- 1MRC Unit for Lifelong Health and Ageing at UCL, Department of Population Science and Experimental Medicine, Institute for Cardiovascular Science, University College London, London, United Kingdom
- 2Department of Cardiology and Vascular Medicine, Sardjito Central Public Hospital, Gadjah Mada University, Yogyakarta, Indonesia
Background: Near-infrared spectroscopy (NIRS) provides a non-invasive, cost-effective method for assessing skeletal muscle oxidative capacity when combined with a short exercise protocol and arterial occlusions. However, the impact of different exercise protocols and reproducibility of the method in non-athletic adults have not previously been assessed.
Methods: Young, non-athletic adults (YA) were invited to perform a short duration, fast frequency contraction (SF) exercise protocol and a long duration slow frequency (LS) contraction protocol, combined with NIRS measurements and arterial occlusions to assess skeletal muscle oxidative capacity. YA and older non-athletic adults (OA; >65 years old) were invited to perform the SF exercise protocol twice to assess the reproducibility of this oxidative capacity measurement.
Results: We included 25 participants (14 male (56%), age range: 18–86 years) in the analyses. There was a strong positive correlation and good agreement between time constants derived following the SF and LS exercise protocols (Lin’s concordance correlation coefficient: 0.69, p-value < 0.001 mean bias [LoA]: −3.2 [−31.0, 24.4] seconds. There was a strong positive correlation and good agreement between time constants derived from the SF exercise protocol in the YA & OA group (Lin’s concordance correlation coefficient: 0.63, p-value < 0.001; mean bias [LoA] −6.4 [−34.0, 21.3] seconds).
Conclusion: These data provide evidence to suggest that NIRS is a reliable in vivo method for the assessment of skeletal muscle oxidative capacity irrespective of exercise protocol duration or muscle contraction frequency. NIRS-measured oxidative capacity via the SF exercise protocol was reproducible in non-athletic adults with a wide range in age.
Introduction
Skeletal muscle function declines with age and in a multitude of disease phenotypes potentially leading to reduced physical function, frailty and loss of independence (Marzetti and Leeuwenburgh, 2006; Gomes et al., 2017). Tracking pathophysiological alterations in skeletal muscle function is critical for understanding disease mechanisms, progression and response to intervention (Coen et al., 2019). Skeletal muscle oxidative capacity is a key feature, representing the overall performance of the muscle tissue to extract and utilize oxygen, which is known to decline with aging and in the presence of many diseases (Santanasto et al., 2016; Gonzalez-Freire et al., 2018; Trevino et al., 2019). There is an urgent need to develop cost-effective, non-invasive methods for assessing oxidative capacity in skeletal muscle.
Current methods for assessing skeletal muscle oxidative capacity include tissue biopsy for high resolution respirometry (HRR) and phosphorus magnetic resonance spectroscopy (31P-MRS) to capture Phosphocreatine (PCr) depletion and recovery and maximal rates of ATP production via oxidative phosphorylation (Lanza et al., 2010). Although HRR provides detailed mechanistic insight into mitochondrial bioenergetic pathways, the invasive nature of the biopsy can be uncomfortable for participants and requires clinical facilities and expertise. 31P-MRS is the reference-standard for directly measuring maximal rates of PCr recovery following a short bout of exercise, however, is limited to specialist centres that have access to expensive scanners.
Near-infrared spectroscopy (NIRS) offers an alternative, non-invasive and cost-effective technique for estimating skeletal muscle oxidative capacity in vivo. When combined with low intensity exercise and subsequent transient arterial occlusions, the recovery kinetics of local muscle oxygen consumption post-exercise provide an estimate of PCr pay-back (Ryan et al., 2012; Scheeren et al., 2012; Sumner et al., 2020). Despite cross-validation of NIRS with 31P-MRS demonstrating a strong correlation (Nagasawa et al., 2003; Ryan et al., 2013a; Ryan et al., 2014a; Jones et al., 2016), only limited work exists investigating the exercise protocols that can be applied when using NIRS (Ryan et al., 2013b). Prior 31P-MRS studies have applied exercise protocols of varying lengths and frequency of muscle contractions. McCully et al utilised a 5-minute exercise phase with plantar flexion muscle contractions every 4–5 s, whereas Sedivy et al used a 6-min exercise phase with plantar flexion every 2-s (McCully et al., 1993; McCully et al., 1994; Šedivý et al., 2015). In contrast, NIRS exercise protocols described in the literature are relatively short (10–30 s) and involve rapid, vigorous contractions (Ryan et al., 2012; Ryan et al., 2013a; Ryan et al., 2013b; Ryan et al., 2014b; Sumner et al., 2020; Menon et al., 2021). A comparison of recovery time constants derived following each of these approaches has not previously been described. A 10-second short-fast protocol was chosen as it is routinely used in NIRS exercise protocol studies and is a practical approach for the future application of this method in a clinical context. It is compared with the 5-minute long-slow protocol that is generally used for 31P-MRS studies as the standard for in-vivo direct measurement of PCr recovery rates. Furthermore, assessing the reproducibility of this method in non-athletic adults and older adults, where oxidative performance is an extremely useful health metric, would be a beneficial addition to the literature. The recruitment of adults of a wide age range would also contribute to the development of this method and its application to large population-based studies.
