- Institute of Cardiovascular Science, University College London, London, United Kingdom
Near-infrared spectroscopy (NIRS) is an optical technique that can be used to non-invasively interrogate haemodynamic changes within skeletal muscle. It can be combined with a short (3–5 min) arterial cuff-occlusion to quantify post-occlusive reactive hyperaemia (PORH). This technique has utility in tracking changes in vascular health in relation to exercise, disease progression or treatment efficacy. However, methods for assessing PORH vary widely and there is little consensus on methodological approaches such as sampling frequency, correction for adipose tissue or the analysis endpoints. The purpose of this review was to: (1) summarise recent advances; (2) compare different methodological approaches and (3) identify current knowledge gaps and future objectives for use of NIRS for vascular assessment. We propose key areas for future work, including optimising occlusion duration and comparing methods of correction for the ischemic stimulus, standardising methods for adjustment of adipose tissue thickness, cross-device comparisons and establishing a standard for minimum sampling rate. Comparisons with alternative methods of capturing PORH or upstream vasodilatory responses would be valuable. Addressing these methodological considerations will aid our understanding of this useful, non-invasive tool for characterising PORH within skeletal muscle and facilitate interpretation of results across studies.
1 Introduction
Post-occlusive reactive hyperaemia (PORH) refers to the increase in blood flow following brief ischemia induced by an arterial occlusion and is an indicator of vasodilatory capacity. Blunted PORH indicates vascular dysfunction and is a predictor of cardiovascular morbidity and mortality (Ishibashi et al., 2006; Paine et al., 2016; Anderson et al., 2011). PORH also provides the stimulus for flow-mediated dilation (FMD) in conduit arteries (Corretti et al., 2002) which also predicts future cardiovascular outcomes (Inaba et al., 2010).
Near-infrared spectroscopy (NIRS) is a non-invasive optical method that measures oxygenated and deoxygenated haemoglobin and myoglobin (referred to as oxy [heme] and deoxy [heme] here, in line with a recent call for standardization but also widely referred to as oxy [Hb + Mb] or deoxy [Hb + Mb]) in small blood vessels and myocytes (Scholkmann et al., 2014). In skeletal muscle, NIRS has been used to detect differences in PORH in peripheral arterial disease (Jarm et al., 2003; Kooijman et al., 1997; Kragelj et al., 2001), coronary heart disease (Monthé-Sagan et al., 2016; Gayda et al., 2015), metabolic syndrome (Gayda et al., 2015), sepsis (Creteur et al., 2007; Mayeur et al., 2011), acute respiratory disorders (Orbegozo Cortés et al., 2016), in smokers versus non-smokers (Siafaka et al., 2007; Zamparini et al., 2015), and between different age groups (Rosenberry et al., 2018; de Oliveira et al., 2020). However, NIRS is relatively under-exploited compared to other tools, such as venous occlusion plethysmography (VOP) or peripheral arterial tonometry (PAT), and methods can vary considerably between studies.
This narrative review aims to describe the application of NIRS for assessment of PORH in skeletal muscle and summarise methods from publications spanning the last 25 years. Publications were identified by screening online databases, MEDLINE and PubMed Central, using search terms ‘post-occlusive reactive hyperaemia’ and ‘near-infrared spectroscopy’ and ‘muscle’. Additional publications were identified through reference lists within the screened publications. We did not aim to undertake a systematic review of the literature or provide an exhaustive list of studies applying NIRS to capture PORH in this mini review. Studies were used as examples to highlight key points discussed. To demonstrate the range of different methods applied, we extracted information about study design, the type of NIRS device used, the signal processing methods and analysis endpoints from the example studies. We did not formally assess the quality of the studies included here. We compared NIRS with alternative methods for assessing PORH and aimed to identify targets for the advancement of NIRS as a vascular assessment tool. A comprehensive comparison of the different methods for capturing PORH is available elsewhere (Rosenberry and Nelson, 2020). A general review of NIRS application to skeletal muscle (Barstow, 2019a) and technical reviews of NIRS technology have also been published previously (Scholkmann et al., 2014).
2 PORH assessment using NIRS, data processing and analysis endpoints
NIRS devices are typically applied to the distal portion of a limb to measure PORH. The mechanism of PORH is fairly well understood (Rosenberry and Nelson, 2020). When the pressure inside the small arteries declines, wall circumferential stress drops, withdrawing myogenic tone and causing vasodilation (the Bayliss effect) (Bayliss, 1902). During ischemia, various factors also contribute to vasodilation, including adenosine (Cheng et al., 2000), H+ ions (Granger and Shepherd, 1973), prostaglandins (Engelke et al., 1996), K+ ions (Shimoda and Polak, 2011), bradykinin (Hornig et al., 1997), endothelium derived hyperpolarisation factor and nitric oxide (NO) (Shu et al., 2015), although the role of NO is debated (Wong et al., 2003). Hyperaemia occurs immediately following cuff release and an increase in shear stress in conduit vessels further promotes re-perfusion via FMD Corretti et al., 2002.
Haemodynamic changes are observed in NIRS signals as rapid increases in oxy [heme], total [heme] (oxy [heme]+ deoxy [heme]) or tissue saturation/oxygenation index (referred to here as StO2 but also commonly referred to as TSI or TOI%). NIRS analysis endpoints can be derived that characterize the changes in these signals, most commonly, they include: reperfusion (resaturation) rate (Kooijman et al., 1997; Creteur et al., 2007; Mayeur et al., 2011; Rosenberry et al., 2018; Cheatle et al., 1991; Cheng et al., 2004; Hammer et al., 1985; Lacroix et al., 2012; McLay et al., 2016a; McLay et al., 2016b), peak response as an absolute tissue saturation value (maximum StO2) or peak value of oxy [heme], the magnitude of change (Δ from baseline value to peak value of StO2 or oxy [heme]) or a percentage change (Kragelj et al., 2001; Creteur et al., 2007; Rosenberry et al., 2018; Cheng et al., 2004; Hammer et al., 1985; McLay et al., 2016a; McLay et al., 2016b; Chung et al., 2018), time to peak reperfusion following cuff-release or a given percentage of that time (50%, 95% of peak) and the area under the reperfusion curve (AUC reperfusion) (Jones et al., 2022) (Figure 1; Table 1). Desaturation rate during the occlusion or magnitude of desaturation (Δ StO2 between rest and minimum StO2 at the end of the occlusion) are frequently reported and response beyond the peak PORH (return of signals to resting values/baseline) has been described in some studies (Table 1). The pattern of the reperfusion response and recovery beyond peak have also been quantified (Bopp et al., 2014; Bopp et al., 2011). Patterns of reperfusion and recovery have been modelled using various functions (Bopp et al., 2014; Bopp et al., 2011). In a study where three functions (Gompertz, logistic and exponential) were fit to the same PORH data across 20 healthy subjects, the authors found that the Gompertz function provided the best fit to the oxy [heme] reperfusion data (Bopp et al., 2011). Studies do not typically present all derivable endpoints and terminology for endpoints is variable. Consensus on a set of standard endpoints and on the nomenclature would be beneficial. A limitation is the assumption that the majority of the change in signal is due to changes in blood flow; it is possible that the changes observed are influenced by alterations in oxygen consumption rate in the tissue. Using the total [heme] or StO2 signals addresses this limitation to some degree as the changes in deoxy [heme] are incorporated into the signal.
