- 1School of Kinesiology, The University of British Columbia, Vancouver, BC, Canada
- 2Department of Pathology and Laboratory Medicine, The University of British Columbia, Vancouver, BC, Canada
- 3Department of Sports and Exercise, Máxima Medical Centre, Veldhoven, Netherlands
- 4Department of Biomedical Physiology and Kinesiology, Faculty of Science, Simon Fraser University, Burnaby, BC, Canada
- 5Division of Sport and Exercise Medicine, Faculty of Medicine, The University of British Columbia, Vancouver, BC, Canada
Introduction: Wearable near-infrared spectroscopy (NIRS) can be used during dynamic exercise to reflect the balance of muscle oxygen delivery and uptake. This study describes the behaviour and reliability of postexercise reoxygenation with NIRS as a function of exercise intensity at four muscle sites during an incremental cycling test. We discuss physiological components of faster and slower reoxygenation kinetics in the context of sport science and clinical applications. We hypothesised that reoxygenation would be slower at higher intensity, and that locomotor muscles would be faster than accessory muscles. We quantified test-retest reliability and agreement for each site.
Methods: Twenty-one trained cyclists performed two trials of an incremental cycling protocol with 5-min work stages and 1-min rest between stages. NIRS was recorded from the locomotor vastus lateralis and rectus femoris muscles, and accessory lumbar paraspinal and lateral deltoid muscles. Reoxygenation time course was analysed as the half-recovery time (HRT) from the end of work to half of the peak reoxygenation amplitude during rest. Coefficient of variability (CV) between participants, standard error of the measurement (SEM) within participants, and intraclass correlation coefficient (ICC) for test-retest reliability were evaluated at 50%, 75%, and 100% peak workloads. A linear mixed-effects model was used to compare differences between workloads and muscle sites.
Results: HRT was slower with increasing workload in the VL, RF, and PS, but not DL. VL had the fastest reoxygenation (lowest HRT) across muscle sites at all workloads (HRT = 8, 12, 17 s at 50%, 75%, 100% workload, respectively). VL also had the greatest reliability and agreement. HRT was sequentially slower between muscle sites in the order of VL < RF < PS < DL, and reliability was lower than for the VL.
Discussion: This study highlights the potential for using wearable NIRS on multiple muscle sites during exercise. Reoxygenation kinetics differ between local muscle sites with increasing intensity. Moderate-to-good reliability in the VL support its increasing use in sport science and clinical applications. Lower reliability in other muscle sites suggest they are not appropriate to be used alone, but may add information when combined to better reflect systemic intensity and fatigue during exercise at different intensities.
1 Introduction
Near-infrared spectroscopy (NIRS) is a promising non-invasive, wearable technology that evaluates the balance of oxygen (O2) delivery and O2 uptake in local tissue during exercise in real time. Commercially available wearable NIRS devices have become more compact, powerful, and accessible in recent years with increasing adoption in sport science and clinical applications (Cornelis et al., 2021; Perrey et al., 2024). Wearable NIRS records the ratio of oxygenated and deoxygenated haemoglobin and myoglobin (oxy- and deoxy[HbMb], respectively), and reports a percent value of muscle oxygen saturation (SmO2). SmO2 represents the relative O2 saturation of Hb and Mb binding sites within the NIRS field of view. Technical details of NIRS can be found elsewhere (Barstow, 2019; Hamaoka and McCully, 2019).
Despite being reported on a 0%–100% scale, wearable SmO2 is a relatively scaled measurement with large differences in percent values observed between individuals and between different NIRS devices (McManus et al., 2018). SmO2 from two common wearable NIRS devices (Moxy monitor, Fortiori Design LLC and Portamon, Artinis Medical Systems) have been observed with test-retest agreement of approximately ±5–10 %SmO2, standard deviation between individuals of ±5–25 %SmO2, and mean bias between devices of ±30–45 %SmO2 during different exercise tasks (Crum et al., 2017; McManus et al., 2018; van Hooff et al., 2022b; Yogev et al., 2023; Skotzke et al., 2024). Because of this limitation in interpreting ‘absolute’ SmO2 values, NIRS research often observes how SmO2 changes over time or as a function of exercise intensity (Kirby et al., 2021; Perrey et al., 2024).
The direction and rate of change of SmO2 can tell us about the dynamic matching of muscle O2 delivery (m
During recovery from exercise, the energetic demand of work is reduced and energy from m
In general, faster postexercise reoxygenation implies a combination of: (1) greater excess m
Reoxygenation kinetics are of particular interest in sport science and clinical applications. For example, faster reoxygenation is observed after endurance training (Puente-Maestu et al., 2003), and are associated with younger and higher fitness participants (Ichimura et al., 2006; Marwood et al., 2011), and with improved endurance performance across various sporting tasks (Buchheit and Ufland, 2011; Paquette et al., 2020). In clinical evaluation, reoxygenation is consistently shown to be delayed in patients with peripheral vascular disease (Baker et al., 2017; Cornelis et al., 2021), diabetes (Baltrūnas et al., 2021), heart failure (Belardinelli et al., 1997), mitochondrial myopathies (Bravo et al., 2012), and sport-related vascular conditions (van Hooff et al., 2022a; Ritchie et al., 2022). Thus, slower reoxygenation is associated with conditions which impair either m
The majority of sport science and clinical research observes NIRS responses in only one muscle site at a time, most often the quadriceps or calf muscles in the lower limb, and biceps or forearm in the upper extremity (Perrey et al., 2024). During exercise, systemic blood flow and O2 delivery is tightly controlled and directed toward the most metabolically active tissues via sympathetic vasoconstriction and locally mediated vasodilation (Laughlin et al., 2012). When NIRS responses are monitored in non-locomotor muscle sites, they appear to demonstrate a reduced metabolic priority (Ogata et al., 2007; Osawa et al., 2017). Therefore, investigating NIRS at multiple muscle sites simultaneously may allow for improved interpretation of how local and systemic processes interact to meet exercise demands (Kirby et al., 2021; Yogev et al., 2022; Perrey et al., 2024).
By observing reoxygenation kinetics across a range of exercise intensities at multiple muscle sites, we hope to gain more insight into how O2 delivery is redirected systemically toward tissues with different rates of metabolic activity, and between muscles with different contraction patterns, e.g., postural stabiliser muscles vs. rhythmically contracting locomotor muscles. It will also be useful to understand the reliability and variability between participants for reoxygenation at these less common muscle sites.
1.1 Purpose, objective, hypotheses
The primary objective of this paper is to describe the time course of NIRS reoxygenation as a function of exercise intensity during an incremental multi-stage cycling protocol, at multiple locomotor and accessory muscle sites. The secondary objective is to describe between-participant variability, test-retest reliability, and within-participant agreement of reoxygenation time course at these muscle sites.
We hypothesised that (1) reoxygenation would be slower with increasing exercise intensity, and (2) reoxygenation would be faster in locomotor muscles than in accessory muscles.
2 Methods
2.1 Participants and experimental design
Twenty-one trained cyclists (n = 10 females, n = 11 males; Table 1) volunteered and provided written informed consent to participate in two experimental trials each of an identical incremental multi-stage cycling protocol consisting of 5-min work stages with 1-min rest periods between stages. The workload began at 1.0 W kg-1 and increased by 0.5 W kg-1 per stage to maximal exercise tolerance. Participants were instructed to maintain the same exercise, diet, and sleep routines for 24 h prior to each visit, and to avoid strenuous exercise for 24 h, and caffeine for 4 h prior. Trials were performed 1 or 2 weeks apart at the same time of day (±1 h). Participants’ body mass and height were recorded at the start of the first visit. Skinfold (SKF) measurements (Harpenden Skinfold Calliper, Baty International, West Sussex, England) were recorded at the placement locations of the NIRS sensors (detailed below). Participants were trained or well-trained and all competitive. This study was approved by the clinical research ethics board of the University of British Columbia and was conducted in accordance with principles established in the Declaration of Helsinki.
Experimental design for this study has been reported previously (Yogev et al., 2023). Briefly, cycling exercise was performed on the participants’ own bike mounted to an electronically controlled trainer (Tacx NEO 2T, Garmin International Inc., Olathe, KS, United States) at a self-selected cadence between 80–100 rpm, matched between both trials. Expired metabolic gases were recorded by an open-circuit mixing chamber analyser (TrueOne 2400, ParvoMedics Inc., Sandy, UT, United States).