Thus, the objectives of this study were two-fold, (1) to compare NIRS-measured skeletal muscle oxidative capacity using two different exercise protocols, a short duration rapid muscle contraction protocol (short-fast) and long duration dispersed/controlled muscle contraction protocol (long-slow), in young healthy subjects and (2) to assess the reproducibility of NIRS-measured oxidative capacity in a non-athletic adult population with a wide age range.
Materials and methods
Study participants
Participants were either young, healthy adults (YA) recruited from the University College London student and staff pool and invited to attend a single research visit, or were older adults (OA), >65 years old, that had previously been enrolled in a longitudinal cohort study (the Southall and Brent Revisited, SABRE study) and were undergoing an oxidative capacity measure as part of a follow-up visit (Jones et al., 2020). All procedures were in accordance with the principles of the Helsinki declaration and all participants gave written informed consent. The study procedures were approved by the reviewer board of the UCL Research Ethics Committee (21787.001) for the YA and by the National Research Ethics Service (NRES) Committee London—North Fulham for the OA SABRE study. Participants were asked not to smoke, consume alcohol, or do moderate-to-vigorous physical activity in the 24 h prior to the testing.
Participant characteristics and anthropometrics
Year of birth, sex, and ethnicity were reported by the participant. Height was measured barefoot using a stadiometer (Seca 217; Seca, Hamburg, Germany) to the closest centimetre and weight was measured in kilograms using digital bio-impedance scales (BC-418; Tanita, IL, United States), to calculate body mass index (BMI).
Skeletal muscle NIRS measurements
Device and device placement
A portable continuous wave (CW) NIRS device (Portamon, Artinis Medical System, Netherlands) that measures oxy-haemoglobin (O2Hb) and deoxy-haemoglobin (HHb) changes at a sampling frequency of 10 Hz was used for all tests. Participants were invited to recline in a semi-supine position on a medical examination couch to reduce hemodynamic variability and optimize cardiovascular exercise adaptation (Kubota et al., 2015; Kubota et al., 2017). The small, wireless CW NIRS device was placed on the skin overlaying the left gastrocnemius muscle, held in position by micropore tape and covered with a neoprene sleeve to avoid ambient light contamination (Figure 1). The neoprene sleeve was included as part of the Artinis Medical System Portamon NIRS equipment kit and used in line with manufacturer guidelines. The leg was supported by cushioned pads placed beneath the knee and ankle. A rapidly inflatable cuff was wrapped around the leg proximal to the NIRS device above the knee and connected to a rapid cuff inflation system (Hokanson Companies, United States). A resistance band was secured around the ball of the left foot and the opposite end secured to a Velcro waistband to avoid arm fatigue during the exercise protocols or changes in tension throughout the exercise (Figure 1). The length of the resistance band was adjusted by the technician so that there was light tension in the dorsi-flexed foot position to account for differences in participant leg length.
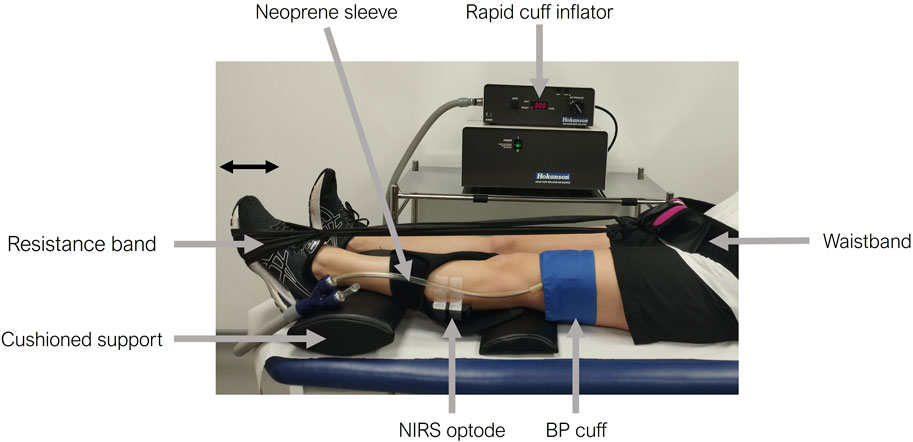
Figure 1. Experimental set-up for the skeletal muscle near-infrared spectroscopy (NIRS) measurements. The participant’s leg is supported with cushioned pads under the knee and ankle. A NIRS optode is positioned on the skin overlaying the gastrocnemius of the left calf, secured with micropore tape, and covered by a black neoprene sleeve. A rapidly inflatable cuff is placed above the knee (proximal to the NIRS optode) and is connected to a rapid cuff inflator. A resistance band is then placed around the participant’s foot and secured using a waistband. The participant’s foot is shown pushing against the resistance band in the plantar flexion position.