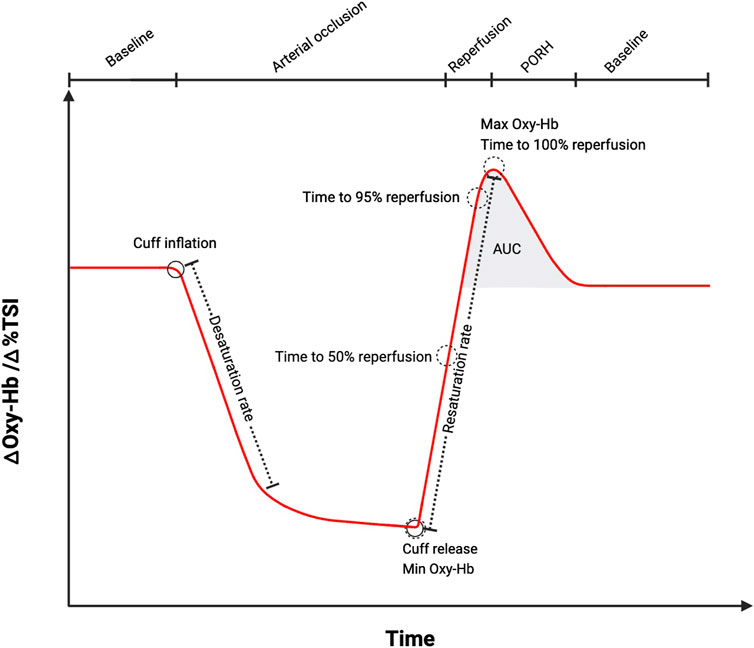
Figure 1. A schematic diagram illustrating change in oxygenated haemoglobin (oxy-Hb, oxy [heme]) or tissue saturation index (TSI, StO2) during ischemia and post-occlusive reactive hyperaemia (PORH) measured using near-infrared spectroscopy (NIRS). The most common endpoints that can be extracted from the NIRS signal are labelled. AUC (area under the curve). Schematic created using BioRender.com software, based on measurements performed by the authors and schematics previously presented (Rosenberry and Nelson, 2020).
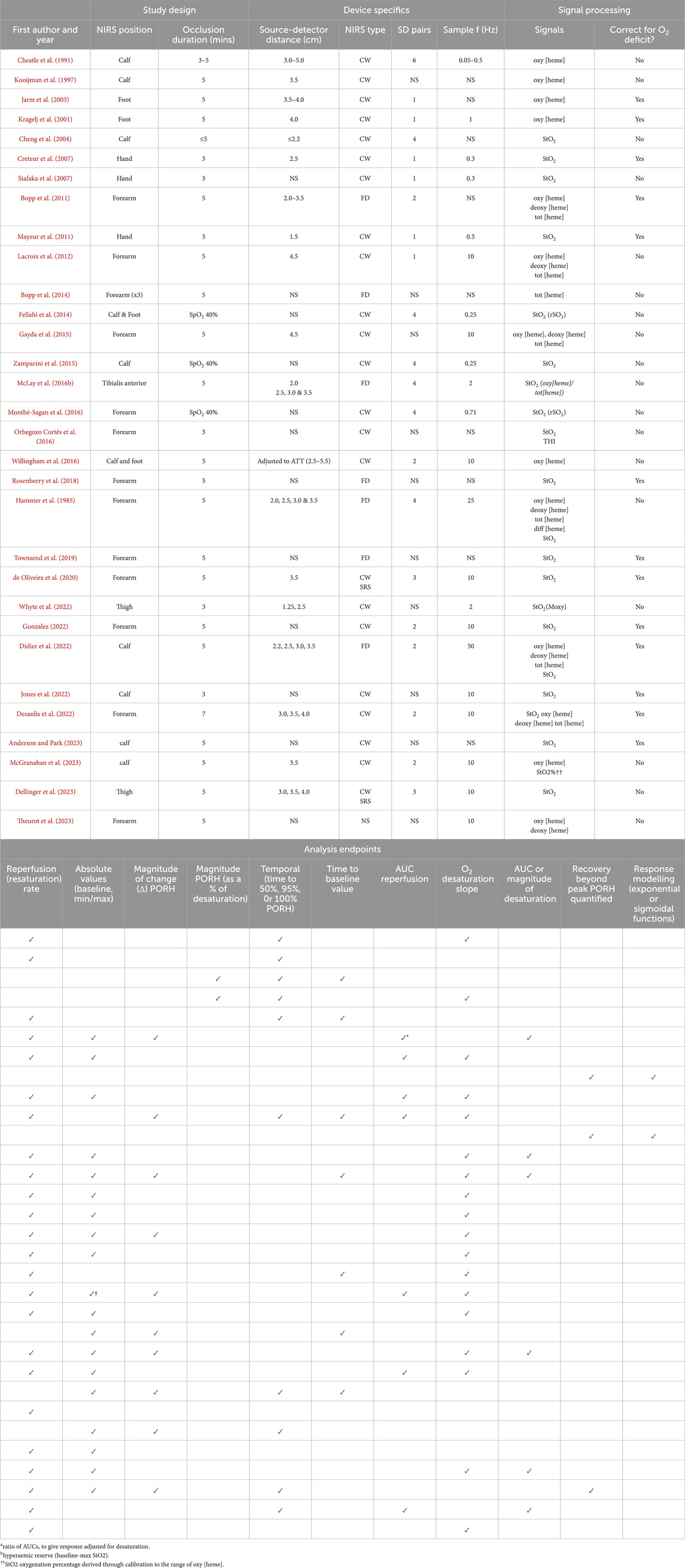
Table 1. An overview of the methodological aspects of key studies from the past ∼30 years including a list of the endpoints derived from each as well as the signals analysed to retrieve endpoints. A NIRS position indicated as ‘calf’ refers to either the lateral or medial gastrocnemius. A NIRS position indicated as ‘forearm’ refers to either the flexor digitorum profundus or brachioradialis, flexor carpi radialis, flexor. Signals indicates the signals used for the processing (not a report of all the signals that are possible to derive from the specified device used) and nomenclature is as reported in the publication. AUC (area under curve), ATT (adipose tissue thickness), CW (continuous wave), deoxy [heme] (deoxygenated haemoglobin/myoglobin concentration), FD (frequency domain), NS (not specified), oxy [heme] (oxygenated haemoglobin/myoglobin concentration), SD (source-detector), SRS (spatially resolved spectroscopy), tot [heme] index (THI).