2.2 NIRS instrumentation
Muscle oxygenation was recorded with four wearable NIRS sensors (Moxy Monitor, Fortiori Design LLC., Hutchinson, MN, United States) positioned on the vastus lateralis (VL), rectus femoris (RF), lumbar paraspinal (PS), and lateral deltoid (DL) muscles. Placement was on the muscle bellies of the VL at ⅓ the distance from the patella to greater trochanter; the RF at ½ the distance from the patella to anterior superior iliac spine; the PS lateral to the L3 vertebra on the erector spinae; and the DL at ½ the distance from the acromion to the deltoid tuberosity on the humerus. NIRS sensors were positioned with the athlete standing and secured with adhesive tape and a plastic light shield to minimise signal interference from ambient light and movement. All measurements were taken from the right side.
The Moxy monitor is a wearable NIRS sensor which can resolve SmO2 on a 0%–100% scale and an arbitrarily scaled concentration of total[HbMb] (Feldmann et al., 2019) from a single LED source and two detectors at 12.5 and 25 mm inter-optode separation, giving a maximum insonation depth of approximately 12.5 mm. SmO2 is resolved across the two optode pairs. Technical considerations of the Moxy device are described elsewhere (Crum et al., 2017; McManus et al., 2018; Feldmann et al., 2019).
2.3 Data analysis
Data processing and statistical analysis were performed in R version 4.3.3 (R Core Team, 2021) with the packages lme4 1.1–35.1 (Bates et al., 2015), lmerTest 3.1–3 (Kuznetsova et al., 2017), emmeans 1.10.0 (Lenth et al., 2023), and SimplyAgree 0.2.0 (Caldwell, 2022). SmO2 was analysed as the primary NIRS signal (McManus et al., 2018). SmO2 was recorded at 0.5 Hz and smoothed with a 5-s symmetrical moving average to 1 Hz as per manufacturer recommended settings.
Reoxygenation bouts were evaluated from SmO2 during rest periods after each work stage. The mean SmO2 value during the final 30 s of the work interval represented the starting value for reoxygenation. The peak SmO2 value observed during the rest period represented the ending value. This peak was defined as the first SmO2 value with no higher subsequent peaks within 30 s during a recovery time window, which was defined separately for the locomotor and accessory muscles. For locomotor muscle sites (VL and RF), this recovery window was to the start of the subsequent work stage. For accessory muscle sites (PS and DL), this window was to 60 s after the start of the subsequent work stage. The recovery window after the final work stage was 4 min for all muscles. The total reoxygenation amplitude was the difference between the peak and end-work mean SmO2 values. Reoxygenation time course was quantified as the half-recovery time (HRT), calculated as the time required to recover half of the total SmO2 amplitude (see representative schematic in Figure 1F).
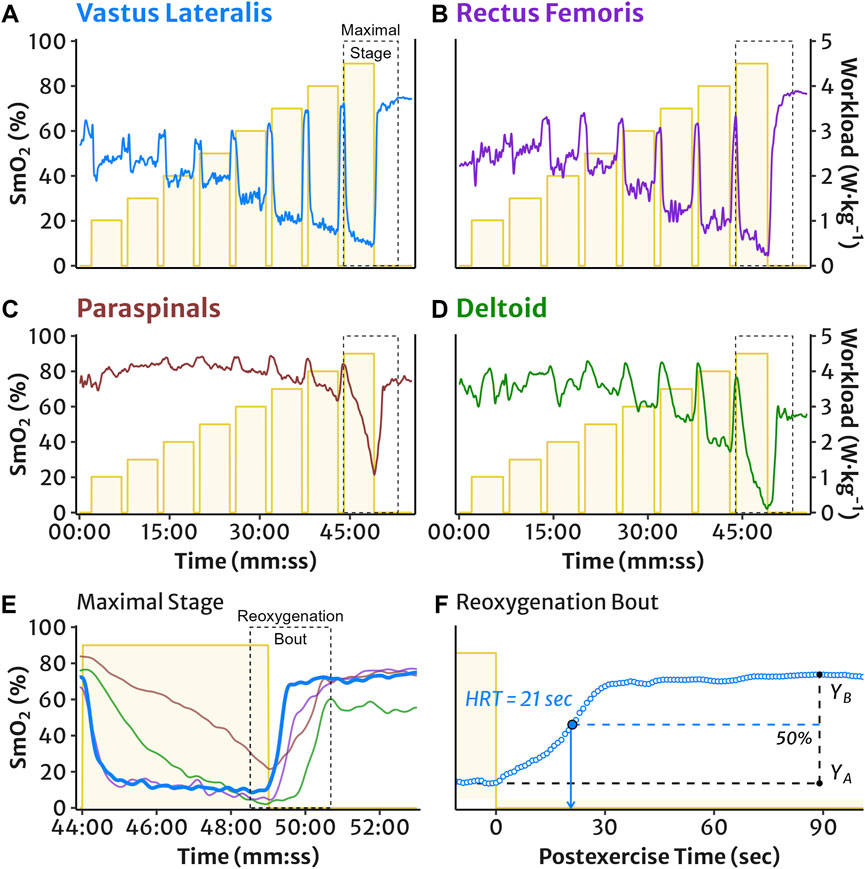
Figure 1. Representative example of the incremental multi-stage cycling protocol and NIRS muscle oxygen saturation (SmO2) measured in (A) vastus lateralis, (B) rectus femoris, (C) lumbar paraspinals, and (D) lateral deltoid muscles. The maximal work stage is outlined and expanded in panel (E): SmO2 demonstrates typical deoxygenation during an exercise interval, followed by postexercise reoxygenation. The maximal reoxygenation bout is outlined and expanded for the VL in panel (F): representative example of VL SmO2 reoxygenation half-recovery time (HRT), calculated as the time required to recover half of the total reoxygenation amplitude, i.e. 50% of the difference between the mean SmO2 value at the end of work (YA) and the peak recovery SmO2 value (YB).
Of the 504 total reoxygenation bouts recorded across 21 participants, two trials, three target work stages, and four muscle sites, there were 43 bouts (8.5%) with missing data where HRT could not be calculated. In two trials, the NIRS recording software failed after the final work stage and reoxygenation was lost for all sites at 100% workload. The NIRS device was not secured well enough at the PS in five trials and the DL in two trials, resulting in unusable data due to noise from light and/or movement. The device failed to record entirely for two trials in the RF, one trial each at the PS and DL, and in one individual reoxygenation bout each at the PS and DL.
We also analysed reoxygenation kinetics with a monoexponential function, however over 25% of the reoxygenation bouts did not conform to a specific phenomenological curve, evaluated by a coefficient of determination pseudo-R2 ≥0.85 and physiologically plausible mean response time between zero and the time of the peak SmO2 value. Therefore, we chose to only discuss the non-parametric HRT estimation of reoxygenation time. Please see Limitations section for further discussion, and Supplementary Material for results of the monoexponential kinetics analysis.
2.4 Between-participant variability and test-retest reliability
Between-participant variability was determined for HRT at work stages corresponding to 50%, 75%, and 100% Wpeak for each of the four muscle sites. This was quantified for absolute variability as the group-level median and interquartile range (Q1-Q3 or IQR) and for relative variability as the coefficient of variation (CV) between participants. Relative reliability was quantified as intraclass correlation coefficient (ICC2,1) and interpreted as poor (<0.5), moderate (0.5–0.75), good (0.75–0.9), and excellent (>0.9) (Koo and Li, 2016). Absolute test-retest reliability and within-participant agreement was quantified as the standard error of the measurement (SEM) and minimal detectable change (MDC), in original units of seconds (Atkinson and Nevill, 1998; Weir, 2005). Within-participant CV was also calculated.
2.5 Statistical analysis
Data are displayed as mean (SD) where normally distributed, and as median (Q1-Q3) where non-normally distributed, unless indicated otherwise. Alpha for all comparisons was set to 0.05. To compare reoxygenation across exercise intensity and between muscle sites, a linear mixed-effects model was used to analyse HRT with fixed effects for workload (%Wpeak with a continuous quadratic spline) and muscle site (VL, RF, PS, DL). Random effects of participant were included for the model slope and intercept. Trial was initially included as an additional fixed effect, however the final chosen model excluding trial was preferred based on the Akaike Information Criterion (AIC) and Bayesian information criterion (BIC). Pairwise post hoc estimated marginal means were contrasted between workloads and muscle sites at 50%, 75%, and 100% Wpeak using the Tukey method to correct for multiple comparisons. Model residuals were examined visually from Q-Q and other residual plots to check for normality and heteroscedasticity.
3 Results
The incremental multi-stage cycling protocol and HRT measurement of SmO2 reoxygenation are shown in Figure 1. Descriptive reoxygenation data and group-level coefficients of variation at 50%, 75%, and 100% workloads are reported in Table 2.