Participants were permitted to push against the resistance band to familiarize themselves with the exercise action (plantar flexion) before resting in the baseline position. Baseline NIRS signals were acquired for at least a minute, or until stable. A resting arterial occlusion was performed for 30 s using a cuff pressure of at least 275 mmHg followed by recovery until NIRS signals returned to baseline. During the cuff inflation, visual inspection of the NIRS traces was performed to check for loss of pulsatility and reciprocity in the oxy-Hb and deoxy-Hb signals. If a complete arterial occlusion was not achieved, participants were excluded from subsequent analyses. Participants were also asked if they could tolerate the cuff inflation at the given pressure (275 mmHg).
Exercise protocols
Comparison of NIRS measured skeletal muscle oxidative capacity exercise protocols
Each participant was invited to complete two exercise protocols in series, the order of which was randomised by alternate allocation. Firstly, a “short-fast” protocol in which participants were asked to perform rapid plantar flexion against the resistance band as many times as possible for a period of 10-s and secondly, a “long-slow” exercise protocol in which participants were asked to perform 5 min of plantar flexion against a resistance band at a rate of 30 plantar flexions a minute in response to a metronome. Following each exercise protocol, short transient arterial occlusions lasting 5–8 s were applied over 3 min (5 s in the first minute and 8 s in the second and third minutes) to track recovery musVO2 and measure the recovery time constant (τ), an estimate of oxidative capacity (Ryan et al., 2013c; Ryan et al., 2014b; Southern et al., 2014). Longer values of τ represent poorer skeletal muscle oxidative capacity (Ryan et al., 2013c). An additional 2 min recovery period was given between each protocol.
Flow-charts illustrating the exercise protocols are presented in the supplementary information file (Supplementary Figures S1, S2).
Reproducibility of NIRS measured skeletal muscle oxygen consumption and oxidative capacity
After stabilisation of the trace, two resting arterial occlusions were imposed by rapidly inflating the cuff to at least 275 mmHg for 30 s on each occasion (ensuring stabilisation of the trace in between occlusions with an interval of approximately 30 s). Local skeletal muscle oxygen consumption (musVO2) was estimated from the change in oxy- and deoxy-Hb (Van Beekvelt et al., 2001). Each participant was invited to complete two short-fast exercise protocols in series in which participants were asked to perform rapid plantar flexion against the resistance band as many times as possible for a period of 10-s. Following each exercise protocol, short transient arterial occlusions were applied over 3 min as described above. An additional 2 min recovery period was given between each protocol.
Adipose tissue thickness
Adipose tissue thickness (ATT) was measured at the NIRS measurement site using B-mode ultrasound (Philips EPIQ 7G Ultrasound System, Netherlands) by four experienced technicians who were involved in data capture. Three ATT measurements were recorded for each participant and averaged.
Data processing
NIRS data were processed using custom written scripts in MATLAB R2021a (The MathWorks, United States) (Ryan et al., 2012). All processing was performed by one experienced technician. For each participant, all traces were plotted and assessed by eye for the presence of artifacts or incomplete arterial occlusion. Incomplete arterial occlusions were identified by pulsatility in the NIRS signals, a lack of reciprocity of the oxy-Hb and deoxy-Hb signals, or noise in the signal whereby the downward slope of the oxy-Hb signal could not be visualised during cuff inflation. The start of the resting arterial occlusion and each intermittent arterial occlusion was selected by eye, all further processing was automated.
Muscle oxygen consumption (musVO2) was measured as the downward slope of the O2Hb to HHb difference signal during each occlusion using at least 40 data points (4 s). The difference signal was used to account for potential shifts in blood volume into the region of interrogation during the cuff inflation (Ryan et al., 2012). The time constant (τ) was calculated using the repeated post-exercise musVo2 measurements from the transient occlusions. These were fit to a mono-exponential curve, visually inspected and only curves with a good fit (r2 > 0.70) were included in subsequent analyses. A sensitivity analysis was performed using thresholds of r2 > 0.60 and r2 > 0.65, however, the pattern of results remained similar. The time constant for recovery indicating oxidative capacity was derived from the fit as described previously (Ryan et al., 2014b).