When conditions where there is vascular dysfunction, such as PAD, are compared to healthy participants, NIRS endpoints tend to be impaired (e.g., slower reperfusion rates, prolonged recovery times and smaller magnitude of change (Δ minimum to maximum during PORH)) (Kooijman et al., 1997; Kragelj et al., 2001; Creteur et al., 2007; Cheatle et al., 1991). It is unlikely that specific physiological mechanisms underpinning PORH (described above) can be inferred from specific analytical endpoints. However, combining NIRS with additional measurement methods in the future may permit these different aspects to be delineated to some extent.
3 Study design
3.1 NIRS measurement site
Theoretically, any portion of a limb or tissue where an arterial occlusion can be applied safely can be a measurement site. Typical measurement sites are: the forearm (brachioradialis or flexor digitorum profundus), calf (gastrocnemius or tibilias), hand (thenar eminence) and foot (Table 1). Consideration of the research question and participant population is important prior to muscle group selection. The thenar eminence has been recommended due to low levels of overlying subcutaneous adipose tissue thickness (ATT), minimal oedema, and good reproducibility in healthy subjects (Mayeur et al., 2011; Gómez et al., 2009). If an exercise intervention is being tested, a muscle group which is suitable for training should be chosen. Similarly, if the participant population is likely to have excess adiposity, a site with less ATT is preferable.
Different anatomical sites may differ in terms of metabolic and vascular properties, muscle thickness, fibre type proportion and overlying ATT. These differences make comparisons between studies challenging. PORH has been compared across different measurement sites in the same individuals by Gomez et al. (Gómez et al., 2009). They found that baseline oxy [heme] and the magnitude of oxy [heme] desaturation and oxy [heme] re-saturation were lower in the forearm compared to the hand; however, we do not know the extent to which these reflect differences in microvascular behaviour or differences in overlying tissue properties that impact NIRS measurements. Future work on these questions would be useful. Use of multi-channel devices at different points along the same muscle during PORH could permit intra-muscle similarities/differences to be examined. Consideration of distance between measurement site and the cuff occlusion site may also be important, but we are unaware of any published work that has explored this.
Participants who undertake PORH measurements often find the occlusions uncomfortable, and at times, painful. Lacroix et al. reported that the forearm occlusion was less uncomfortable than lower limb occlusion (Lacroix et al., 2012) but there has been limited work exploring the effect of discomfort on results; if investigators frequently find it necessary to exclude participants who are unable to complete the pre-specified occlusion duration, this may introduce selection bias into presented results. Future work exploring participant discomfort during arterial occlusions, perhaps via incorporation of pain scale questionnaires, could provide valuable practical insights.
3.2 Measuring adipose tissue thickness and adjusting source-detector distance
If ATT is greater than half the distance between the NIRS source and detector, little or no signal from skeletal muscle will be detected (Van Beekvelt M. et al., 2001). Most NIRS devices have fixed distances of 2–4 cm, but some have multiple light sources or detectors fixed in series (multi-distance devices). Using longer source-detector distances allows greater sampling depth but at the cost of reduced signal.
Despite being a well-recognised limitation, ATT is not routinely measured or corrected for in studies that use NIRS. Measurement of subcutaneous ATT overlying the muscle of interest can be done using callipers or an appropriate ultrasound device. Out of the studies in Table 1, three included a correction method for ATT, one adjusted source-detector distance to at least twice that of an individual’s ATT (41), and two used statistical adjustment to account for ATT (36, 37). In a study where total [heme] (frequency domain NIRS) and ATT were measured across different muscles, results suggested ATT correction equations for oxy [heme] and deoxy [heme] may be specific to the muscle of interest (Craig et al., 2017). In work where the reperfusion response has been modelled using sigmoidal or exponential functions, Bopp et al. found that the calculated time constants representing the reperfusion curve (τ1) or the return to baseline curve (τ2) were far less affected by ATT than the parameters reflecting the magnitude of change (Bopp et al., 2014; Bopp et al., 2011). This suggests that modelling the kinetics of the PORH may be a useful way to circumvent the impact of ATT on the analysis endpoints. Further studies examining the effect of ATT on quantification of PORH would be useful.