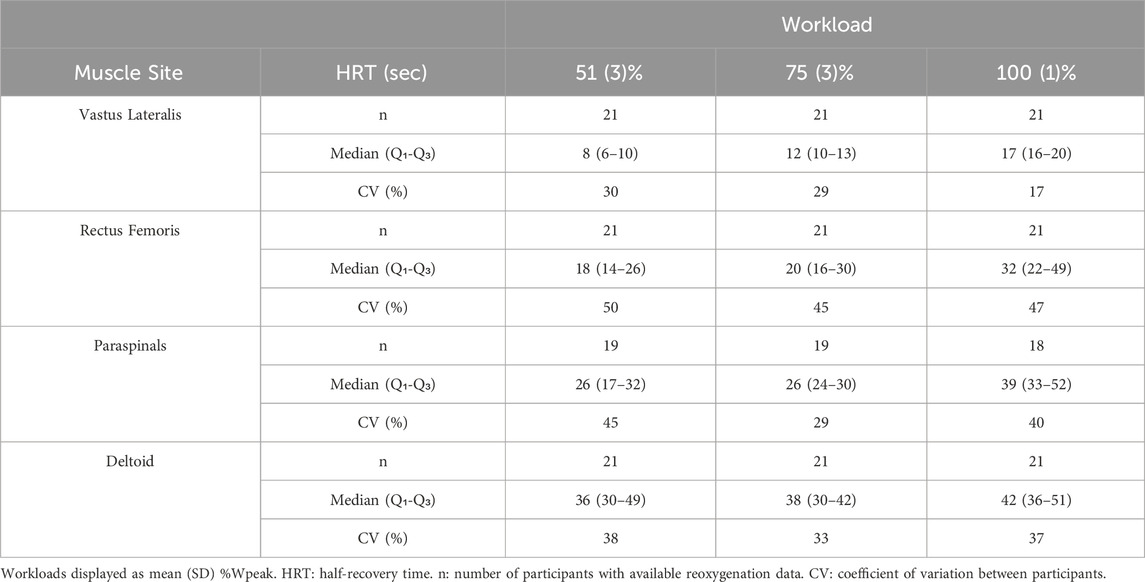
Table 2. Reoxygenation descriptive data at work stages corresponding to 50%, 75%, and 100% peak workload.
3.1 Comparison of reoxygenation with increasing exercise intensity
Linear mixed-effects modelling of HRT as a function of exercise intensity in four muscle sites are shown in Figure 2. Post hoc comparisons of estimated marginal mean HRT between 50%, 75%, and 100% workloads indicated that reoxygenation was significantly slower sequentially with increasing workload in all muscle sites (all p < 0.05) apart from the DL where HRT was not different across workloads (Table 3). See Supplementary Table S1 for full HRT model coefficients.
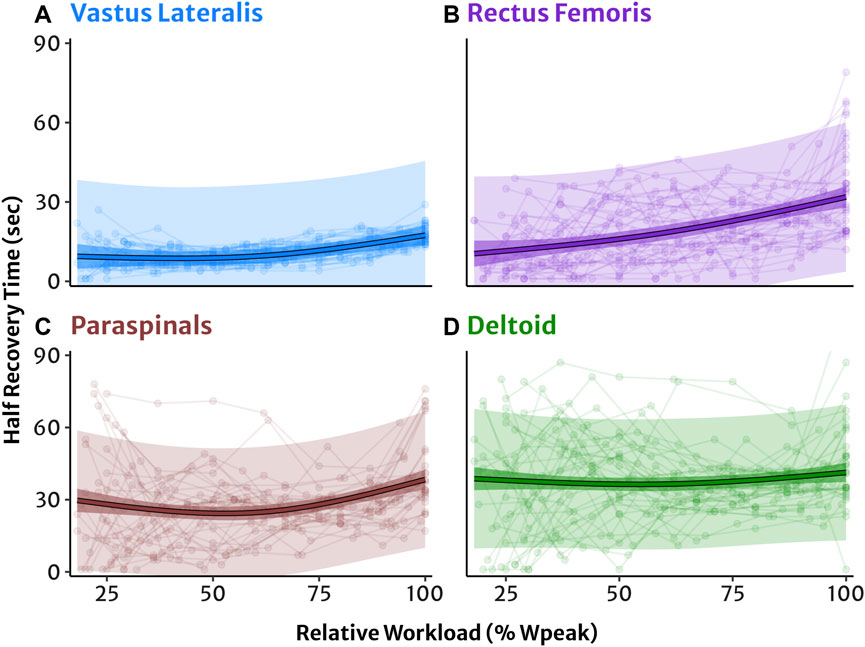
Figure 2. NIRS reoxygenation in four muscle sites modelled as a function of exercise intensity relative to each participant’s 100% peak workload. (A) Vastus lateralis, (B) rectus femoris, (C) lumbar paraspinals, and (D) lateral deltoid muscles. Half-recovery time (HRT) in seconds with individual datapoints (dots and lines). Predicted trendlines, dark shaded 95% confidence intervals, and light shaded 95% prediction intervals derived from the linear mixed-effects model.
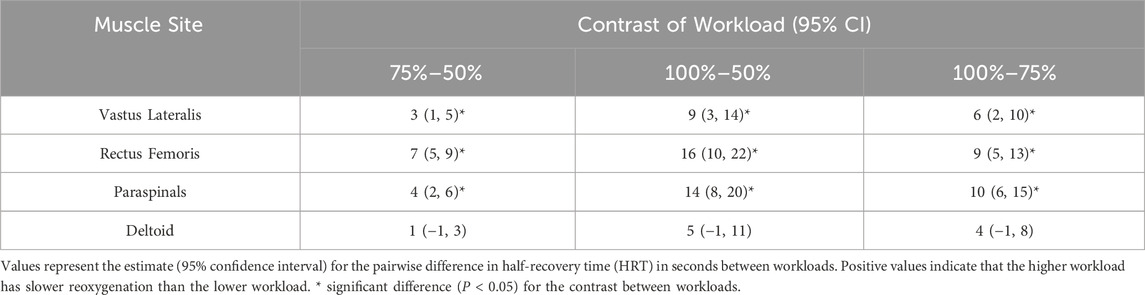
Table 3. Estimated marginal contrasts of workloads between reoxygenation at 50%, 75%, and 100% peak workload in each muscle site from the linear mixed-effects model.
3.2 Comparison between locomotor and accessory muscle sites
Post hoc comparisons of estimated marginal mean HRT between each muscle site at 50%, 75%, and 100% workloads indicated that reoxygenation was significantly slower sequentially in order of VL < RF < PS < DL (p < 0.05) at each workload, except for between the PS and DL which were not different at 100% (Table 4).
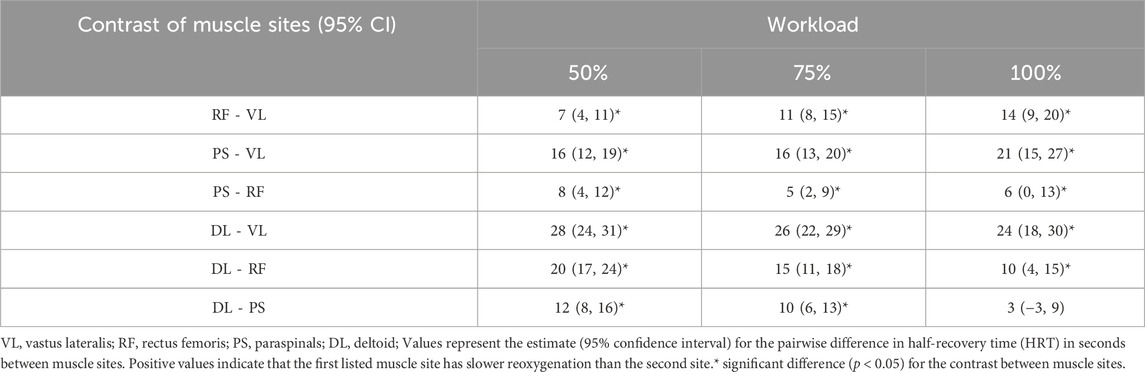
Table 4. Estimated marginal contrasts of muscle sites between reoxygenation at 50%, 75%, and 100% peak workload from the linear mixed-effects model.
