Lipophagy impairment is associated with disease progression in NAFLD. Front. Physiol. 11, 850. doi:10.3389/fphys.2020.00850
Che L., Huang J., Lin J. X., Xu C. Y., Wu X. M., Du Z. B., et al. (2023). Aflatoxin B1 exposure triggers hepatic lipotoxicity via p53 and perilipin 2 interaction-mediated mitochondria-lipid droplet contacts: an in vitro and in vivo assessment. J. Hazard Mater 445, 130584. doi:10.1016/j.jhazmat.2022.130584
Chen C., Ye Y., Zhang Y., Pan X. F., Pan A. (2019). Weight change across adulthood in relation to all cause and cause specific mortality: prospective cohort study. Bmj 367, l5584. doi:10.1136/bmj.l5584
Cho M. J., Kim Y. J., Yu W. D., Kim Y. S., Lee J. H. (2021). Microtubule integrity is associated with the functional activity of mitochondria in HEK293. Cells 10 (12), 3600. doi:10.3390/cells10123600
Christiansen M. N., Køber L., Weeke P., Vasan R. S., Jeppesen J. L., Smith J. G., et al. (2017). Age-specific trends in incidence, mortality, and comorbidities of heart failure in Denmark, 1995 to 2012. Circulation 135 (13), 1214–1223. doi:10.1161/circulationaha.116.025941
Cinato M., Andersson L., Miljanovic A., Laudette M., Kunduzova O., Borén J., et al. (2024). Role of perilipins in oxidative stress-implications for cardiovascular disease. Antioxidants (Basel) 13 (2), 209. doi:10.3390/antiox13020209
Cohen A. W., Razani B., Schubert W., Williams T. M., Wang X. B., Iyengar P., et al. (2004). Role of caveolin-1 in the modulation of lipolysis and lipid droplet formation. Diabetes 53 (5), 1261–1270. doi:10.2337/diabetes.53.5.1261
Cottier S., Schneiter R. (2022). Lipid droplets form a network interconnected by the endoplasmic reticulum through which their proteins equilibrate. J. Cell Sci. 135 (5), jcs258819. doi:10.1242/jcs.258819
Cui L., Mirza A. H., Zhang S., Liang B., Liu P. (2019). Lipid droplets and mitochondria are anchored during brown adipocyte differentiation. Protein Cell 10 (12), 921–926. doi:10.1007/s13238-019-00661-1
De Geest B., Mishra M. (2022). Role of oxidative stress in diabetic cardiomyopathy. Antioxidants (Basel) 11 (4), 784. doi:10.3390/antiox11040784
de Mello A. H., Costa A. B., Engel J. D. G., Rezin G. T. (2018). Mitochondrial dysfunction in obesity. Life Sci. 192, 26–32. doi:10.1016/j.lfs.2017.11.019
Demirel-Yalciner T., Cetinkaya B., Sozen E., Ozer N. K. (2024). Impact of Seipin in cholesterol mediated lipid droplet maturation; status of endoplasmic reticulum stress and lipophagy. Mech. Ageing Dev. 219, 111933. doi:10.1016/j.mad.2024.111933
Desgrouas C., Thalheim T., Cerino M., Badens C., Bonello-Palot N. (2024). Perilipin 1: a systematic review on its functions on lipid metabolism and atherosclerosis in mice and humans. Cardiovasc Res. 120 (3), 237–248. doi:10.1093/cvr/cvae005
Ding L., Huwyler F., Long F., Yang W., Binz J., Wernlé K., et al. (2024). Glucose controls lipolysis through Golgi PtdIns4P-mediated regulation of ATGL. Nat. Cell Biol. 26 (4), 552–566. doi:10.1038/s41556-024-01386-y
D'Oria R., Schipani R., Leonardini A., Natalicchio A., Perrini S., Cignarelli A., et al. (2020). The role of oxidative stress in cardiac disease: from physiological response to injury factor. Oxid. Med. Cell Longev. 2020, 5732956. doi:10.1155/2020/5732956
Drevinge C., Dalen K. T., Mannila M. N., Täng M. S., Ståhlman M., Klevstig M., et al. (2016). Perilipin 5 is protective in the ischemic heart. Int. J. Cardiol. 219, 446–454. doi:10.1016/j.ijcard.2016.06.037
Ebrahim H. A., Kamar S. S., Haidara M. A., Latif N. S. A., Ellatif M. A., ShamsEldeen A. M., et al. (2022). Association of resveratrol with the suppression of TNF-α/NF-kB/iNOS/HIF-1α axis-mediated fibrosis and systemic hypertension in thioacetamide-induced liver injury. Naunyn Schmiedeb. Arch. Pharmacol. 395 (9), 1087–1095. doi:10.1007/s00210-022-02264-w