Statistical analysis
Statistical analysis was performed with STATA MP17 (StataCorp, United States). Categorical descriptive data are presented as n (%) and continuous variables are presented as mean ± standard deviation (SD) if normally distributed or as median [interquartile range, IQR] if skewed. The Shapiro-Wilk test was used to formally test the assumption of data normality and data were visualised with histograms. Correlations were assessed using Pearson`s or Spearman`s correlation coefficient, depending on data normality. Lin’s concordance correlation coefficients (CCC) and Bland-Altman plots [presented as mean bias (Limits of Agreement; LoA)] were used to assess the level of agreement between measurements derived from the short-fast and long-slow exercise protocols and between measurements derived from test 1 versus test 2 of the short-fast exercise protocol in the reproducibility study. For resting and end-exercise musVO2 assessment, the negative slope values were converted to positive values (multiplying by −1) to simplify the analysis and interpretation. The level of significance was set at p < 0.05.
Results
In total, 16 YA were recruited for the exercise protocol comparison study. In 3 YA participants, time constants for the recovery of mus
Twenty eight participants (14 YA and 14 OA) were recruited for the reproducibility study. In three participants, time constants for the recovery of musVO2 were excluded from summary analyses due to mono-exponential fits not exceeding our quality inclusion criteria (r2 > 0.70). We excluded two additional participants from the reproducibility of resting arterial occlusion assessment due to either an observed positive change in O2-Hb during the resting occlusion, indicating that an arterial occlusion had not been achieved. Therefore, 25 of the 28 participants recruited were included in the time constant reproducibility analysis and 23 participants were included in the resting musVO2 reproducibility analysis, respectively.
Participant characteristics
13 YA (9 male (70%), age 26 ± 3 years old) were included in the summary analyses for the exercise protocol comparison and 25 adult participants [14 male (56%), age 59 (26, 78) years old] were included in the summary analyses for the reproducibility study. YA participants in the exercise protocol comparison study were predominantly of self-reported Asian ethnicity (77%). By study design SABRE participants (OA included in the reproducibility analyses) were either first generation Indian Asian, African Caribbean or of White European ethnicity. This was because one of the primary research questions of the original SABRE study addresses health disparities by ethnic group in the UK (Jones et al., 2020). ATT was <1.5 cm in all participants. A summary of participant characteristics is presented in Table 1.
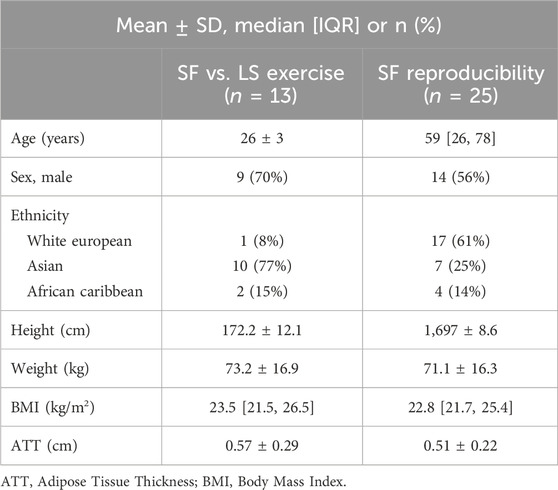
Table 1. Participant characteristics for 13 young adults included in the short-fast (SF) versus long-slow (LS) exercise protocol comparison and 25 adults included in the SF reproducibility study.
Short-fast versus long-slow exercise protocol
Representative time constant curves generated from the short-fast and long-slow exercise protocols for a YA participant are included in the supplementary information file (Supplementary Figures S3, S4). The mean time constants (τ) derived after the short-fast and long-slow exercise protocols for 12 participants were 35.1 ± 19.2 s and 38.4 ± 17.3 s, respectively. There was a strong positive correlation between the two measurements (r = 0.70, p-value = 0.011; Lin’s CCC = 0.69, p-value <0.001; Figure 2A). The bias was small without indication of systematic bias, but the limits of agreement were fairly wide [mean bias (LoA): −3.2 (−31.0, 24.4) seconds; Figure 2B].