3.3 Between-participant variability and test-retest reliability
Test-retest agreement for HRT values between trial 1 and 2 are shown in Figure 3. Between-participant variability tended to be greater in absolute (IQR) and relative (CV) terms in sequential order of VL < PS < DL < RF at equivalent workloads (Table 2). Within-participant agreement tended to be lower in absolute terms (SEM, MDC) in the order of VL < RF ≈ PS < DL, but in relative terms (CV) RF had the greatest variability and lowest agreement. This resulted in mostly poor to moderate relative reliability (ICC) estimates, with good reliability observed only in the VL at 50% and 75% workloads. Descriptive data for test-retest reliability and within-participant agreement are reported in Table 5.
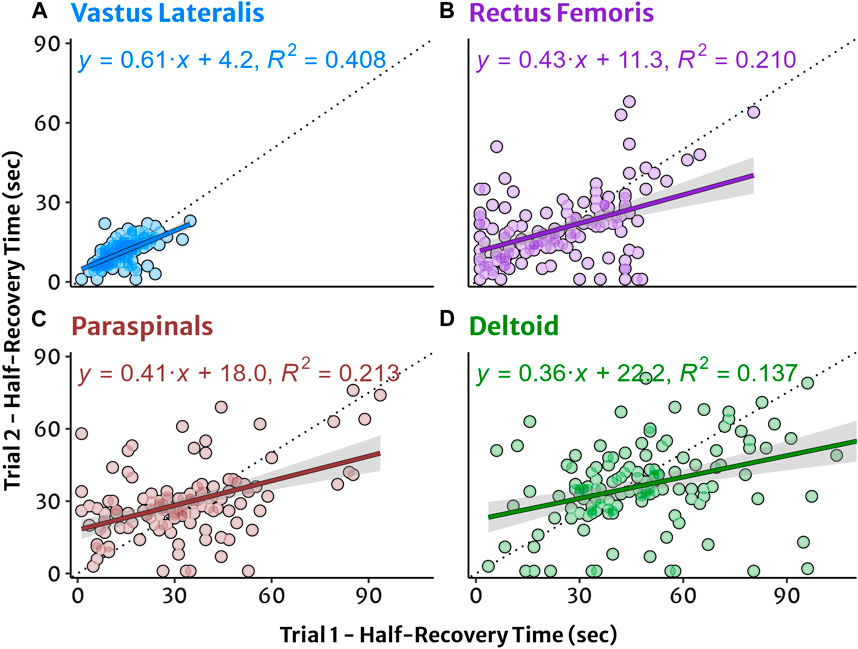
Figure 3. Test-retest agreement for NIRS reoxygenation in four muscle sites plotted from trial 1 and trial 2. (A) Vastus lateralis, (B) rectus femoris, (C) lumbar paraspinals, and (D) lateral deltoid muscles. Half-recovery time (HRT) in seconds with individual datapoints. Simple linear regression trendlines and shaded 95% confidence interval for HRT with equation and coefficient of determination (R2) provided for descriptive information.
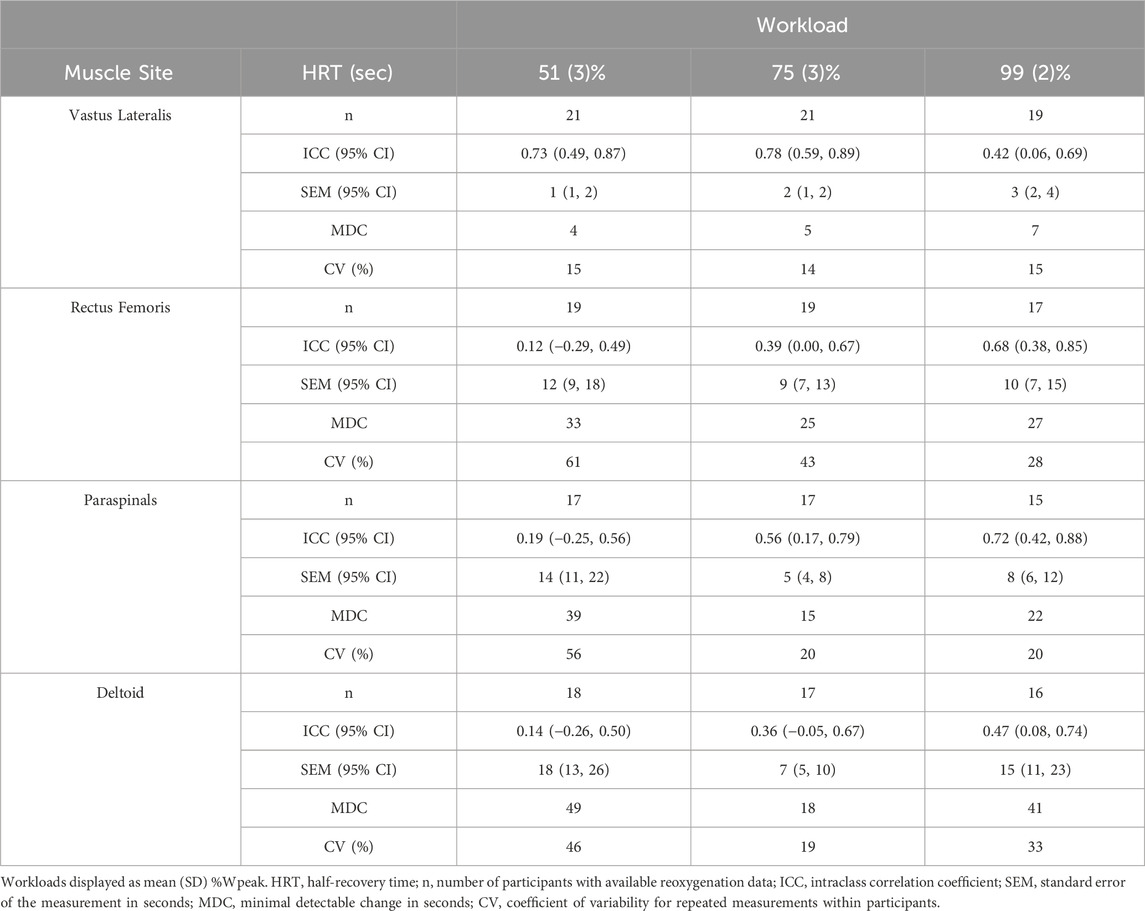
Table 5. Reoxygenation reliability and within-participant agreement at 50%, 75%, and 100% peak workload.
4 Discussion
This study aimed to describe and compare NIRS reoxygenation in multiple muscle sites, as a function of increasing exercise intensity during an incremental multi-stage cycling protocol with a wearable NIRS device (Moxy monitor). The main findings were that reoxygenation was slower (HRT was greater) with increasing exercise intensity in locomotor VL and RF muscles, and fastest in the primary locomotor VL compared to the RF and accessory PS and DL muscle sites. Descriptively, the between-participant variability tended to be lowest and test-retest reliability tended to be highest for the VL compared to other muscle sites, although variability was not statistically compared.
4.1 Locomotor reoxygenation across exercise intensity
Reoxygenation was slower with increasing workload in locomotor muscle sites (VL and RF) across the observed intensity range. This was consistent with our first hypothesis. However, reoxygenation in accessory muscles PS and DL was more variable overall and tended to be less sensitive to changes in workload.
Zuccarelli et al. (2020) recorded reoxygenation kinetics of deoxy[HbMb] in the VL after cycling exercise and found that reoxygenation was faster after a moderate intensity work bout compared to either a heavy intensity work bout or a maximal incremental test. There was no difference between the heavy and maximal intensity reoxygenation, whereas we observed differences between the analogous 75% and 100% workloads (Table 3). This discrepancy could be related to cycling protocol, where the moderate, heavy, and maximal incremental work bouts were performed on different days in the previous study, whereas we observed them during a single incremental protocol in the present experiment.
Previous experiments have shown equivocal findings regarding muscle recovery after exercise at different intensities: The kinetics of [PCr] resynthesis appear to be unchanged with exercise intensity, while inorganic phosphate ([Pi]) recovers more slowly after higher intensity exercise (Yoshida and Watari, 1993; Rossiter et al., 2002). m
The experiment from Zuccarelli et al. (2020) simultaneously monitored m
Koga et al. (2017) also measured deoxy[HbMb] reoxygenation in the VL and RF after cycling in the heavy intensity domain. They observed that reoxygenation kinetics in the VL were faster than in the RF, consistent with our findings. The mean response time (MRT) of a monoexponential function had mean (SD) values of 29 (10) s in the VL, and 77 (37) s in the RF. These are somewhat slower than the median (IQR) HRT values observed in the present study at the analogous 75% Wpeak, of 12 (3) s and 20 (14) s in the VL and RF, respectively (Table 2). MRT represents the time to recover approximately 63% of the reoxygenation amplitude, whereas HRT is calculated at 50%, and so MRT is expected to be larger. Even accounting for this, the faster reoxygenation observed in the present study could also be explained by participant fitness level: we evaluated trained and competitive cyclists, while the previous study investigated untrained participants (Koga et al., 2017).