Elmentaite R., Domínguez Conde C., Yang L., Teichmann S. A. (2022). Single-cell atlases: shared and tissue-specific cell types across human organs. Nat. Rev. Genet. 23 (7), 395–410. doi:10.1038/s41576-022-00449-w
Enyart D. S., Crocker C. L., Stansell J. R., Cutrone M., Dintino M. M., Kinsey S. T., et al. (2020). Low-dose caffeine administration increases fatty acid utilization and mitochondrial turnover in C2C12 skeletal myotubes. Physiol. Rep. 8 (1), e14340. doi:10.14814/phy2.14340
Fan H., Tan Y. (2024). Lipid droplet-mitochondria contacts in health and disease. Int. J. Mol. Sci. 25 (13), 6878. doi:10.3390/ijms25136878
Fang C., Liu S., Yang W., Zheng G., Zhou F., Gao X., et al. (2024). Exercise ameliorates lipid droplet metabolism disorder by the PLIN2-LIPA axis-mediated lipophagy in mouse model of non-alcoholic fatty liver disease. Biochim. Biophys. Acta Mol. Basis Dis. 1870 (3), 167045. doi:10.1016/j.bbadis.2024.167045
Ferreira P. G., Muñoz-Aguirre M., Reverter F., Cp S. G., Sousa A., Amadoz A., et al. (2018). The effects of death and post-mortem cold ischemia on human tissue transcriptomes. Nat. Commun. 9 (1), 490. doi:10.1038/s41467-017-02772-x
Freyre C. A. C., Rauher P. C., Ejsing C. S., Klemm R. W. (2019). MIGA2 links mitochondria, the ER, and lipid droplets and promotes de novo lipogenesis in adipocytes. Mol. Cell 76 (5), 811–825. doi:10.1016/j.molcel.2019.09.011
Gao X., Nan Y., Zhao Y., Yuan Y., Ren B., Sun C., et al. (2017). Atorvastatin reduces lipid accumulation in the liver by activating protein kinase A-mediated phosphorylation of perilipin 5. Biochim. Biophys. Acta Mol. Cell Biol. Lipids 1862 (12), 1512–1519. doi:10.1016/j.bbalip.2017.09.007
Ghosh M., Niyogi S., Bhattacharyya M., Adak M., Nayak D. K., Chakrabarti S., et al. (2016). Ubiquitin ligase COP1 controls hepatic fat metabolism by targeting ATGL for degradation. Diabetes 65 (12), 3561–3572. doi:10.2337/db16-0506
Gross D. A., Silver D. L. (2014). Cytosolic lipid droplets: from mechanisms of fat storage to disease. Crit. Rev. Biochem. Mol. Biol. 49 (4), 304–326. doi:10.3109/10409238.2014.931337
He Y., Liu L., Dong Y., Zhang X., Song Y., Jing Y., et al. (2024). Lipid droplets-related Perilipin-3: potential immune checkpoint and oncogene in oral squamous cell carcinoma. Cancer Immunol. Immunother. 73 (5), 78. doi:10.1007/s00262-024-03659-9
He Z., Tian M., Fu X. (2021). Reduced expression of miR-30c-5p promotes hepatocellular carcinoma progression by targeting RAB32. Mol. Ther. Nucleic Acids 26, 603–612. doi:10.1016/j.omtn.2021.08.033
Herms A., Bosch M., Reddy B. J., Schieber N. L., Fajardo A., Rupérez C., et al. (2015). AMPK activation promotes lipid droplet dispersion on detyrosinated microtubules to increase mitochondrial fatty acid oxidation. Nat. Commun. 6, 7176. doi:10.1038/ncomms8176
Humeres C., Frangogiannis N. G. (2019). Fibroblasts in the infarcted, remodeling, and failing heart. JACC Basic Transl. Sci. 4 (3), 449–467. doi:10.1016/j.jacbts.2019.02.006
Ibayashi M., Tatsumi T., Tsukamoto S. (2024). Perilipin2 depletion causes lipid droplet enlargement in the ovarian corpus luteum in mice. J. Reprod. Dev. 70, 296–302. doi:10.1262/jrd.2024-023
Irungbam K., Churin Y., Matono T., Weglage J., Ocker M., Glebe D., et al. (2020). Cannabinoid receptor 1 knockout alleviates hepatic steatosis by downregulating perilipin 2. Lab. Invest 100 (3), 454–465. doi:10.1038/s41374-019-0327-5
Jägerström S., Polesie S., Wickström Y., Johansson B. R., Schröder H. D., Højlund K., et al. (2009). Lipid droplets interact with mitochondria using SNAP23. Cell Biol. Int. 33 (9), 934–940. doi:10.1016/j.cellbi.2009.06.011
Jarc E., Petan T. (2019). Lipid droplets and the management of cellular stress. Yale J. Biol. Med. 92 (3), 435–452.