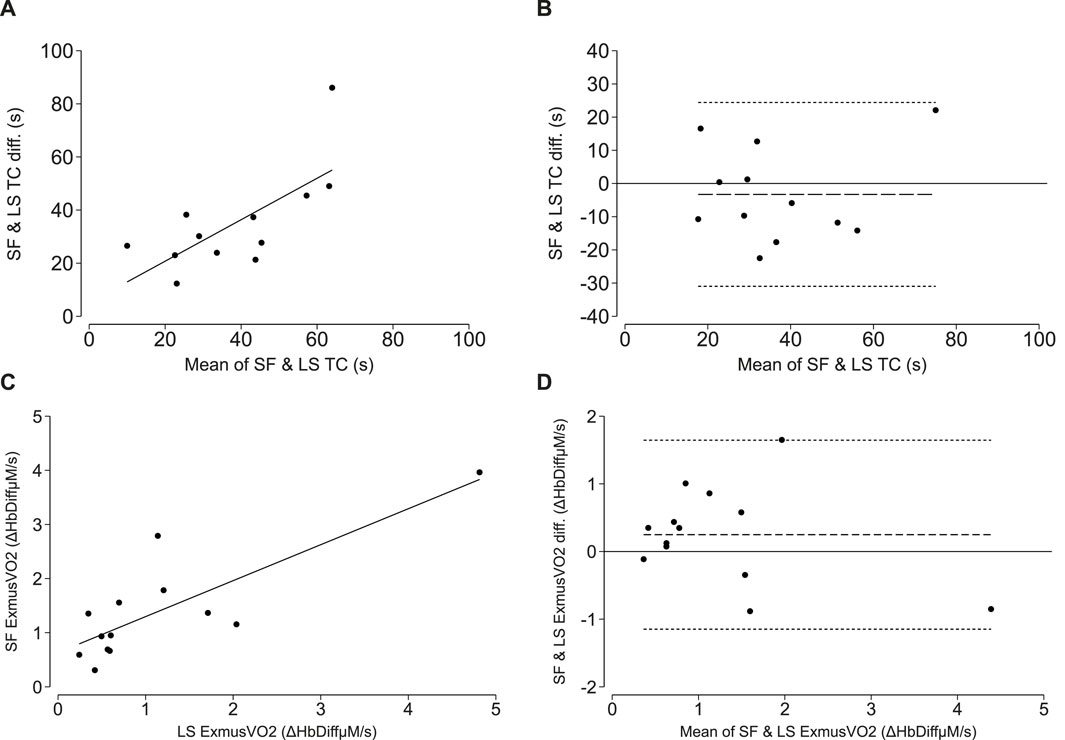
Figure 2. NIRS-measured oxidative capacity and end exercise muscle oxygen consumption (ExmusVO2) measure from short-fast (SF) and long-slow (LS) exercise protocols. (A, C) correlations between the SF and LS exercise protocol time constant (TC; τ) and SF and LS end exercise oxygen consumption. The line of best fit is plotted in solid black. (B, D) Bland-Altman plots demonstrating levels of agreement between the SF and LS time constant (TC; τ) and SF and LS end exercise oxygen consumption. The mean difference is plotted as the long-dashed line and the upper and lower limits of agreement are plotted as short-dashed lines.
Resting and end-exercise musVO2
The mean resting musVO2 for 13 participants was 0.12 ± 0.09 HbDiffµM/s. The mean end-exercise musVO2 derived after the short-fast and long-slow exercise protocols were 1.39 ± 1.00 and 1.44 ± 1.23 HbDiffµM/s, respectively. There was a strong positive correlation between end-exercise musVO2 measured during the short-fast versus long-slow exercise protocols (r = 0.82, p-value <0.001; Lin’s CCC = 0.78, p-value <0.001; Figure 2C). A Bland-Altman plot depicts the mean bias and limits of agreement [Mean bias (LoA): 0.25 (−1.15, 1.65) HbDiffµM/s; Figure 2D].
Reproducibility study
The mean values for the first and second time constant derived from the short-fast exercise protocol were 34.0 ± 16.4 s and 40.4 ± 18.5 s, respectively. There was a strong positive correlation (cc = 0.68, p < 0.001; Lin’s CCC = 0.63; p < 0.001; Figure 3A) and good agreement between the first and second measurements with a mean difference [LoA] of −6.4 [−34.0, 21.3] seconds (Figure 3B).