Reoxygenation kinetics have been shown to be faster in individuals with higher fitness (Ichimura et al., 2006) and after endurance training (Puente-Maestu et al., 2003) as a consequence of enhanced adaptations toward better and faster matching of m
We did not correlate our findings to athlete fitness level, and so future research should clarify not only whether reoxygenation kinetics are enhanced, but whether the reliability of reoxygenation measurements also changes with training and fitness. Additionally, reoxygenation in non-locomotor muscle may interact differently with fitness (e.g., kinetics may be slower or unchanged with intensity) as blood flow is prioritised toward locomotor muscle sites and other critical tissues (Ogata et al., 2007; Laughlin et al., 2012; Osawa et al., 2017).
4.2 Comparison between muscle sites
Reoxygenation was fastest in the VL muscle and sequentially slower in the RF, PS, and DL, consistent with our second hypothesis. The VL is more involved than the RF during cycling, as demonstrated by muscle contraction activity measured with electromyography (EMG) and deoxygenation with NIRS (Hug and Dorel, 2009; Chin et al., 2011; Iannetta et al., 2017). The biarticular RF is described as having more of a secondary role through the pedal cycle to accelerate the pedal over top dead centre, while the uniarticular VL strongly activates during the pushing phase (Hug and Dorel, 2009). The RF is less efficient and has greater variability in power production between athletes than the VL (Hug and Dorel, 2009; Blake and Wakeling, 2015), which may explain the higher variability observed in the present study. It is difficult to isolate m
Osawa et al. (2017) observed slower reoxygenation in the upper extremity biceps brachii (BB) compared to the VL after 30 s high intensity cycling intervals, consistent with the present results in the DL. They also observed wider variability between participants at the BB compared to VL. Slower reoxygenation and wider variability could be explained by differences in recruitment of the upper extremity for stabilisation during cycling, particularly at higher workloads (Ozyener et al., 2012). However, deoxygenation is observed in the upper extremity even when the arm is supported and m
Data are sparse on the NIRS responses of the spinal erector muscles during cycling. Kell and Bhambhani (2007) recorded NIRS in the lumbar paraspinals during a horizontal isometric stabilisation task to maximal tolerance and recovery. They observed reoxygenation with a mean (SD) HRT of 35 (22) s. This is comparable to our median (IQR) of 39 (19) s after 100% workload. EMG activity of the spinal erector muscles increases in proportion to cycling workload, as they contribute to stabilisation and efficient energy transfer to pedalling (Muyor et al., 2022). While the contractile forces likely differ between an isometric hold and rhythmic pedalling, the similar reoxygenation time course observed after these tasks may indicate a common matching of m
The sequence in the time course of reoxygenation observed across the four sites investigated, representing primary and secondary locomotor, postural stabiliser, and upper extremity muscles, points toward the prioritisation of more metabolically active tissues and systemic redirection of blood flow and O2 delivery to meet those metabolic demands. The variability observed in the non-locomotor muscle sites could simply be biological noise, or it could help to understand how systemic responses arise from an aggregation of heterogenous local responses.
4.3 Test-retest reliability and within-participant agreement
Relative reliability was evaluated as ICC and represents the proportion of measurement error attributable to, e.g., biological variability and instrument error, compared to the variability observed between individuals (Atkinson and Nevill, 1998; Weir, 2005). ICC was poor-to-good for the four muscle sites investigated at 50%, 75%, and 100% workloads (Table 5). While the RF and PS had moderate reliability at higher workloads, the VL had good reliability at lower workloads and poor reliability after 100% Wpeak. The DL reliability was poor at all workloads. Poor ICC suggests that not only was there high variability in HRT between participants, but the variability in repeated measurements between the two trials was even greater. This is also demonstrated by the high SEM and CV values (low within-participant agreement) for repeated measurements (Table 5). SEM represents a range of values an individual might see with repeated testing day-to-day, while MDC represents a minimum change that would need to be seen to confidently attribute that change to a particular intervention rather than chance alone (Atkinson and Nevill, 1998; Weir, 2005). Given that the participants in this study represented a heterogenous group of mixed sex, fitness levels, tissue composition, and training histories, these values may be larger than those expected for a more homogenous group, such as athletes at the same competitive level.
Reoxygenation was most consistent between trials in the VL, with SEM of 1–3 s and MDC 4–7 s across cycling workloads. While MDC does not imply a minimal clinically important difference between participants, a lower measurement error in general will improve the ability to detect meaningful differences between clinical groups or in athletic performances (Atkinson and Nevill, 1998). Previous studies in healthy participants have found mean differences between groups for reoxygenation kinetics of 7–16 s related to age and fitness (Ichimura et al., 2006; Marwood et al., 2011) and 12–20 s after endurance training (Puente-Maestu et al., 2003). Even larger differences are observed in clinical evaluation, e.g., 7–30 s between healthy cyclists and cyclists with a sport-related vascular condition limiting blood flow to the legs (van Hooff et al., 2022a; van Hooff et al., 2022b). Differences of approximately 15 s are observed in patients with mitochondrial myopathy compared to healthy controls (Bravo et al., 2012), and within-participant changes of 20 s are observed in patients with peripheral vascular disease before vs. after exercise training (Baker et al., 2017). These clinically important differences fall well outside the MDC found in the present study, which is encouraging for the use of reoxygenation in the VL to detect meaningful differences between performance groups and after training interventions.
van Hooff et al. (2022b) reported reoxygenation kinetics in the VL after two trials of maximal incremental cycling in healthy cyclists and in patients with blood flow limitations in the iliac arteries (FLIA). They observed mean (SD) HRT values of approximately 22 (13) s for healthy athletes and 30 (12) s for patients with FLIA. For healthy athletes, this is comparable to the median (IQR) HRT of 17 (4) s observed in the present study (Table 2). They also reported test-retest absolute bias (95% limits of agreement) of −1.2 (18.7) s for healthy athletes and 3.4 (20.3) s for patients, with ICC of 0.59 and 0.64 for each group, respectively. These ICC values are higher than that observed in the present study after 100% workload, however the limits of agreement are also considerably larger than the 4–7 s presently observed for MDC (Table 5). Higher ICC despite larger within-participant variability as observed by van Hooff et al. (2022b) can be explained by relatively greater between-participant variability, compared to the present findings. These discrepancies could be related to the different protocol and NIRS device used, or to a wider heterogeneity between participants in the previous study, which recruited recreational, competitive, and professional athletes. On the other hand, the similar mean HRT values observed between these experiments suggests that different NIRS devices may return similar reoxygenation kinetics, whereas the same two devices report very different ‘absolute’ %SmO2 values (McManus et al., 2018). However, a direct comparison of reoxygenation kinetics between these devices would be valuable to understand the potential sources of signal variability.
4.4 Applications of reoxygenation kinetics
We now understand that postexercise reoxygenation is dependent on both metabolic O2 exchange (i.e., m
The present findings suggest that postexercise reoxygenation in the VL has satisfactory test-retest reliability to be used for monitoring longitudinal training effects and to detect cross-sectional differences between groups. Recent work in trained cyclists with FLIA has found that post-maximal exercise reoxygenation in the VL is discriminative of blood flow and O2 delivery limitations between FLIA patients and healthy athletes, and even more subtle differences between patients’ affected and unaffected limbs (van Hooff et al., 2022a). Thus, VL reoxygenation appears to be sensitive enough to detect pathological local imbalances in O2 delivery and O2 uptake. We observed higher reliability in the VL at 50% and 75% Wpeak than at 100% workload, raising the possibility that submaximal reoxygenation may be even more discriminative or predictive of individual performance differences than after maximal exercise. Interestingly, Beckitt et al. (2012) observed that after endurance training, patients with peripheral vascular disease had faster reoxygenation in the gastrocnemius after submaximal exercise, but not after the now higher maximal exercise level attained post-training. This was suggested to reflect an increased work capacity with no change in oxygen debt, facilitated by improved matching of m
Reliability was low for the RF, PS, and DL suggesting that monitoring these muscle sites alone is not appropriate for detecting longitudinal changes. However, combining individual observations of multiple muscle sites may reveal more about systemic changes not captured by the primary locomotor muscle. For example, there are individual differences expected in SmO2 values between left and right legs (Skotzke et al., 2024), and yet during exercise onset, averaging the NIRS kinetics from left and right VL corresponded to systemic
4.5 Limitations
Adipose tissue thickness and SKF influence SmO2 values and reoxygenation rates (slopes) (Niemeijer et al., 2017; McManus et al., 2018; Keller et al., 2023), however the time course of NIRS responses are expected to be less sensitive to superficial tissue layers (Hamaoka and McCully, 2019). SKF is recommended to be no greater than ½ the inter-optode distance for NIRS research (Barstow, 2019), which was 25 mm (SKF cut-off of 12.5 mm) in the present study. However, no exclusions were made based on skinfolds to prioritise ecological validity for a heterogenous population of male and female competitive cyclists. Seven participants (7 F) had SKF >12.5 mm in the VL, nine (8 F) in the RF, and seven (5 F) in the DL (SKF at the PS was not recorded). The study from van Hooff et al. (2022b) excluded participants with SKF >15 mm at the VL, and yet they still observed greater variability than we observed in the present findings. We investigated the effects of SKF by including it as an additional fixed factor in the linear mixed-effects model, however SKF explained only an additional 5% of the total variance (conditional R2 = 0.581 vs. 0.529 for the chosen model), therefore it was not included in the chosen model. However, future research should more thoroughly investigate the effects of SKF on reoxygenation kinetics, which may have interactions with sex and exercise intensity.