Jarc E., Petan T. (2020). A twist of FATe: lipid droplets and inflammatory lipid mediators. Biochimie 169, 69–87. doi:10.1016/j.biochi.2019.11.016
Jin H., Lian N., Bian M., Zhang C., Chen X., Shao J., et al. (2018a). Oroxylin A prevents alcohol-induced hepatic steatosis through inhibition of hypoxia inducible factor 1alpha. Chem. Biol. Interact. 285, 14–20. doi:10.1016/j.cbi.2018.02.025
Jin Y., Tan Y., Chen L., Liu Y., Ren Z. (2018b). Reactive oxygen species induces lipid droplet accumulation in HepG2 cells by increasing perilipin 2 expression. Int. J. Mol. Sci. 19 (11), 3445. doi:10.3390/ijms19113445
Kalashnikova M. M., Fadeeva E. O. (2006). Ultrastructural study of liver cells from rooks living in ecologically unfavorable areas. Izv. Akad. Nauk. Ser. Biol. (2), 133–141.
Kaushik S., Cuervo A. M. (2015). Degradation of lipid droplet-associated proteins by chaperone-mediated autophagy facilitates lipolysis. Nat. Cell Biol. 17 (6), 759–770. doi:10.1038/ncb3166
Kaze A. D., Santhanam P., Musani S. K., Ahima R., Echouffo-Tcheugui J. B. (2021). Metabolic dyslipidemia and cardiovascular outcomes in type 2 diabetes mellitus: findings from the look AHEAD study. J. Am. Heart Assoc. 10 (7), e016947. doi:10.1161/jaha.120.016947
Keenan S. N., De Nardo W., Lou J., Schittenhelm R. B., Montgomery M. K., Granneman J. G., et al. (2021). Perilipin 5 S155 phosphorylation by PKA is required for the control of hepatic lipid metabolism and glycemic control. J. Lipid Res. 62, 100016. doi:10.1194/jlr.RA120001126
Keenan S. N., Meex R. C., Lo J. C. Y., Ryan A., Nie S., Montgomery M. K., et al. (2019). Perilipin 5 deletion in hepatocytes remodels lipid metabolism and causes hepatic insulin resistance in mice. Diabetes 68 (3), 543–555. doi:10.2337/db18-0670
Khamoui A. V., Tokmina-Roszyk D., Rossiter H. B., Fields G. B., Visavadiya N. P. (2020). Hepatic proteome analysis reveals altered mitochondrial metabolism and suppressed acyl-CoA synthetase-1 in colon-26 tumor-induced cachexia. Physiol. Genomics 52 (5), 203–216. doi:10.1152/physiolgenomics.00124.2019
Kien B., Kolleritsch S., Kunowska N., Heier C., Chalhoub G., Tilp A., et al. (2022). Lipid droplet-mitochondria coupling via perilipin 5 augments respiratory capacity but is dispensable for FA oxidation. J. Lipid Res. 63 (3), 100172. doi:10.1016/j.jlr.2022.100172
Kim H. Y., Kwon W. Y., Kim Y. A., Oh Y. J., Yoo S. H., Lee M. H., et al. (2017). Polychlorinated biphenyls exposure-induced insulin resistance is mediated by lipid droplet enlargement through Fsp27. Arch. Toxicol. 91 (6), 2353–2363. doi:10.1007/s00204-016-1889-2
Kim J. T., Chen J., Zhou Y., Son M. J., Jeon D. H., Kwon J. W., et al. (2024). Cycloastragenol inhibits adipogenesis and fat accumulation in vitro and in vivo through activating Hedgehog signaling. Food Sci. Biotechnol. 33 (3), 711–720. doi:10.1007/s10068-023-01403-0
Kim M. H., Lee J. H., Lee J. S., Kim D. C., Yang J. W., An H. J., et al. (2023). Perilipin1 expression as a prognostic factor in patients with squamous cell carcinoma of the lung. Diagn. (Basel) 13 (22), 3475. doi:10.3390/diagnostics13223475
Krizanac M., Mass Sanchez P. B., Schröder S. K., Weiskirchen R., Asimakopoulos A. (2023). Lipid-independent regulation of PLIN5 via IL-6 through the JAK/STAT3 Axis in Hep3B cells. Int. J. Mol. Sci. 24 (8), 7219. doi:10.3390/ijms24087219
Kuramoto K., Okamura T., Yamaguchi T., Nakamura T. Y., Wakabayashi S., Morinaga H., et al. (2012). Perilipin 5, a lipid droplet-binding protein, protects heart from oxidative burden by sequestering fatty acid from excessive oxidation. J. Biol. Chem. 287 (28), 23852–23863. doi:10.1074/jbc.M111.328708
Langhi C., Marquart T. J., Allen R. M., Baldán A. (2014). Perilipin-5 is regulated by statins and controls triglyceride contents in the hepatocyte. J. Hepatol. 61 (2), 358–365. doi:10.1016/j.jhep.2014.04.009
Lennicke C., Cochemé H. M. (2021). Redox metabolism: ROS as specific molecular regulators of cell signaling and function. Mol. Cell 81 (18), 3691–3707. doi:10.1016/j.molcel.2021.08.018