3.3 Occlusion duration
The magnitude of the ischemic stimulus is an important determinant of PORH. The most common occlusion durations reported are three or 5 min. A limitation of using pre-set occlusion durations is that rates of deoxygenation may vary considerably across individuals. In a recent model, the StO2 deficit was directly associated with the reoxygenation rate; as oxygen deficit increased, reoxygenation rate increased (Anderson and Park, 2023). McLay et al. (McLay et al., 2016a) found that in all of their participants, a desaturation plateau was reached before 5-min of occlusion, but 3-min occlusions in other studies have resulted in varying desaturation levels (Mayeur et al., 2011). A major advantage of NIRS, compared to other techniques, is that changes in tissue saturation can be recorded during the occlusion and a rate of desaturation (oxygen consumption rate) can be calculated. This allows users to standardize the ischemic stimulus or account for it in a post-processing step. Rosenberry et al. (Rosenberry et al., 2018) highlighted the potential importance of differences in desaturation. When they adjusted the occlusion duration in young and old individuals to standardize the level of desaturation, they found that age-related differences in PORH were attenuated. Some studies present the amplitude of oxy [heme] change as a percentage of the occlusion stimulus to circumvent this problem (Jarm et al., 2003; Kragelj et al., 2001; McGranahan et al., 2023). Adjusting occlusion duration so that a pre-specified reduction in oxy [heme] or StO2 is achieved is an alternative and several studies have employed this approach (Monthé-Sagan et al., 2016; Mayeur et al., 2011; Zamparini et al., 2015; Fellahi et al., 2014). Taking baseline StO2 value into account may also be beneficial, since this can differ between participants due to varying basal metabolic rates and vascular architecture at the measurement site.
4 Technical considerations
4.1 NIRS devices and sampling frequency
Broadly speaking, a NIRS device works by emitting a known intensity of NIR-light (650–950 nm) at two or more wavelengths into tissue, measuring the intensity of returning light at each wavelength. Based on differential absorption patterns of each wavelength by oxy [heme] and deoxy [heme], the device estimates the concentration (or concentration change) of each chromophore (Jones et al., 2022). This simple technology has been developed into several different device types: continuous wave (CW), frequency-domain (FD) and time domain (TD) devices, reviewed elsewhere (Barstow, 2019b). Thus far, only FD and CW devices appear to have been employed for PORH measurements (Table 1). CW devices are simple and cost-effective but are limited in only providing information about relative chromophore concentrations. During PORH, as we are interested in the response to ischemia, this is not a major limitation. FD devices can account for light scattering and therefore measure absolute concentrations. Direct comparisons between different types of NIRS devices during PORH would be valuable.
Multi-distance CW-NIRS devices can use spatially resolved spectroscopy (SRS) to calculate a StO2 (Artinis Medical Systems TN, 2022). SRS addresses some of the problems relating to variations in ATT, and StO2 is frequently used for PORH. There are some variations in the design of devices applying SRS. Some devices use multiple light sources and a single detector while others use a single light source with multiple detectors to quantify linear change of light attenuation against distance (Suzuki et al., 1999). We have not found studies comparing different light source-detector arrangements for PORH. A source of confusion is that the term ‘StO2’ is used synonymously with ‘TSI’ (tissue saturation index), ‘TOI’ (tissue oxygen index) and ‘SmO2’ (muscle tissue oxygen saturation) in the literature. Also, some authors calculate StO2 as oxy-[heme]/(oxy [heme] +deoxy [heme])*100% in the absence of SRS. Consensus on use of these terms has previously been called for and would be beneficial.
NIRS devices typically have high temporal resolution (>1 Hz), although sampling rates as low as 0.25 Hz have been applied successfully (Mayeur et al., 2011; Zamparini et al., 2015; Cheatle et al., 1991; Fellahi et al., 2014). In contrast, VOP is limited to one measurement every 5–10 s. Higher sampling frequency is beneficial for capturing changes that occur immediately on cuff release and allows identification of pulsatile changes in the oxy-Hb signal at rest. Reciprocal changes in oxy [heme] and deoxy [heme] signals and absence of pulsatility are useful criteria to indicate successful arterial occlusion.
4.2 Myoglobin contribution to the NIRS signals during PORH
The absorption spectra of oxy- and deoxy-myoglobin (Mb) within myocytes and circulating oxy- and deoxy [heme], are very similar. It has been estimated that, in contracting muscles, the Mb contribution to the signal ranges from 20% to 80% (Lai et al., 2009; Davis and Barstow, 2013). The possibility that the oxy [heme] contribution is not constant, changing with varying degrees of tissue hypoxia, has implications for PORH. This was recently explored through combining an experimental canine model with a computational model of muscle O2 transport and utilization (Koirala et al., 2023). This analysis indicated that Mb contributed ∼20–30% of NIRS signals at rest, but when O2 delivery was reduced, the proportion of deoxy-[heme] signal due to Mb increased to 50% and the contribution to the oxy [heme] signal increased from 0% to 30%. This suggests analysis endpoints derived using only the oxy [heme] NIRS signal would be influenced by changing Mb contributions throughout desaturation or resaturation. Considering changes in the total [heme] signal or a StO2 signal would address this problem to some extent as it is unlikely that the total concentration of myocyte Mb changes during the ischemia-reperfusion manoeuvre.
Another consideration is that type I (slow twitch) muscle fibres typically contain higher concentrations of Mb than type II (fast twitch) muscle fibres (Bekedam et al., 2009). Variation exists in the typical fibre-type proportion between muscles; for example, the soleus muscle is known to be comprised of mostly type I fibres while triceps contain mostly type II fibres (Talbot and Maves, 2016). This could be important when comparisons are made across different muscle groups. However, it is also known that muscle fibre type can be altered with training, which may pose difficulties in comparing untrained with trained populations or pre-versus post-exercise intervention (Talbot and Maves, 2016). Recent work in elite cyclists suggests that they may overall have reduced Mb concentration compared to active controls (Jacobs et al., 2023). Furthermore, it has been shown that Mb concentration is greater in older compared with young adults (Möller and Sylven, 1981); this has implications for comparisons of PORH by age.
4.3 Reproducibility of PORH parameters measured by NIRS
Several studies have reported the reproducibility of NIRS PORH(13, 32, 34, 41, 44, 56). Test-re-test reproducibility for temporal, absolute range and reperfusion recovery slope parameters ranges from adequate to excellent (intra-class correlation coefficients (ICC) between 0.5 and 0.9) in the leg (McLay et al., 2016b; Willingham et al., 2016; McGranahan et al., 2023) and adequate to good in the arm (ICC 0.6–0.84) (Lacroix et al., 2012). Time to peak hyperaemia was less reproducible than time to 95% peak, since the duration of the last 5% of reperfusion can vary substantially (Willingham et al., 2016). Mclay et al. directly compared repeatability of PORH by NIRS and FMD in the leg and found better intra- and inter-day reproducibility for the NIRS measurements (McLay et al., 2016b). These studies used 5-min occlusion durations and did not adjust for oxygen deficits (desaturation) during the occlusion. Mayeur et al. (Mayeur et al., 2011) reported better reproducibility when using a 40% desaturation target versus a set 3-min duration in the forearm (coefficient of variation 5.9% versus 10.5%). Further studies exploring reproducibility of NIRS-measured PORH following different occlusion durations will be valuable in establishing the precision of the technique.