In the present study, the DL, and to a lesser extent PS reoxygenation were visibly delayed compared to the locomotor muscle sites, such that reoxygenation continued beyond the 60-s rest period between work intervals. Whereas the locomotor muscles reached their peak values within the passive rest periods. For this reason, the recovery window used to model reoxygenation was extended for the two accessory muscles to include the first 60 s during the subsequent work interval, where PS and DL were observed to eventually reach their peak reoxygenation values and subsequently begin to deoxygenate. At the start of the subsequent work stage, restarting pedalling and the ‘muscle pump’ effect of rhythmic contractions may have increased venous return from the lower extremity and facilitated further reoxygenation in the accessory muscles compared to passive rest (Laughlin et al., 2012). This may have contributed to the greater variability observed in the accessory muscles between and within participants (Tables 2, 5).
Finally, reoxygenation kinetics are commonly described by the MRT of a monoexponential function, however over 25% of our data did not conform to monoexponential kinetics and would have been lost if we restricted our analysis to this method. Therefore, estimating reoxygenation non-parametrically with half-recovery time was a compromise to compare across our heterogenous dataset from different muscles and exercise intensities. Whereas MRT describes and can be used to predict the shape of the response, HRT is a descriptive estimate of reoxygenation and may not represent the same underlying physiological phenomena. Therefore, caution must be advised when comparing HRT values, for example, between different muscle sites. However, the results were not meaningfully different from either HRT or MRT (available in the Supplementary Material), therefore we believe our interpretations are consistent with either calculation method. An advantage of HRT is that it can be easily manually calculated by practitioners without advanced processing or coding. However, we encourage practitioners and researchers to carefully consider the limitations of using any single parameter to describe the complex physiological phenomena occurring during reoxygenation. Future research should develop empirically derived and mechanistically robust equations to describe muscle oxygenation behaviours during exercise onset and recovery.
4.6 Conclusion
Our main objective was to describe the behaviour and the variability of reoxygenation with wearable NIRS in four muscle sites (locomotor VL and RF, accessory PS and DL) as exercise intensity increased during an incremental multi-stage cycling protocol. We observed that reoxygenation was generally slower with increasing workload in VL, RF, and PS, but that DL was not sensitive to exercise intensity. The primary locomotor VL muscle tended to have the fastest reoxygenation at all workloads followed sequentially by the more proximal muscle sites in the order VL < RF < PS < DL. Between-participant variability was lowest and within-participant reliability was highest in the VL, whereas the RF showed the highest variability and lowest reliability. Of the four muscles evaluated, only the VL appeared to have sufficiently high test-retest reliability to be used alone for monitoring change with training interventions or for detecting differences between groups. Future research should investigate whether observing NIRS responses across multiple muscle sites together may be useful to help explain day-to-day variations in performance and training responses.
Data availability statement
The raw data supporting the conclusions of this article will be made available by the authors, without undue reservation.
Ethics statement
The studies involving humans were approved by The University of British Columbia Clinical Research Ethics Board. The studies were conducted in accordance with the local legislation and institutional requirements. The participants provided their written informed consent to participate in this study.
Author contributions
JA: Conceptualization, Formal Analysis, Investigation, Methodology, Visualization, Writing–original draft, Writing–review and editing. AY: Conceptualization, Investigation, Methodology, Writing–review and editing. HN: Methodology, Project administration, Writing–review and editing. MH: Writing–review and editing. MK: Funding acquisition, Project administration, Supervision, Writing–review and editing.
Funding
The author(s) declare that financial support was received for the research, authorship, and/or publication of this article. This study was funded by a Natural Sciences and Engineering Research Council Discovery Grant and an equipment grant from the British Columbia Sport and Exercise Medicine Research Foundation.
Conflict of interest
The authors declare that the research was conducted in the absence of any commercial or financial relationships that could be construed as a potential conflict of interest.
Publisher’s note
All claims expressed in this article are solely those of the authors and do not necessarily represent those of their affiliated organizations, or those of the publisher, the editors and the reviewers. Any product that may be evaluated in this article, or claim that may be made by its manufacturer, is not guaranteed or endorsed by the publisher.
Supplementary material
The Supplementary Material for this article can be found online at: https://www.frontiersin.org/articles/10.3389/fphys.2024.1449384/full#supplementary-material
References
Adami A., Rossiter H. B. (2018). Principles, insights, and potential pitfalls of the noninvasive determination of muscle oxidative capacity by near-infrared spectroscopy. J. Appl. Physiol. (1985) 124 (1), 245–248. doi:10.1152/japplphysiol.00445.2017
Atkinson G., Nevill A. M. (1998). Statistical methods for assessing measurement error (reliability) in variables relevant to sports medicine. Sports Med. 26 (4), 217–238. doi:10.2165/00007256-199826040-00002
Baker W. B., Li Z., Schenkel S. S., Chandra M., Busch D. R., Englund E. K., et al. (2017). Effects of exercise training on calf muscle oxygen extraction and blood flow in patients with peripheral artery disease. J. Appl. Physiol. (1985) 123 (6), 1599–1609. doi:10.1152/japplphysiol.00585.2017
Baltrūnas T., Mosenko V., Mackevičius A., Dambrauskas V., Ašakienė I., Ručinskas K., et al. (2021). The use of near-infrared spectroscopy in the diagnosis of peripheral artery disease: a systematic review. Vascular 0 (0), 715–727. doi:10.1177/17085381211025174
Bangsbo J., Hellsten Y. (1998). Muscle blood flow and oxygen uptake in recovery from exercise. Acta Physiol. Scand. 162 (3), 305–312. doi:10.1046/j.1365-201x.1998.0331e.x
Barstow T. J. (2019). Understanding near infrared spectroscopy and its application to skeletal muscle research. J. Appl. Physiol. 126 (5), 1360–1376. doi:10.1152/japplphysiol.00166.2018
Bates D., Mächler M., Bolker B., Walker S. (2015). Fitting linear mixed-effects models using lme4. J. Stat. Softw. 67 (1), 1–48. doi:10.18637/jss.v067.i01
Batterson P. M., Norton M. R., Hetz S. E., Rohilla S., Lindsay K. G., Subudhi A. W., et al. (2020). Improving biologic predictors of cycling endurance performance with near-infrared spectroscopy derived measures of skeletal muscle respiration: E pluribus unum. Physiol. Rep. 8 (2), e14342. doi:10.14814/phy2.14342
Beckitt T. A., Day J., Morgan M., Lamont P. M. (2012). Calf muscle oxygen saturation and the effects of supervised exercise training for intermittent claudication. J. Vasc. Surg. 56 (2), 470–475. doi:10.1016/j.jvs.2011.11.140
Beever A. T., Tripp T. R., Zhang J., MacInnis M. J. (2020). NIRS-derived skeletal muscle oxidative capacity is correlated with aerobic fitness and independent of sex. J. Appl. Physiol. (1985) 129 (3), 558–568. doi:10.1152/japplphysiol.00017.2020
Behnke B. J., Ferreira L. F., McDonough P. J., Musch T. I., Poole D. C. (2009). Recovery dynamics of skeletal muscle oxygen uptake during the exercise off-transient. Respir. Physiol. Neurobiol. 168 (3), 254–260. doi:10.1016/j.resp.2009.07.013