Li B., Liu J., Xin X., Zhang L., Zhou J., Xia C., et al. (2021). MiR-34c promotes hepatic stellate cell activation and Liver Fibrogenesis by suppressing ACSL1 expression. Int. J. Med. Sci. 18 (3), 615–625. doi:10.7150/ijms.51589
Li H., Dong Y., Han C., Xia L., Zhang Y., Chen T., et al. (2023a). Suramin, an antiparasitic drug, stimulates adipocyte differentiation and promotes adipogenesis. Lipids Health Dis. 22 (1), 222. doi:10.1186/s12944-023-01980-3
Li J., Mihalcioiu M., Li L., Zakikhani M., Camirand A., Kremer R. (2018). Vitamin D prevents lipid accumulation in murine muscle through regulation of PPARγ and perilipin-2 expression. J. Steroid Biochem. Mol. Biol. 177, 116–124. doi:10.1016/j.jsbmb.2017.10.010
Li L. J., Ma J., Li S. B., Chen X., Zhang J. (2019). Vascular endothelial growth factor B inhibits lipid accumulation in C2C12 myotubes incubated with fatty acids. Growth factors. 37 (1-2), 76–84. doi:10.1080/08977194.2019.1626851
Li Q., Wang J., Wan Y., Chen D. (2016). Depletion of Rab32 decreases intracellular lipid accumulation and induces lipolysis through enhancing ATGL expression in hepatocytes. Biochem. Biophys. Res. Commun. 471 (4), 492–496. doi:10.1016/j.bbrc.2016.02.047
Li S., Hao L., Deng J., Zhang J., Hu X. (2023b). Coptidis rhizoma and evodiae fructus against lipid droplet deposition in nonalcoholic fatty liver disease-related liver cancer by AKT. Chem. Biol. Drug Des. 102 (4), 828–842. doi:10.1111/cbdd.14295
Li T., Li X., Meng H., Chen L., Meng F. (2020). ACSL1 affects triglyceride levels through the PPARγ pathway. Int. J. Med. Sci. 17 (6), 720–727. doi:10.7150/ijms.42248
Lima K. G., Schneider L. V. G., Rosa Garcia M. C., de Souza B. B., Pasqualotto Costa B., Antunes G. L., et al. (2020). Octyl gallate induces hepatic steatosis in HepG2 cells through the regulation of SREBP-1c and PPAR-gamma gene expression. Excli J. 19, 962–971. doi:10.17179/excli2020-2214
Liu W., Liu X., Liu Y., Ling T., Chen D., Otkur W., et al. (2022). PLIN2 promotes HCC cells proliferation by inhibiting the degradation of HIF1α. Exp. Cell Res. 418 (1), 113244. doi:10.1016/j.yexcr.2022.113244
Liu X., Wang S., Wu Z., Wang Z., Zheng Q., Li D. (2018). Deoxyschizandrin loaded liposomes on the suppression lipid accumulation in 3T3-L1 adipocytes. Molecules 23 (9), 2158. doi:10.3390/molecules23092158
Ma S. Y., Sun K. S., Zhang M., Zhou X., Zheng X. H., Tian S. Y., et al. (2020). Disruption of Plin5 degradation by CMA causes lipid homeostasis imbalance in NAFLD. Liver Int. 40 (10), 2427–2438. doi:10.1111/liv.14492
Mahdaviani K., Benador I. Y., Su S., Gharakhanian R. A., Stiles L., Trudeau K. M., et al. (2017). Mfn2 deletion in brown adipose tissue protects from insulin resistance and impairs thermogenesis. EMBO Rep. 18 (7), 1123–1138. doi:10.15252/embr.201643827
McGarry J. D., Leatherman G. F., Foster D. W. (1978). Carnitine palmitoyltransferase I. The site of inhibition of hepatic fatty acid oxidation by malonyl-CoA. J. Biol. Chem. 253 (12), 4128–4136. doi:10.1016/s0021-9258(17)34693-8
Michel V., Singh R. K., Bakovic M. (2011). The impact of choline availability on muscle lipid metabolism. Food Funct. 2 (1), 53–62. doi:10.1039/c0fo00069h
Moetlediwa M. T., Jack B. U., Mazibuko-Mbeje S. E., Pheiffer C., Titinchi S. J. J., Salifu E. Y., et al. (2024). Evaluating the therapeutic potential of Curcumin and synthetic Derivatives: a computational approach to anti-obesity treatments. Int. J. Mol. Sci. 25 (5), 2603. doi:10.3390/ijms25052603
Nguyen M. K., Kim C. Y., Kim J. M., Park B. O., Lee S., Park H., et al. (2016). Optogenetic oligomerization of Rab GTPases regulates intracellular membrane trafficking. Nat. Chem. Biol. 12 (6), 431–436. doi:10.1038/nchembio.2064
Nguyen T. B., Louie S. M., Daniele J. R., Tran Q., Dillin A., Zoncu R., et al. (2017). DGAT1-Dependent lipid droplet biogenesis protects mitochondrial function during starvation-induced autophagy. Dev. Cell 42 (1), 9–21. doi:10.1016/j.devcel.2017.06.003