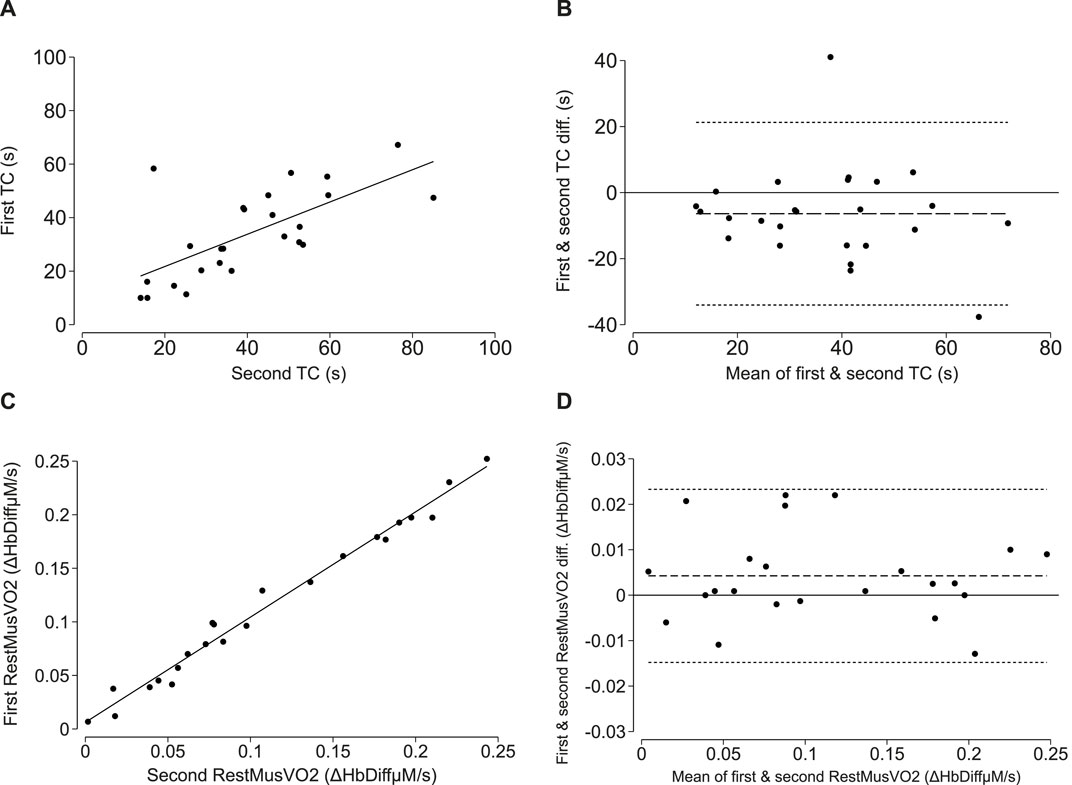
Figure 3. Reproducibility of NIRS-measured oxidative capacity and resting muscle oxygen consumption (RestmusVO2) in adults. (A, C) correlations between the first and second time constant (TC; τ) and first and second resting oxygen consumption measure. The line of best fit is plotted in solid black. (B, D) Bland-Altman plots demonstrating levels of agreement between the first and second time constant (TC; τ) and first and second resting oxygen consumption measure. The mean difference is plotted as the long-dashed line and the upper and lower limits of agreement are plotted as short, dashed lines.
Resting musVO2
The mean values for the first and second resting musVO2 measurements were 0.11 ± 0.07 HbDiffµM/s and 0.11 ± 0.07 HbDiffµM/s, respectively. There was a positive correlation (cc = 0.99, p < 0.001; Lin’s CCC = 0.99, p < 0.001; Figure 3C) and good agreement between the first and second musVO2 measurements with a mean difference [LoA] of 0.004 [−0.02, 0.02] HbDiffµM/s (Figure 3D).
Discussion
In this study we present evidence for good agreement between NIRS measures of oxidative capacity (τ) in the gastrocnemius performed using a short, rapid-contraction (short-fast) versus long, low frequency contraction (long-slow) exercise protocol. We also show good reproducibility of oxidative capacity measured using the short-fast protocol in non-athletic adults.
Agreement between τ derived after short-fast versus long-slow exercise protocols
This study demonstrates, for the first time, comparable measures of oxidative capacity can be derived following two different resistance-band exercise protocols. This finding has important implications for protocol development in both the field of NIRS and 31P-MRS, where the reference-standard for PCr recovery can be measured (Šedivý et al., 2015; Menon et al., 2021; Meyerspeer et al., 2020). During 31P-MRS, depletion of PCr can be tracked using a long-slow protocol which permits measurements to be made intermittently throughout exercise whilst minimising movement artifact. When conducting NIRS assessments, the recovery of PCr (estimated through repeat measures of oxygen consumption during arterial occlusions) is the measurement of interest, therefore a short-fast protocol, is sufficient to deplete PCr and the potential for movement artifact during exercise is less problematic. Previously, Ryan et al. concluded that NIRS-measured muscle oxidative capacity is comparable across different 15-s exercise performed on a mechanical pedal versus electrical stimulation of various randomized contraction frequencies (Ryan et al., 2013b). In line with Ryan et al., this study further demonstrates that NIRS can be used for oxidative capacity assessment using different duration and contraction frequency exercise protocols. A limitation of this study, however, is the use of alternate allocation randomization of exercise protocols. The use of a randomization software would have been a more rigorous approach.