Belardinelli R., Barstow T. J., Nguyen P., Wasserman K. (1997). Skeletal muscle oxygenation and oxygen uptake kinetics following constant work rate exercise in chronic congestive heart failure. Am. J. Cardiol. 80 (10), 1319–1324. doi:10.1016/S0002-9149(97)00672-3
Blake O. M., Wakeling J. M. (2015). Muscle coordination limits efficiency and power output of human limb movement under a wide range of mechanical demands. J. Neurophysiol. 114 (6), 3283–3295. doi:10.1152/jn.00765.2015
Bravo D. M., Gimenes A. C., Nascimento R. B., Ferreira E. V. M., Siqueira A. C. B., Meda E. D. S., et al. (2012). Skeletal muscle reoxygenation after high-intensity exercise in mitochondrial myopathy. Eur. J. Appl. Physiol. 112 (5), 1763–1771. doi:10.1007/s00421-011-2136-4
Buchheit M., Ufland P. (2011). Effect of endurance training on performance and muscle reoxygenation rate during repeated-sprint running. Eur. J. Appl. Physiol. 111 (2), 293–301. doi:10.1007/s00421-010-1654-9
Caldwell A. R. (2022). SimplyAgree: an R package and jamovi module for simplifying agreement and reliability analyses. J. Open Source Softw. 7 (71), 4148. doi:10.21105/joss.04148
Chin L. M., Kowalchuk J. M., Barstow T. J., Kondo N., Amano T., Shiojiri T., et al. (2011). The relationship between muscle deoxygenation and activation in different muscles of the quadriceps during cycle ramp exercise. J. Appl. Physiol. 111 (5), 1259–1265. doi:10.1152/japplphysiol.01216.2010
Clarsen B., Krosshaug T., Bahr R. (2010). Overuse injuries in professional road cyclists. Am. J. Sports Med. 38 (12), 2494–2501. doi:10.1177/0363546510376816
Cornelis N., Chatzinikolaou P., Buys R., Fourneau I., Claes J., Cornelissen V. (2021). The use of near infrared spectroscopy to evaluate the effect of exercise on peripheral muscle oxygenation in patients with lower extremity artery disease: a systematic review. Eur. J. Vasc. Endovasc. Surg. 61 (5), 837–847. doi:10.1016/j.ejvs.2021.02.008
Crum E. M., O'Connor W. J., Van Loo L., Valckx M., Stannard S. R. (2017). Validity and reliability of the Moxy oxygen monitor during incremental cycling exercise. Eur. J. Sport Sci. 17 (8), 1037–1043. doi:10.1080/17461391.2017.1330899
Feldmann A., Schmitz R., Erlacher D. (2019). Near-infrared spectroscopy-derived muscle oxygen saturation on a 0% to 100% scale: reliability and validity of the Moxy Monitor. J. Biomed. Opt. 24 (11), 1–11. doi:10.1117/1.JBO.24.11.115001
Hamaoka T., McCully K. K. (2019). Review of early development of near-infrared spectroscopy and recent advancement of studies on muscle oxygenation and oxidative metabolism. J. Physiol. Sci. 69 (6), 799–811. doi:10.1007/s12576-019-00697-2
Hellsten Y., Nyberg M. (2015). Cardiovascular adaptations to exercise training. Compr. Physiol. 6 (1), 1–32. doi:10.1002/cphy.c140080
Hug F., Dorel S. (2009). Electromyographic analysis of pedaling: a review. J. Electromyogr. Kinesiol. 19 (2), 182–198. doi:10.1016/j.jelekin.2007.10.010
Iannetta D., Qahtani A., Millet G. Y., Murias J. M. (2017). Quadriceps muscles O2 extraction and EMG breakpoints during a ramp incremental test. Front. Physiol. 8, 686. doi:10.3389/fphys.2017.00686
Ichimura S., Murase N., Osada T., Kime R., Homma T., Ueda C., et al. (2006). Age and activity status affect muscle reoxygenation time after maximal cycling exercise. Med. Sci. Sports Exerc. 38 (7), 1277–1281. doi:10.1249/01.mss.0000227312.08599.f1
Kell R. T., Bhambhani Y. (2007). Relationship between erector spinae muscle oxygenation via in vivo near infrared spectroscopy and static endurance time in healthy males. Eur. J. Appl. Physiol. 102 (2), 243–250. doi:10.1007/s00421-007-0577-6
Keller J. L., Traylor M. K., Gray S. M., Hill E. C., Weir J. P. (2023). Sex differences in NIRS derived values of reactive hyperemia persist after experimentally controlling for the ischemic vasodilatory stimulus. J. Appl. Physiol. 1985, 3–14. doi:10.1152/japplphysiol.00174.2023
Kirby B. S., Clark D. A., Bradley E. M., Wilkins B. W. (2021). The balance of muscle oxygen supply and demand reveals critical metabolic rate and predicts time to exhaustion. J. Appl. Physiol. (1985) 130 (6), 1915–1927. doi:10.1152/japplphysiol.00058.2021
Koga S., Okushima D., Barstow T. J., Rossiter H. B., Kondo N., Poole D. C. (2017). Near-infrared spectroscopy of superficial and deep rectus femoris reveals markedly different exercise response to superficial vastus lateralis. Physiol. Rep. 5 (17), e13402. doi:10.14814/phy2.13402
Koo T. K., Li M. Y. (2016). A guideline of selecting and reporting intraclass correlation coefficients for reliability research. J. Chiropr. Med. 15 (2), 155–163. doi:10.1016/j.jcm.2016.02.012
Krustrup P., Jones A. M., Wilkerson D. P., Calbet J. A. L., Bangsbo J. (2009). Muscular and pulmonary O2 uptake kinetics during moderate- and high-intensity sub-maximal knee-extensor exercise in humans. J. Physiology 587 (8), 1843–1856. doi:10.1113/jphysiol.2008.166397
Kuznetsova A., Brockhoff P. B., Christensen R. H. B. (2017). lmerTest package: tests in linear mixed effects models. J. Stat. Softw. 82. doi:10.18637/jss.v082.i13
Langenfeld A., Wirth B., Scherer-Vrana A., Riner F., Gaehwiler K., Valdivieso P., et al. (2022). No alteration of back muscle oxygenation during isometric exercise in individuals with non-specific low back pain. Sci. Rep. 12 (1), 8306. doi:10.1038/s41598-022-11683-x
Laughlin M. H., Davis M. J., Secher N. H., Van Lieshout J. J., Arce-Esquivel A. A., Simmons G. H., et al. (2012). Peripheral circulation. Compr. Physiol. 2, 321–447. doi:10.1002/cphy.c100048
Lenth R., Bolker B., Buerkner P., Giné-Vázquez I., Herve M., Jung M., et al. (2023). Emmeans: estimated marginal means, aka least-squares means. R package version 1.8.8. Available at: https://CRAN.R-project.org/package=emmeans (Accessed October 05, 2023).
Marwood S., Roche D., Garrard M., Unnithan V. B. (2011). Pulmonary oxygen uptake and muscle deoxygenation kinetics during recovery in trained and untrained male adolescents. Eur. J. Appl. Physiol. 111 (11), 2775–2784. doi:10.1007/s00421-011-1901-8
McCully K. K., Iotti S., Kendrick K., Wang Z., Posner J. D., Leigh J. S., et al. (1994). Simultaneous in vivo measurements of HbO2 saturation and PCr kinetics after exercise in normal humans. J. Appl. Physiol. 77 (1), 5–10. doi:10.1152/jappl.1994.77.1.5
McManus C. J., Collison J., Cooper C. E. (2018). Performance comparison of the MOXY and PortaMon near-infrared spectroscopy muscle oximeters at rest and during exercise. J. Biomed. Opt. 23 (1), 1–14. doi:10.1117/1.JBO.23.1.015007
Messere A., Roatta S. (2013). Influence of cutaneous and muscular circulation on spatially resolved versus standard Beer-Lambert near-infrared spectroscopy. Physiol. Rep. 1 (7), e00179. doi:10.1002/phy2.179
Muyor J. M., Antequera-Vique J. A., Oliva-Lozano J. M., Arrabal-Campos F. M. (2022). Effect of incremental intensities on the spinal morphology and core muscle activation in competitive cyclists. Sports Biomech. 22, 597–620. doi:10.1080/14763141.2022.2097945
Niemeijer V. M., Jansen J. P., Van Dijk T., Spee R. F., Meijer E. J., Kemps H. M. C., et al. (2017). The influence of adipose tissue on spatially resolved near-infrared spectroscopy derived skeletal muscle oxygenation: the extent of the problem. Physiol. Meas. 38 (3), 539–554. doi:10.1088/1361-6579/aa5dd5
Ogata H., Arimitsu T., Matsuura R., Yunoki T., Horiuchi M., Yano T. (2007). Relationship between oxygenation in inactive biceps brachii muscle and hyperventilation during leg cycling. Physiol. Res. 56 (1), 57–65. doi:10.33549/physiolres.930888
Okushima D., Poole D. C., Barstow T. J., Kondo N., Chin L. M. K., Koga S. (2020). Effect of differential muscle activation patterns on muscle deoxygenation and microvascular haemoglobin regulation. Exp. Physiol. 105 (3), 531–541. doi:10.1113/ep088322
Osawa T., Shiose K., Takahashi H. (2017). Delayed onset of reoxygenation in inactive muscles after high-intensity exercise. Adv. Exp. Med. Biol. 977, 255–260. doi:10.1007/978-3-319-55231-6_35
Ozyener F., Whipp B. J., Ward S. A. (2012). The contribution of "resting" body muscles to the slow component of pulmonary oxygen uptake during high-intensity cycling. J. Sports Sci. Med. 11 (4), 759–767.