Nimura S., Yamaguchi T., Ueda K., Kadokura K., Aiuchi T., Kato R., et al. (2015). Olanzapine promotes the accumulation of lipid droplets and the expression of multiple perilipins in human adipocytes. Biochem. Biophys. Res. Commun. 467 (4), 906–912. doi:10.1016/j.bbrc.2015.10.045
Ong S. B., Samangouei P., Kalkhoran S. B., Hausenloy D. J. (2015). The mitochondrial permeability transition pore and its role in myocardial ischemia reperfusion injury. J. Mol. Cell Cardiol. 78, 23–34. doi:10.1016/j.yjmcc.2014.11.005
Op den Kamp Y. J. M., Gemmink A., de Ligt M., Dautzenberg B., Kornips E., Jorgensen J. A., et al. (2022). Effects of SGLT2 inhibitor dapagliflozin in patients with type 2 diabetes on skeletal muscle cellular metabolism. Mol. Metab. 66, 101620. doi:10.1016/j.molmet.2022.101620
Ouyang Q., Chen Q., Ke S., Ding L., Yang X., Rong P., et al. (2023). Rab8a as a mitochondrial receptor for lipid droplets in skeletal muscle. Dev. Cell 58 (4), 289–305.e6. doi:10.1016/j.devcel.2023.01.007
Panajatovic M. V., Singh F., Krähenbühl S., Bouitbir J. (2020). Simvastatin impairs glucose homeostasis in mice depending on PGC-1α skeletal muscle expression. Biomedicines 8 (9), 351. doi:10.3390/biomedicines8090351
Panajatovic M. V., Singh F., Krähenbühl S., Bouitbir J. (2021). Effects of simvastatin on lipid metabolism in wild-type mice and mice with muscle PGC-1α overexpression. Int. J. Mol. Sci. 22 (9), 4950. doi:10.3390/ijms22094950
Ramos S. V., MacPherson R. E., Turnbull P. C., Bott K. N., LeBlanc P., Ward W. E., et al. (2014). Higher PLIN5 but not PLIN3 content in isolated skeletal muscle mitochondria following acute in vivo contraction in rat hindlimb. Physiol. Rep. 2 (10), e12154. doi:10.14814/phy2.12154
Sanvee G. M., Panajatovic M. V., Bouitbir J., Krähenbühl S. (2019). Mechanisms of insulin resistance by simvastatin in C2C12 myotubes and in mouse skeletal muscle. Biochem. Pharmacol. 164, 23–33. doi:10.1016/j.bcp.2019.02.025
Sato S., Suzuki J., Hirose M., Yamada M., Zenimaru Y., Nakaya T., et al. (2019). Cardiac overexpression of perilipin 2 induces atrial steatosis, connexin 43 remodeling, and atrial fibrillation in aged mice. Am. J. Physiol. Endocrinol. Metab. 317 (6), E1193-E1204–e1204. doi:10.1152/ajpendo.00227.2019
Schnell D. M., Walton R. G., Vekaria H. J., Sullivan P. G., Bollinger L. M., Peterson C. A., et al. (2019). Vitamin D produces a perilipin 2-dependent increase in mitochondrial function in C2C12 myotubes. J. Nutr. Biochem. 65, 83–92. doi:10.1016/j.jnutbio.2018.11.002
Seidell J. C., Halberstadt J. (2015). The global burden of obesity and the challenges of prevention. Ann. Nutr. Metab. 66 (Suppl. 2), 7–12. doi:10.1159/000375143
Song Y. F., Hogstrand C., Ling S. C., Chen G. H., Luo Z. (2020). Creb-Pgc1α pathway modulates the interaction between lipid droplets and mitochondria and influences high fat diet-induced changes of lipid metabolism in the liver and isolated hepatocytes of yellow catfish. J. Nutr. Biochem. 80, 108364. doi:10.1016/j.jnutbio.2020.108364
Stoldt S., Stephan T., Jans D. C., Brüser C., Lange F., Keller-Findeisen J., et al. (2019). Mic60 exhibits a coordinated clustered distribution along and across yeast and mammalian mitochondria. Proc. Natl. Acad. Sci. U. S. A. 116 (20), 9853–9858. doi:10.1073/pnas.1820364116
Stone S. J., Levin M. C., Zhou P., Han J., Walther T. C., Farese R. V. (2009). The endoplasmic reticulum enzyme DGAT2 is found in mitochondria-associated membranes and has a mitochondrial targeting signal that promotes its association with mitochondria. J. Biol. Chem. 284 (8), 5352–5361. doi:10.1074/jbc.M805768200
Su W., Wang Y., Jia X., Wu W., Li L., Tian X., et al. (2014). Comparative proteomic study reveals 17β-HSD13 as a pathogenic protein in nonalcoholic fatty liver disease. Proc. Natl. Acad. Sci. U. S. A. 111 (31), 11437–11442. doi:10.1073/pnas.1410741111
Suzuki K., Takahashi K., Nishimaki-Mogami T., Kagechika H., Yamamoto M., Itabe H. (2009). Docosahexaenoic acid induces adipose differentiation-related protein through activation of retinoid x receptor in human choriocarcinoma BeWo cells. Biol. Pharm. Bull. 32 (7), 1177–1182. doi:10.1248/bpb.32.1177