As NIRS technology develops, it will be important to assess repeatability of newer devices for assessment of PORH. For example, use of StO2% measured by SRS in place of raw oxy [heme] and deoxy [heme] data for PORH is becoming more widespread and it will be important to establish the reproducibility of StO2% in quantifying PORH. Assessing the precision of more advanced modelling techniques for quantifying PORH, such as methods described by Bopp et al. (Bopp et al., 2014), will also be important.
5 NIRS agreement with alternative methods
VOP is widely used to assess PORH (Rosenberry and Nelson, 2020; Wilkinson and Webb, 2001). Studies comparing NIRS with VOP report mixed results. (De Blasi et al., 1994) reported excellent correlation between NIRS and VOP (r = 0.93) for forearm blood flow at rest and after 6-min ischemia. Others have also found that resting measures of blood flow using the venous occlusion method by NIRS and strain-gauge plethysmography are strongly correlated (Edwards et al., 1993; Van Beekvelt MC. et al., 2001). Contrastingly, Gomez et al. found negligible correlation with some NIRS parameters and acceptable agreement with others (Gomez et al., 2022). VOP is considered to represent tissue flow while NIRS interrogates only a small region of interest (∼1 cm3) which could explain inconsistencies between studies. Furthermore, the technical limitations in NIRS, such as variation in the contribution of Mb to the signal that would not impact VOP are likely to play a role in the discrepant findings.
Flow mediated dilatation (FMD) non-invasively assesses conduit vessel diameter up-stream of an arterial occlusion using ultrasound and, the extent to which the vessel can dilate post-ischemia is thought to largely depend on endothelial function. Downstream vasodilation is governed by multiple mechanisms (described above), all of which contribute to the NIRS signal. A positive correlation between reperfusion rate measured using NIRS and FMD has been shown in young, healthy men and in PAD patients (Cheatle et al., 1991; McLay et al., 2016a; Chung et al., 2018). Nevertheless, NIRS-measured PORH and FMD probably provide different but complementary information on the vascular response to ischemia.
6 Summary
We describe the application of NIRS for the assessment of PORH in skeletal muscle. We found no consensus on basic methodological approaches such as device sampling frequency, correction for adipose tissue, or analytical endpoints that should be calculated. Some areas for future research include: establishing the impact of variations in occlusion duration and comparing methods of accounting for different ischemic stimuli, the best method to account for ATT, the impact of different devices and establishing standards for minimum sampling rates. Addressing these methodological considerations should improve our understanding of PORH in skeletal muscle and facilitate interpretation of results across studies.
Author contributions
EH: Writing–original draft, Writing–review and editing. AJ: Writing–original draft, Writing–review and editing. SC: Writing–original draft, Writing–review and editing. AH: Writing–review and editing, Writing–original draft. SJ: Writing–original draft, Writing–review and editing.
Funding
The author(s) declare that financial support was received for the research, authorship, and/or publication of this article. SJ is funded by a BHF special project (grant number SP/F/21/150020). AJ is funded by a PhD studentship supported by the British Heart Foundation (grant number FS/19/63/34902). ADH works in a unit that receives support from the UK Medical Research Council (grant number MC_UU_12019/1). STC is supported by an Alzheimer’s Research UK David Carr Fellowship (ARUK-RF2021B-006).
Conflict of interest
The authors declare that the research was conducted in the absence of any commercial or financial relationships that could be construed as a potential conflict of interest.
Publisher’s note
All claims expressed in this article are solely those of the authors and do not necessarily represent those of their affiliated organizations, or those of the publisher, the editors and the reviewers. Any product that may be evaluated in this article, or claim that may be made by its manufacturer, is not guaranteed or endorsed by the publisher.
References
Anderson C. P., Park S. Y. (2023). Attenuated reactive hyperemia after prolonged sitting is associated with reduced local skeletal muscle metabolism: insight from artificial intelligence. Am. J. Physiol. Regul. Integr. Comp. Physiol. 325 (4), R380–R388. doi:10.1152/ajpregu.00067.2023
Anderson T. J., Charbonneau F., Title L. M., Buithieu J., Rose M. S., Conradson H., et al. (2011). Microvascular function predicts cardiovascular events in primary prevention: long-term results from the Firefighters and Their Endothelium (FATE) study. Circulation 123 (2), 163–169. doi:10.1161/CIRCULATIONAHA.110.953653
Artinis Medical Systems TN. (2022). Tissue Saturation Index (TSI) - absolute oxygenation measure in local tissues The Netherlands. Artinis Medical Systems. Available at: https://www.artinis.com/blogpost-all/2022/tissue-saturation-index-tsi-absolute-oxygenation-measure-in-local-tissues.
Barstow T. J. (2019a). Understanding near infrared spectroscopy and its application to skeletal muscle research. J. Appl. Physiol. (1985) 126 (5), 1360–1376. doi:10.1152/japplphysiol.00166.2018
Barstow T. J. (2019b). Understanding near infrared spectroscopy and its application to skeletal muscle research. J. Appl. Physiology 126 (5), 1360–1376. doi:10.1152/japplphysiol.00166.2018
Bayliss W. M. (1902). On the local reactions of the arterial wall to changes of internal pressure. J. physiology 28 (3), 220–231. doi:10.1113/jphysiol.1902.sp000911
Bekedam M. A., van Beek-Harmsen B. J., van Mechelen W., Boonstra A., van der Laarse W. J. (2009). Myoglobin concentration in skeletal muscle fibers of chronic heart failure patients. J. Appl. Physiology 107 (4), 1138–1143. doi:10.1152/japplphysiol.00149.2009
Bopp C. M., Townsend D. K., Barstow T. J. (2011). Characterizing near-infrared spectroscopy responses to forearm post-occlusive reactive hyperemia in healthy subjects. Eur. J. Appl. Physiol. 111 (11), 2753–2761. doi:10.1007/s00421-011-1898-z
Bopp C. M., Townsend D. K., Warren S., Barstow T. J. (2014). Relationship between brachial artery blood flow and total [hemoglobin+myoglobin] during post-occlusive reactive hyperemia. Microvasc. Res. 91, 37–43. doi:10.1016/j.mvr.2013.10.004
Cheatle T. R., Potter L. A., Cope M., Delpy D. T., Coleridge Smith P. D., Scurr J. H. (1991). Near-infrared spectroscopy in peripheral vascular disease. Br. J. Surg. 78 (4), 405–408. doi:10.1002/bjs.1800780408
Cheng B., Essackjee H., Ballard H. (2000). Evidence for control of adenosine metabolism in rat oxidative skeletal muscle by changes in pH. J. physiology 522 (3), 467–477. doi:10.1111/j.1469-7793.2000.t01-1-00467.x
Cheng X., Mao J. M., Xu X., Elmandjra M., Bush R., Christenson L., et al. (2004). Post-occlusive reactive hyperemia in patients with peripheral vascular disease. Clin. Hemorheol. Microcirc. 31 (1), 11–21.