In this study, the mean NIRS-measured oxidative capacity values for both short duration-fast contraction and long duration-slow contraction exercise protocols (35 and 38 s, respectively) are similar to values previously reported (31–35 s) (Nagasawa et al., 2003; Ryan et al., 2013a; Ryan et al., 2014b) and similar to values for PCr recovery previously reported in a similar group of young healthy, non-athletic individuals (McCully et al., 1993; McCully et al., 1994; Forbes et al., 2009; Ryan et al., 2013a; Ryan et al., 2014a).
For future NIRS studies, it is arguably more convenient and time-efficient to employ the 10-s exercise protocol. This is in line with Larsen et al. who propose that a short, moderate-to-vigorous exercise is sufficient to achieve approximately 50% PCr depletion from baseline without inducing acidosis (Larsen et al., 2012). Similar studies comparing oxidative capacity measured across muscle groups or from different positions within the same muscle would permit better characterisation of NIRS reliability.
Several studies have previously employed NIRS to estimate oxidative capacity in individuals with different physical activity levels (Ryan et al., 2013c; Erickson et al., 2013; Lagerwaard et al., 2020), in different age groups (Lagerwaard et al., 2020) and across different muscle groups/locations (Nagasawa et al., 2003; Adami et al., 2020; Lagerwaard et al., 2020). We observed a considerably wide range of time constant values across our YA group for both short-fast and long-slow protocols (10–86 s). We speculate that this may be due to participants undertaking different volumes of physical activity or exercise training, both of which are known to improve skeletal muscle oxidative capacity and therefore reduce τ (McCully et al., 1994). Conversely, detraining or inactivity is known to deteriorate oxidative capacity and lengthen τ (Šedivý et al., 2015). A limitation of our study is that we did not measure habitual physical activity in our participants and therefore, we cannot confirm this speculation. Furthermore, we included a heterogenous population with respect to age, sex and ethnicity into our study which may also contribute to our wide range of oxidative capacity values across individuals (Erickson et al., 2013).
We observed a small bias of ∼3 s shorter τ following the short-fast exercise protocol. One possible explanation for this is that the LS protocol elicited a slightly higher intensity or workload compared to the short-fast protocol, however, the confidence limits around this estimate are wide and therefore the direction of the difference is more likely a chance finding than a systematically shorter TC following the SF protocol. Previous work suggests high intensity exercise severely prolongs the recovery time constants (Arnold et al., 1984; McCully et al., 1993; McCully et al., 1994), likely due to the onset of anaerobic metabolism and associated drop in intracellular pH that impairs the highly active skeletal muscle creatine kinase activity and reduces PCr re-synthesis rate (Arnold et al., 1984; Bendahan et al., 1990). A limitation of NIRS is that it is not possible to measure pH during exercise. To explore this further, we compared the muscle oxygen consumption values measured immediately at the end of exercise (end-exercise musVO2). End-exercise musVO2 slopes were well-correlated between the two exercise protocols, indicating a similar rate of oxygen consumption at the end of exercise. End-exercise musVO2 was on average greater in the short-fast protocol, however, in line with our τ findings, this was also likely a chance finding.
Mono-exponential curves were excluded from our summary analyses based on poor fit (r2 < 0.70) and had associated prolonged time constants. It is possible that the prolonged time constants were due to myocellular acidosis leading to a failure of the participant to recover fully within the 3-min window where we monitored recovery, highlighting the importance of monitoring recovery beyond 3 min. However, we cannot rule out a methodological error that may have led to these outliers.
To date, most published studies that assessed muscle oxidative capacity by comparing exercises of different intensities were carried out with 31P-MRS. PCr recovery after exercise is independent of exercise intensity as long as pH remains relatively constant, but will be prolonged with decreased pH (McCully et al., 1993; McCully et al., 1994; Walter et al., 1997; Forbes et al., 2009). Forbes et al. demonstrated a similar PCr recovery time constant between low and high-intensity exercise, despite a significantly different end-exercise PCr level, but with similar end-exercise pH. In such a case, fast glycolytic ATP production following higher-intensity exercise offsets the higher post-exercise PCr reduction, thereby resulting in a comparable PCr time constant between lower and higher-intensity exercise (Forbes et al., 2009). Using 31P-MRS of different protocols, Walter et al. (1997), Ryan et al. (2013a), and McCully et al. (1993), McCully et al. (1994) documented end-exercise pH of ≥7.00 results in comparable PCr time constants.
Therefore, our study provides novel evidence that, similar to 31P-MRS, NIRS can be a reliable alternative for in vivo oxidative capacity assessment with different intensity exercise protocols. Compared to the long exercise protocol, the short exercise is arguably more feasible, time efficient and acceptable for participants.