Sztalryd C., Brasaemle D. L. (2017). The perilipin family of lipid droplet proteins: gatekeepers of intracellular lipolysis. Biochim. Biophys. Acta Mol. Cell Biol. Lipids 1862 (10 Pt B), 1221–1232. doi:10.1016/j.bbalip.2017.07.009
Talari N. K., Mattam U., Meher N. K., Paripati A. K., Mahadev K., Krishnamoorthy T., et al. (2023). Lipid-droplet associated mitochondria promote fatty-acid oxidation through a distinct bioenergetic pattern in male Wistar rats. Nat. Commun. 14 (1), 766. doi:10.1038/s41467-023-36432-0
Tan L., Song A., Ren L., Wang C., Song G. (2020). Effect of pioglitazone on skeletal muscle lipid deposition in the insulin resistance rat model induced by high fructose diet under AMPK signaling pathway. Saudi J. Biol. Sci. 27 (5), 1317–1323. doi:10.1016/j.sjbs.2020.03.014
Tan Y., Jin Y., Wang Q., Huang J., Wu X., Ren Z. (2019). Perilipin 5 protects against cellular oxidative stress by enhancing mitochondrial function in HepG2 cells. Cells 8 (10), 1241. doi:10.3390/cells8101241
Tarnopolsky M. A., Rennie C. D., Robertshaw H. A., Fedak-Tarnopolsky S. N., Devries M. C., Hamadeh M. J. (2007). Influence of endurance exercise training and sex on intramyocellular lipid and mitochondrial ultrastructure, substrate use, and mitochondrial enzyme activity. Am. J. Physiol. Regul. Integr. Comp. Physiol. 292 (3), R1271–R1278. doi:10.1152/ajpregu.00472.2006
Thiam A. R., Ikonen E. (2021). Lipid droplet nucleation. Trends Cell Biol. 31 (2), 108–118. doi:10.1016/j.tcb.2020.11.006
Tilg H., Adolph T. E., Dudek M., Knolle P. (2021). Non-alcoholic fatty liver disease: the interplay between metabolism, microbes and immunity. Nat. Metab. 3 (12), 1596–1607. doi:10.1038/s42255-021-00501-9
Toledo F. G. S., Johannsen D. L., Covington J. D., Bajpeyi S., Goodpaster B., Conley K. E., et al. (2018). Impact of prolonged overfeeding on skeletal muscle mitochondria in healthy individuals. Diabetologia 61 (2), 466–475. doi:10.1007/s00125-017-4496-8
Tsubai T., Yoshimi A., Hamada Y., Nakao M., Arima H., Oiso Y., et al. (2017). Effects of clozapine on adipokine secretions/productions and lipid droplets in 3T3-L1 adipocytes. J. Pharmacol. Sci. 133 (2), 79–87. doi:10.1016/j.jphs.2017.01.004
Ueno M., Suzuki J., Hirose M., Sato S., Imagawa M., Zenimaru Y., et al. (2017). Cardiac overexpression of perilipin 2 induces dynamic steatosis: prevention by hormone-sensitive lipase. Am. J. Physiol. Endocrinol. Metab. 313 (6), E699-E709–e709. doi:10.1152/ajpendo.00098.2017
Valm A. M., Cohen S., Legant W. R., Melunis J., Hershberg U., Wait E., et al. (2017). Applying systems-level spectral imaging and analysis to reveal the organelle interactome. Nature 546 (7656), 162–167. doi:10.1038/nature22369
Walther T. C., Chung J., Farese R. V. (2017). Lipid droplet biogenesis. Annu. Rev. Cell Dev. Biol. 33, 491–510. doi:10.1146/annurev-cellbio-100616-060608
Wang C., Yuan Y., Wu J., Zhao Y., Gao X., Chen Y., et al. (2019). Plin5 deficiency exacerbates pressure overload-induced cardiac hypertrophy and heart failure by enhancing myocardial fatty acid oxidation and oxidative stress. Free Radic. Biol. Med. 141, 372–382. doi:10.1016/j.freeradbiomed.2019.07.006
Wang H., Becuwe M., Housden B. E., Chitraju C., Porras A. J., Graham M. M., et al. (2016). Seipin is required for converting nascent to mature lipid droplets. Elife 5, e16582. doi:10.7554/eLife.16582
Wang H., Sreenivasan U., Hu H., Saladino A., Polster B. M., Lund L. M., et al. (2011). Perilipin 5, a lipid droplet-associated protein, provides physical and metabolic linkage to mitochondria. J. Lipid Res. 52 (12), 2159–2168. doi:10.1194/jlr.M017939
Wang S., Zhang B., Mauck J., Loor J. J., Fan W., Tian Y., et al. (2024). Diacylglycerol O-acyltransferase (DGAT) isoforms play a role in peridroplet mitochondrial fatty acid metabolism in bovine liver. J. Dairy Sci. doi:10.3168/jds.2024-24738