Chung S., Rosenberry R., Ryan T. E., Munson M., Dombrowsky T., Park S., et al. (2018). Near-infrared spectroscopy detects age-related differences in skeletal muscle oxidative function: promising implications for geroscience. Physiol. Rep. 6 (3), e13588. doi:10.14814/phy2.13588
Corretti M. C., Anderson T. J., Benjamin E. J., Celermajer D., Charbonneau F., Creager M. A., et al. (2002). Guidelines for the ultrasound assessment of endothelial-dependent flow-mediated vasodilation of the brachial artery: a report of the International Brachial Artery Reactivity Task Force. J. Am. Coll. Cardiol. 39 (2), 257–265. doi:10.1016/s0735-1097(01)01746-6
Craig J. C., Broxterman R. M., Wilcox S. L., Chen C., Barstow T. J. (2017). Effect of adipose tissue thickness, muscle site, and sex on near-infrared spectroscopy derived total-[hemoglobin+ myoglobin]. J. Appl. Physiology 123 (6), 1571–1578. doi:10.1152/japplphysiol.00207.2017
Creteur J., Carollo T., Soldati G., Buchele G., De Backer D., Vincent J.-L. (2007). The prognostic value of muscle StO2 in septic patients. Intensive Care Med. 33 (9), 1549–1556. doi:10.1007/s00134-007-0739-3
Davis M. L., Barstow T. J. (2013). Estimated contribution of hemoglobin and myoglobin to near infrared spectroscopy. Respir. physiology and Neurobiol. 186 (2), 180–187. doi:10.1016/j.resp.2013.01.012
De Blasi R. A., Ferrari M., Natali A., Conti G., Mega A., Gasparetto A. (1994). Noninvasive measurement of forearm blood flow and oxygen consumption by near-infrared spectroscopy. J. Appl. Physiology 76 (3), 1388–1393. doi:10.1152/jappl.1994.76.3.1388
Dellinger J. R., Figueroa A., Gonzales J. U. (2023). Reactive hyperemia half-time response is associated with skeletal muscle oxygen saturation changes during cycling exercise. Microvasc. Res. 149, 104569. doi:10.1016/j.mvr.2023.104569
de Oliveira G. V., Volino-Souza M., Leitao R., Pinheiro V., Alvares T. S. (2020). Is flow-mediated dilatation associated with near-infrared spectroscopy-derived magnitude of muscle O2 desaturation in healthy young and individuals at risk for cardiovascular disease? Microvasc. Res. 129, 103967. doi:10.1016/j.mvr.2019.103967
Desanlis J., Gordon D., Calveyrac C., Cottin F., Gernigon M. (2022). Intra-and inter-day reliability of the NIRS portamon device after three induced muscle ischemias. Sensors 22 (14), 5165. doi:10.3390/s22145165
Didier K. D., Hammer S. M., Alexander A. M., Rollins K. S., Barstow T. J. (2022). The acute effects of passive heating on endothelial function, muscle microvascular oxygen delivery, and expression of serum HSP90α. Microvasc. Res. 142, 104356. doi:10.1016/j.mvr.2022.104356
Edwards A., Richardson C., Van Der Zee P., Elwell C., Wyatt J., Cope M., et al. (1993). Measurement of hemoglobin flow and blood flow by near-infrared spectroscopy. J. Appl. Physiology 75 (4), 1884–1889. doi:10.1152/jappl.1993.75.4.1884
Engelke K. A., Halliwill J. R., Proctor D. N., Dietz N. M., Joyner M. J. (1996). Contribution of nitric oxide and prostaglandins to reactive hyperemia in human forearm. J. Appl. Physiology 81 (4), 1807–1814. doi:10.1152/jappl.1996.81.4.1807
Fellahi J. L., Butin G., Zamparini G., Fischer M. O., Gérard J. L., Hanouz J. L. (2014). Lower limb peripheral NIRS parameters during a vascular occlusion test: an experimental study in healthy volunteers. Ann. Fr. Anesth. Reanim. 33 (1), e9–e14. doi:10.1016/j.annfar.2013.11.014
Gayda M., Juneau M., Tardif J. C., Harel F., Levesque S., Nigam A. (2015). Cardiometabolic and traditional cardiovascular risk factors and their potential impact on macrovascular and microvascular function: preliminary data. Clin. Hemorheol. Microcirc. 59 (1), 53–65. doi:10.3233/CH-141816
Gómez H., Mesquida J., Simon P., Kim H. K., Puyana J. C., Ince C., et al. (2009). Characterization of tissue oxygen saturation and the vascular occlusion test: influence of measurement sites, probe sizes and deflation thresholds. Crit. Care 13, S3–S7. doi:10.1186/cc8001
Gomez M., Montalvo S., Gurovich A. N. (2022). Near infrared spectroscopy is not a surrogate of venous occlusion plethysmography to assess microvascular resting blood flow and function. Int. J. Exerc. Sci. 15 (2), 1616–1626.
Gonzalez M. R. (2022). “The effects of melatonin on vascular function,” in Oxidative stress and blood pressure reactivity during a high sodium diet. University of Delaware.