Paquette M., Bieuzen F., Billaut F. (2020). Effect of a 3-weeks training camp on muscle oxygenation, VO2 and performance in elite sprint kayakers. Front. Sports Act. Living 2, 47. doi:10.3389/fspor.2020.00047
Perrey S., Quaresima V., Ferrari M. (2024). Muscle oximetry in sports science: an updated systematic review. Sports Med. 54, 975–996. doi:10.1007/s40279-023-01987-x
Pilotto A. M., Adami A., Mazzolari R., Brocca L., Crea E., Zuccarelli L., et al. (2022). Near-infrared spectroscopy estimation of combined skeletal muscle oxidative capacity and O2 diffusion capacity in humans. J. Physiology 600, 4153–4168. doi:10.1113/jp283267
Puente-Maestu L., Tena T., Trascasa C., Pérez-Parra J., Godoy R., García J. M., et al. (2003). Training improves muscle oxidative capacity and oxygenation recovery kinetics in patients with chronic obstructive pulmonary disease. Eur. J. Appl. Physiol. 88 (6), 580–587. doi:10.1007/s00421-002-0743-9
R Core Team (2021). R: a language and environment for statistical computing. Vienna, Austria: R Foundation for Statistical Computing. Available at: https://www.R-project.org/(Accessed February 29, 2024).
Reinpõld K., Rannama I. (2023). Oxygen uptake and bilaterally measured vastus lateralis muscle oxygen desaturation kinetics in well-trained endurance cyclists. J. Funct. Morphol. Kinesiol. 8 (2), 64. doi:10.3390/jfmk8020064
Ritchie E. D., Vogels S., Van Dongen T. T. C. F., Borger Van Der Burg B., Scheltinga M. R., Zimmermann W. O., et al. (2022). Systematic review of innovative diagnostic tests for chronic exertional compartment syndrome. Int. J. Sports Med. 44, 20–28. doi:10.1055/a-1866-5957
Rossiter H. B., Ward S. A., Kowalchuk J. M., Howe F. A., Griffiths J. R., Whipp B. J. (2002). Dynamic asymmetry of phosphocreatine concentration and O2 uptake between the on- and off-transients of moderate- and high-intensity exercise in humans. J. Physiol. 541 (3), 991–1002. doi:10.1113/jphysiol.2001.012910
Ryan T. E., Southern W. M., Reynolds M. A., McCully K. K. (2013). A cross-validation of near-infrared spectroscopy measurements of skeletal muscle oxidative capacity with phosphorus magnetic resonance spectroscopy. J. Appl. Physiol. (1985) 115 (12), 1757–1766. doi:10.1152/japplphysiol.00835.2013
Shiroishi K., Kime R., Osada T., Murase N., Shimomura K., Katsumura T. (2010). “Decreased muscle oxygenation and increased arterial blood flow in the non-exercising limb during leg exercise,” in Oxygen transport to tissue XXXI (Springer US), 379–384.
Simmons G. H., Wong B. J., Holowatz L. A., Kenney W. L. (2011). Changes in the control of skin blood flow with exercise training: where do cutaneous vascular adaptations fit in? Exp. Physiol. 96 (9), 822–828. doi:10.1113/expphysiol.2010.056176
Skotzke P., Schwindling S., Meyer T. (2024). Side differences and reproducibility of the Moxy muscle oximeter during cycling in trained men. Eur. J. Appl. Physiol. doi:10.1007/s00421-024-05514-2
Tripp T. R., McDougall R. M., Frankish B. P., Wiley J. P., Lun V., MacInnis M. J. (2023). Contraction intensity affects NIRS-derived skeletal muscle oxidative capacity but not its relationships to mitochondrial protein content or aerobic fitness. J. Appl. Physiol. 136, 298–312. doi:10.1152/japplphysiol.00342.2023
Van Beekvelt M. C. P., Shoemaker J. K., Tschakovsky M. E., Hopman M. T. E., Hughson R. L. (2001). Blood flow and muscle oxygen uptake at the onset and end of moderate and heavy dynamic forearm exercise. Am. J. Physiology-Regulatory, Integr. Comp. Physiology 280 (6), R1741–R1747. doi:10.1152/ajpregu.2001.280.6.R1741
van Hooff M., Arnold J., Meijer E., Schreuder P., Regis M., Xu L., et al. (2022a). Diagnosing sport-related flow limitations in the iliac arteries using near-infrared spectroscopy. J. Clin. Med. 11 (24), 7462. doi:10.3390/jcm11247462
van Hooff M., Meijer E. J., Scheltinga M. R. M., Savelberg H. H. C. M., Schep G. (2022b). Test–retest reliability of skeletal muscle oxygenation measurement using near-infrared spectroscopy during exercise in patients with sport-related iliac artery flow limitation. Clin. Physiol. Funct. Imaging. 42, 114–126. doi:10.1111/cpf.12738
Weir J. P. (2005). Quantifying test-retest reliability using the intraclass correlation coefficient and the SEM. J. Strength Cond. Res. 19 (1), 231–240. doi:10.1519/15184.1
Yogev A., Arnold J., Clarke D., Guenette J. A., Sporer B. C., Koehle M. S. (2022). Comparing the respiratory compensation point with muscle oxygen saturation in locomotor and non-locomotor muscles using wearable NIRS spectroscopy during whole-body exercise. Front. Physiol. 13, 818733. doi:10.3389/fphys.2022.818733
Yogev A., Arnold J., Nelson H., Clarke D. C., Guenette J. A., Sporer B. C., et al. (2023). Comparing the reliability of muscle oxygen saturation with common performance and physiological markers across cycling exercise intensity. Front. Sports Act. Living 5, 1143393. doi:10.3389/fspor.2023.1143393
Yoshida T., Watari H. (1993). 31P-Nuclear magnetic resonance spectroscopy study of the time course of energy metabolism during exercise and recovery. Eur. J. Appl. Physiol. Occup. Physiol. 66 (6), 494–499. doi:10.1007/bf00634298
Keywords: near-infrared spectroscopy, muscle oxygenation, muscle oxygen uptake, wearable, exercise testing, repeatability, re-oxygenation, recovery
Citation: Arnold JI, Yogev A, Nelson H, van Hooff M and Koehle MS (2024) Muscle reoxygenation is slower after higher cycling intensity, and is faster and more reliable in locomotor than in accessory muscle sites. Front. Physiol. 15:1449384. doi: 10.3389/fphys.2024.1449384
Received: 14 June 2024; Accepted: 22 July 2024;
Published: 14 August 2024.
Edited by:
Stephane Perrey, Université de Montpellier, FranceReviewed by:
Anton Ušaj, University of Ljubljana, SloveniaJoshua L. Keller, University of North Texas, United States
Aldo A. Vasquez-Bonilla, University of Extremadura, Spain
Copyright © 2024 Arnold, Yogev, Nelson, van Hooff and Koehle. This is an open-access article distributed under the terms of the Creative Commons Attribution License (CC BY). The use, distribution or reproduction in other forums is permitted, provided the original author(s) and the copyright owner(s) are credited and that the original publication in this journal is cited, in accordance with accepted academic practice. No use, distribution or reproduction is permitted which does not comply with these terms.
*Correspondence: Jem I. Arnold, amVtLmFybm9sZEB1YmMuY2E=