Wei F., Wang Y., Yao J., Mei L., Huang X., Kong H., et al. (2024). ZDHHC7-mediated S-palmitoylation of ATG16L1 facilitates LC3 lipidation and autophagosome formation. Autophagy, 1–19. doi:10.1080/15548627.2024.2386915
Wei X., Li L., Zhao J., Huo Y., Hu X., Lu J., et al. (2023). BAP31 depletion inhibited adipogenesis, repressed lipolysis and promoted lipid droplets abnormal growth via attenuating Perilipin1 proteasomal degradation. Int. J. Biol. Sci. 19 (6), 1713–1730. doi:10.7150/ijbs.82178
Wende A. R., Brahma M. K., McGinnis G. R., Young M. E. (2017). Metabolic origins of heart failure. JACC Basic Transl. Sci. 2 (3), 297–310. doi:10.1016/j.jacbts.2016.11.009
Wolins N. E., Quaynor B. K., Skinner J. R., Tzekov A., Croce M. A., Gropler M. C., et al. (2006). OXPAT/PAT-1 is a PPAR-induced lipid droplet protein that promotes fatty acid utilization. Diabetes 55 (12), 3418–3428. doi:10.2337/db06-0399
Wolins N. E., Skinner J. R., Schoenfish M. J., Tzekov A., Bensch K. G., Bickel P. E. (2003). Adipocyte protein S3-12 coats nascent lipid droplets. J. Biol. Chem. 278 (39), 37713–37721. doi:10.1074/jbc.M304025200
Xia S., Wang Z., Chen L., Zhou Y., Li Y., Wang S., et al. (2021). Dihydroartemisinin regulates lipid droplet metabolism in hepatic stellate cells by inhibiting lncRNA-H19-induced AMPK signal. Biochem. Pharmacol. 192, 114730. doi:10.1016/j.bcp.2021.114730
Xiang J., Qin L., Zhong J., Xia N., Liang Y. (2023). GLP-1RA liraglutide and semaglutide improves obesity-induced muscle atrophy via SIRT1 pathway. Diabetes Metab. Syndr. Obes. 16, 2433–2446. doi:10.2147/dmso.S425642
Xie X., Liu Y., Yang Q., Ma X., Lu Y., Hu Y., et al. (2024). Adipose triglyceride lipase-mediated adipocyte lipolysis exacerbates acute pancreatitis severity in mouse models and patients. Am. J. Pathol. 3, 1494–1510. doi:10.1016/j.ajpath.2024.03.014
Yang M., Zhao L., Gao P., Zhu X., Han Y., Chen X., et al. (2019). DsbA-L ameliorates high glucose induced tubular damage through maintaining MAM integrity. EBioMedicine 43, 607–619. doi:10.1016/j.ebiom.2019.04.044
Yao P., Zhang Z., Liu H., Jiang P., Li W., Du W. (2023). p53 protects against alcoholic fatty liver disease via ALDH2 inhibition. Embo J. 42 (8), e112304. doi:10.15252/embj.2022112304
Yen C. H., Chang H. S., Yang T. H., Wang S. F., Wu H. C., Chen Y. C., et al. (2018). High-content screening of a Taiwanese indigenous plant extract library identifies Syzygium simile leaf extract as an inhibitor of fatty acid uptake. Int. J. Mol. Sci. 19 (7), 2130. doi:10.3390/ijms19072130
Young P. A., Senkal C. E., Suchanek A. L., Grevengoed T. J., Lin D. D., Zhao L., et al. (2018). Long-chain acyl-CoA synthetase 1 interacts with key proteins that activate and direct fatty acids into niche hepatic pathways. J. Biol. Chem. 293 (43), 16724–16740. doi:10.1074/jbc.RA118.004049
Younossi Z. M., Koenig A. B., Abdelatif D., Fazel Y., Henry L., Wymer M. (2016). Global epidemiology of nonalcoholic fatty liver disease-Meta-analytic assessment of prevalence, incidence, and outcomes. Hepatology 64 (1), 73–84. doi:10.1002/hep.28431
Yu J., Zhang S., Cui L., Wang W., Na H., Zhu X., et al. (2015). Lipid droplet remodeling and interaction with mitochondria in mouse brown adipose tissue during cold treatment. Biochim. Biophys. Acta 1853 (5), 918–928. doi:10.1016/j.bbamcr.2015.01.020
Yu W. Q., Yin F., Shen N., Lin P., Xia B., Li Y. J., et al. (2021). Polysaccharide CM1 from Cordyceps militaris hinders adipocyte differentiation and alleviates hyperlipidemia in LDLR((+/-)) hamsters. Lipids Health Dis. 20 (1), 178. doi:10.1186/s12944-021-01606-6
Yuan X., Wei G., You Y., Huang Y., Lee H. J., Dong M., et al. (2017). Rutin ameliorates obesity through brown fat activation. Faseb J. 31 (1), 333–345. doi:10.1096/fj.201600459RR
Yuan Z., Lu X., Lei F., Sun H., Jiang J., Xing D., et al. (2023). Novel effect of p-coumaric acid on hepatic lipolysis: inhibition of hepatic lipid-droplets. Molecules 28 (12), 4641. doi:10.3390/molecules28124641
Zadoorian A., Du X., Yang H. (2023). Lipid droplet biogenesis and functions in health and disease. Nat. Rev. Endocrinol. 19 (8), 443–459. doi:10.1038/s41574-023-00845-0