Granger H., Shepherd Jr A. (1973). Intrinsic microvascular control of tissue oxygen delivery. Microvasc. Res. 5 (1), 49–72. doi:10.1016/s0026-2862(73)80006-8
Hammer S. M., Hueber D. M., Townsend D. K., Huckaby L. M., Alexander A. M., Didier K. D., et al. (1985)2019). Effect of assuming constant tissue scattering on measured tissue oxygenation values during tissue ischemia and vascular reperfusion. J. Appl. Physiol. 127 (1), 22–30. doi:10.1152/japplphysiol.01138.2018
Hornig B., Kohler C., Drexler H. (1997). Role of bradykinin in mediating vascular effects of angiotensin-converting enzyme inhibitors in humans. Circulation 95 (5), 1115–1118. doi:10.1161/01.cir.95.5.1115
Inaba Y., Chen J. A., Bergmann S. R. (2010). Prediction of future cardiovascular outcomes by flow-mediated vasodilatation of brachial artery: a meta-analysis. Int. J. Cardiovasc Imaging 26 (6), 631–640. doi:10.1007/s10554-010-9616-1
Ishibashi Y., Takahashi N., Shimada T., Sugamori T., Sakane T., Umeno T., et al. (2006). Short duration of reactive hyperemia in the forearm of subjects with multiple cardiovascular risk factors. Circ. J. 70 (1), 115–123. doi:10.1253/circj.70.115
Jacobs N., Mos D., Bloemers F. W., van der Laarse W. J., Jaspers R. T., van der Zwaard S. (2023). Low myoglobin concentration in skeletal muscle of elite cyclists is associated with low mRNA expression levels. Eur. J. Appl. physiology 123 (7), 1469–1478. doi:10.1007/s00421-023-05161-z
Jarm T., Kragelj R., Liebert A., Lukasiewitz P., Erjavec T., Preseren-Strukelj M., et al. (2003). Postocclusive reactive hyperemia in healthy volunteers and patients with peripheral vascular disease measured by three noninvasive methods. Adv. Exp. Med. Biol. 530, 661–669. doi:10.1007/978-1-4615-0075-9_66
Jones S., Tillin T., Williams S., Rapala A., Chaturvedi N., Hughes A. D. (2022). Skeletal muscle tissue saturation changes measured using near infrared spectroscopy during exercise are associated with post-occlusive reactive hyperaemia. Front. Physiology 13, 919754. doi:10.3389/fphys.2022.919754
Koirala B., Concas A., Sun Y., Gladden L. B., Lai N. (2023). Relationship between muscle venous blood oxygenation and near-infrared spectroscopy: quantitative analysis of the Hb and Mb contributions. J. Appl. Physiology 134 (5), 1063–1074. doi:10.1152/japplphysiol.00406.2022
Kooijman H. M., Hopman M. T., Colier W. N., van der Vliet J. A., Oeseburg B. (1997). Near infrared spectroscopy for noninvasive assessment of claudication. J. Surg. Res. 72 (1), 1–7. doi:10.1006/jsre.1997.5164
Kragelj R., Jarm T., Erjavec T., Presern-Strukelj M., Miklavcic D. (2001). Parameters of postocclusive reactive hyperemia measured by near infrared spectroscopy in patients with peripheral vascular disease and in healthy volunteers. Ann. Biomed. Eng. 29 (4), 311–320. doi:10.1114/1.1359451
Lacroix S., Gayda M., Gremeaux V., Juneau M., Tardif J. C., Nigam A. (2012). Reproducibility of near-infrared spectroscopy parameters measured during brachial artery occlusion and reactive hyperemia in healthy men. J. Biomed. Opt. 17 (7), 077010. doi:10.1117/1.JBO.17.7.077010
Lai N., Zhou H., Saidel G. M., Wolf M., McCully K., Gladden L. B., et al. (2009). Modeling oxygenation in venous blood and skeletal muscle in response to exercise using near-infrared spectroscopy. J. Appl. Physiology 106 (6), 1858–1874. doi:10.1152/japplphysiol.91102.2008
Mayeur C., Campard S., Richard C., Teboul J.-L. (2011). Comparison of four different vascular occlusion tests for assessing reactive hyperemia using near-infrared spectroscopy. Crit. Care Med. 39 (4), 695–701. doi:10.1097/CCM.0b013e318206d256
McGranahan M. J., Kibildis S. W., McCully K. K., O'Connor P. J. (2023). Evaluation of inter-rater and test-retest reliability for near-infrared spectroscopy reactive hyperemia measures. Microvasc. Res. 148, 104532. doi:10.1016/j.mvr.2023.104532
McLay K. M., Fontana F. Y., Nederveen J. P., Guida F. F., Paterson D. H., Pogliaghi S., et al. (2016a). Vascular responsiveness determined by near-infrared spectroscopy measures of oxygen saturation. Exp. Physiol. 101 (1), 34–40. doi:10.1113/EP085406
McLay K. M., Nederveen J. P., Pogliaghi S., Paterson D. H., Murias J. M. (2016b). Repeatability of vascular responsiveness measures derived from near-infrared spectroscopy. Physiol. Rep. 4 (9), e12772. doi:10.14814/phy2.12772
Möller P., Sylven C. (1981). Myoglobin in human skeletal muscle. Scand. J. Clin. laboratory investigation 41 (5), 479–482. doi:10.3109/00365518109090486
Monthé-Sagan K., Fischer M. O., Saplacan V., Gerard J. L., Hanouz J. L., Fellahi J. L. (2016). Near-infrared spectroscopy to assess microvascular dysfunction: a prospective pilot study in cardiac surgery patients. J. Crit. Care 31 (1), 264–268. doi:10.1016/j.jcrc.2015.09.026
Orbegozo Cortés D., Rahmania L., Irazabal M., Santacruz C., Fontana V., De Backer D., et al. (2016). Microvascular reactivity is altered early in patients with acute respiratory distress syndrome. Respir. Res. 17 (1), 59. doi:10.1186/s12931-016-0375-y
Paine N. J., Hinderliter A. L., Blumenthal J. A., Adams K. F., Sueta C. A., Chang P. P., et al. (2016). Reactive hyperemia is associated with adverse clinical outcomes in heart failure. Am. Heart J. 178, 108–114. doi:10.1016/j.ahj.2016.05.008