Zhang E., Yin S., Zhao C., Fan L., Hu H. (2020a). Involvement of activation of PLIN5-Sirt1 axis in protective effect of glycycoumarin on hepatic lipotoxicity. Biochem. Biophys. Res. Commun. 528 (1), 7–13. doi:10.1016/j.bbrc.2020.05.072
Zhang W., An R., Li Q., Sun L., Lai X., Chen R., et al. (2020b). Theaflavin TF3 relieves hepatocyte lipid deposition through activating an AMPK signaling pathway by targeting plasma kallikrein. J. Agric. Food Chem. 68 (9), 2673–2683. doi:10.1021/acs.jafc.0c00148
Zhao H., Han T., Hong X., Sun D. (2017). Adipose differentiation-related protein knockdown inhibits vascular smooth muscle cell proliferation and migration and attenuates neointima formation. Mol. Med. Rep. 16 (3), 3079–3086. doi:10.3892/mmr.2017.6997
Zheng P., Xie Z., Yuan Y., Sui W., Wang C., Gao X., et al. (2017). Plin5 alleviates myocardial ischaemia/reperfusion injury by reducing oxidative stress through inhibiting the lipolysis of lipid droplets. Sci. Rep. 7, 42574. doi:10.1038/srep42574
Zheng Q. W., Ding X. F., Cao H. J., Ni Q. Z., Zhu B., Ma N., et al. (2021). Ochratoxin A induces steatosis via pparγ-CD36 Axis. Toxins (Basel) 13 (11), 802. doi:10.3390/toxins13110802
Zhong W., Fan B., Cong H., Wang T., Gu J. (2019). Oleic acid-induced perilipin 5 expression and lipid droplets formation are regulated by the PI3K/PPARα pathway in HepG2 cells. Appl. Physiol. Nutr. Metab. 44 (8), 840–848. doi:10.1139/apnm-2018-0729
Zhou H., Du W., Li Y., Shi C., Hu N., Ma S., et al. (2018a). RETRACTED: effects of melatonin on fatty liver disease: the role of NR4A1/DNA-PKcs/p53 pathway, mitochondrial fission, and mitophagy. J. Pineal Res. 64 (1). doi:10.1111/jpi.12450
Zhou R., Yi L., Ye X., Zeng X., Liu K., Qin Y., et al. (2018b). Resveratrol ameliorates lipid droplet accumulation in liver through a SIRT1/ATF6-dependent mechanism. Cell Physiol. Biochem. 51 (5), 2397–2420. doi:10.1159/000495898
Zhou X. T., Zhu A. Q., Li X. M., Sun L. Y., Yan J. G., Luo N., et al. (2024). Mulberry and Hippophae-based solid beverage promotes weight loss in rats by antagonizing white adipose tissue PPARγ and FGFR1 signaling. Front. Endocrinol. (Lausanne) 15, 1344262. doi:10.3389/fendo.2024.1344262
Keywords: lipid droplets, mitochondria, peridroplet mitochondria, obesity, metabolic syndrome, pharmacotherapy
Citation: Zhang C, Zheng M, Bai R, Chen J, Yang H and Luo G (2024) Molecular mechanisms of lipid droplets-mitochondria coupling in obesity and metabolic syndrome: insights and pharmacological implications. Front. Physiol. 15:1491815. doi: 10.3389/fphys.2024.1491815
Received: 05 September 2024; Accepted: 29 October 2024;
Published: 11 November 2024.
Edited by:
Geraldine M. Dowling Sfhea, Atlantic Technological University, IrelandReviewed by:
Revathi Sekar, Helmholtz Association of German Research Centres (HZ), GermanyCopyright © 2024 Zhang, Zheng, Bai, Chen, Yang and Luo. This is an open-access article distributed under the terms of the Creative Commons Attribution License (CC BY). The use, distribution or reproduction in other forums is permitted, provided the original author(s) and the copyright owner(s) are credited and that the original publication in this journal is cited, in accordance with accepted academic practice. No use, distribution or reproduction is permitted which does not comply with these terms.
*Correspondence: Hong Yang, eWFuZ2hvbmdAY2R1dGNtLmVkdS5jbg==; Gan Luo, bHVvZ2FuaGRAMTI2LmNvbQ==
†These authors contributed equally to this work and should be considered co-first authors
Disclaimer: All claims expressed in this article are solely those of the authors and do not necessarily represent those of their affiliated organizations, or those of the publisher, the editors and the reviewers. Any product that may be evaluated in this article or claim that may be made by its manufacturer is not guaranteed or endorsed by the publisher.
Research integrity at Frontiers
Learn more about the work of our research integrity team to safeguard the quality of each article we publish.