Rosenberry R., Munson M., Chung S., Samuel T. J., Patik J., Tucker W. J., et al. (2018). Age-related microvascular dysfunction: novel insight from near-infrared spectroscopy. Exp. Physiol. 103 (2), 190–200. doi:10.1113/EP086639
Rosenberry R., Nelson M. D. (2020). Reactive hyperemia: a review of methods, mechanisms, and considerations. Am. J. Physiol. Regul. Integr. Comp. Physiol. 318 (3), R605-R618–r18. doi:10.1152/ajpregu.00339.2019
Scholkmann F., Kleiser S., Metz A. J., Zimmermann R., Mata Pavia J., Wolf U., et al. (2014). A review on continuous wave functional near-infrared spectroscopy and imaging instrumentation and methodology. Neuroimage 85 (Pt 1), 6–27. doi:10.1016/j.neuroimage.2013.05.004
Shimoda L. A., Polak J. (2011). Hypoxia. 4. Hypoxia and ion channel function. Am. J. Physiol. Cell Physiol. 300 (5), C951–C967. doi:10.1152/ajpcell.00512.2010
Shu X., Keller T. S., Begandt D., Butcher J. T., Biwer L., Keller A. S., et al. (2015). Endothelial nitric oxide synthase in the microcirculation. Cell. Mol. life Sci. 72, 4561–4575. doi:10.1007/s00018-015-2021-0
Siafaka A., Angelopoulos E., Kritikos K., Poriazi M., Basios N., Gerovasili V., et al. (2007). Acute effects of smoking on skeletal muscle microcirculation monitored by near-infrared spectroscopy. Chest 131 (5), 1479–1485. doi:10.1378/chest.06-2017
S. Suzuki, S. Takasaki, T. Ozaki, and Y. Kobayashi (1999). “Tissue oxygenation monitor using NIR spatially resolved spectroscopy,” Opt. Tomogr. Spectrosc. tissue, III. SPIE. doi:10.1117/12.356862
Talbot J., Maves L. (2016). Skeletal muscle fiber type: using insights from muscle developmental biology to dissect targets for susceptibility and resistance to muscle disease. Wiley Interdiscip. Rev. Dev. Biol. 5 (4), 518–534. doi:10.1002/wdev.230
Theurot D., Dupuy O., Louis J., Douzi W., Morin R., Arc-Chagnaud C., et al. (2023). Partial-body cryostimulation does not impact peripheral microvascular responsiveness but reduces muscular metabolic O2 consumption (mV˙O2) at rest. Cryobiology 112, 104561. doi:10.1016/j.cryobiol.2023.104561
Townsend D. K., Deysher D. M., Wu E. E., Barstow T. J. (2019). Reduced insulin sensitivity in young, normoglycaemic subjects alters microvascular tissue oxygenation during postocclusive reactive hyperaemia. Exp. Physiol. 104 (6), 967–974. doi:10.1113/EP087216
Van Beekvelt M., Borghuis M., Van Engelen B., Wevers R., Colier W. (2001a). Adipose tissue thickness affects in vivo quantitative near-IR spectroscopy in human skeletal muscle. Clin. Sci. 101 (1), 21–28. doi:10.1042/cs20000247
Van Beekvelt M. C., Colier W. N., Wevers R. A., Van Engelen B. G. (2001b). Performance of near-infrared spectroscopy in measuring local O2 consumption and blood flow in skeletal muscle. J. Appl. physiology 90 (2), 511–519. doi:10.1152/jappl.2001.90.2.511
Whyte E., Thomas S., Marzolini S. (2022). Muscle oxygenation of the paretic and nonparetic legs during and after arterial occlusion in chronic stroke. J. Stroke Cerebrovasc. Dis. 31 (3), 106265. doi:10.1016/j.jstrokecerebrovasdis.2021.106265
Wilkinson I. B., Webb D. J. (2001). Venous occlusion plethysmography in cardiovascular research: methodology and clinical applications. Br. J. Clin. Pharmacol. 52 (6), 631–646. doi:10.1046/j.0306-5251.2001.01495.x
Willingham T. B., Southern W. M., McCully K. K. (2016). Measuring reactive hyperemia in the lower limb using near-infrared spectroscopy. J. Biomed. Opt. 21 (9), 091302. doi:10.1117/1.JBO.21.9.091302
Wong B. J., Wilkins B. W., Holowatz L. A., Minson C. T. (2003). Nitric oxide synthase inhibition does not alter the reactive hyperemic response in the cutaneous circulation. J. Appl. Physiology 95 (2), 504–510. doi:10.1152/japplphysiol.00254.2003
Zamparini G., Butin G., Fischer M. O., Gérard J. L., Hanouz J. L., Fellahi J. L. (2015). Noninvasive assessment of peripheral microcirculation by near-infrared spectroscopy: a comparative study in healthy smoking and nonsmoking volunteers. J. Clin. Monit. Comput. 29 (5), 555–559. doi:10.1007/s10877-014-9631-1
Keywords: NIRS (near-infrared spectroscopy), post-occlusive reactive hyperaemia, microvascular, skeletal muscle, methods
Citation: Hendrick E, Jamieson A, Chiesa ST, Hughes AD and Jones S (2024) A short review of application of near-infrared spectroscopy (NIRS) for the assessment of microvascular post-occlusive reactive hyperaemia (PORH) in skeletal muscle. Front. Physiol. 15:1480720. doi: 10.3389/fphys.2024.1480720
Received: 14 August 2024; Accepted: 29 October 2024;
Published: 27 November 2024.
Edited by:
Carlos Alonso Escudero, University of the Bío Bío, ChileReviewed by:
Roland Pittman, Virginia Commonwealth University, United StatesBen Jones, University of Essex, United Kingdom
Copyright © 2024 Hendrick, Jamieson, Chiesa, Hughes and Jones. This is an open-access article distributed under the terms of the Creative Commons Attribution License (CC BY). The use, distribution or reproduction in other forums is permitted, provided the original author(s) and the copyright owner(s) are credited and that the original publication in this journal is cited, in accordance with accepted academic practice. No use, distribution or reproduction is permitted which does not comply with these terms.
*Correspondence: Siana Jones, c2lhbmEuam9uZXNAdWNsLmFjLnVr