Reproducibility in non-athletic adults
We present evidence for good agreement between repeated measures of oxidative capacity in the gastrocnemius using the short-fast protocol in a group of non-athletic adults of wide age range (18–86 years old). Our findings are aligned with good reproducibility of these measures in young adults and in lean athletic older adults undertaking NIRS measures from the vastus lateralis muscle (Fennell et al., 2023). NIRS-measured oxidative capacity was also found to be reproducible in OA smokers with and without chronic obstructive pulmonary disease (COPD) (Adami et al., 2018). Interestingly, compared to Fennel et al (Fennell et al., (2023), we obtained a shorter mean time constant despite including obese and older participants. This suggests the variability of oxidative capacity of different muscle, relative to muscle activeness, as the gastrocnemius is the primary locomotor muscle in walking, standing, or in sway (Adami et al., 2018). Another notable strength of this study is that we have included an ethnically diverse population. This is an important contribution to the literature, as previous NIRS studies have predominantly been performed in Caucasian individuals only. In addition, participants in this study were non-athletic and therefore likely reflect the general population. A limitation of this study, however, is that an objective and formal assessment of habitual physical activity levels was not performed. A further strength of our work is that the gastrocnemius muscle was selected as the NIRS measurement site, limiting the NIR light scattering that may occur at other measurement sites where ATT is likely to be much higher. We also utilised an exercise protocol set-up which consisted of a Velcro waistband and plastic resistance band that is MRI compatible, allowing for future applications. Despite selecting the difference signal to account for blood volume shifts and greater signal to noise ratio in our analyses, as has been previously described (Ryan et al., 2012), a general limitation of this work is that we cannot exclude the possibility that this signal might be influenced by changes in local blood volume.
Conclusion
In conclusion, there is good agreement between different exercise protocols for estimates of skeletal muscle oxidative capacity using NIRS. The short-fast exercise protocol was reproducible in non-athletic adults. Together these findings support NIRS as a valuable non-invasive tool for measuring muscle oxidative capacity in young and older non-athletic adults alike.
Data availability statement
The datasets presented in this article are not readily available because of the sensitive nature of the data collected for this study, but requests to access the dataset from qualified researchers trained in human subject confidentiality protocols may be sent to SJ at the MRC Unit for Lifelong Health and Ageing at UCL. Requests to access the datasets should be directed to c2lhbmEuam9uZXNAdWNsLmFjLnVr.
Ethics statement
The studies involving humans were approved by UCL Research Ethics Committee (21787.001) and National Research Ethics Service (NRES) Committee London—North Fulham. The studies were conducted in accordance with the local legislation and institutional requirements. The participants provided their written informed consent to participate in this study.
Author contributions
FT: Data curation, Formal Analysis, Investigation, Methodology, Writing–original draft, Writing–review and editing. AJ: Conceptualization, Data curation, Formal Analysis, Investigation, Methodology, Project administration, Supervision, Writing–original draft, Writing–review and editing. EH: Data curation, Investigation, Writing–original draft, Writing–review and editing. AH: Conceptualization, Supervision, Writing–original draft, Writing–review and editing. SJ: Conceptualization, Data curation, Investigation, Methodology, Supervision, Writing–original draft, Writing–review and editing.
Funding
The author(s) declare that financial support was received for the research, authorship, and/or publication of this article. The SABRE study was supported by the Wellcome Trust (grant numbers 067100, 60337055891 and 086676/7/08/Z), the British Heart Foundation (grant numbers PG/06/145, PG/08/103/26133, PG/12/29/29497 and CS/13/1/30327) and Diabetes UK (grant number 13/0004774). FT is supported by the Indonesian Endowment Fund for Education (LPDP, Indonesian Ministry of Finance). AJ is supported by the British Heart Foundation (grant number FS/19/63/34902). SJ is supported by a grant from the British Heart Foundation (grant number SP/F/21/150020). AH receives support from the BHF, the Horizon 2020 Framework Programme of the European Union, the National Institute for Health Research University College London Hospitals Biomedical Research Centre, the UK Medical Research Council, the Wellcome Trust and works in a unit that receives support from the UK Medical Research Council (grant number MC_UU_12019/1).
Acknowledgments
We are extremely grateful to all the people who took part in this study and to the past and present members of the research team at Bloomsbury Centre for Clinical Phenotyping who supported the authors in setting up the study.
Conflict of interest
The authors declare that the research was conducted in the absence of any commercial or financial relationships that could be construed as a potential conflict of interest.
Publisher’s note
All claims expressed in this article are solely those of the authors and do not necessarily represent those of their affiliated organizations, or those of the publisher, the editors and the reviewers. Any product that may be evaluated in this article, or claim that may be made by its manufacturer, is not guaranteed or endorsed by the publisher.
Supplementary material
The Supplementary Material for this article can be found online at: https://www.frontiersin.org/articles/10.3389/fphys.2024.1429673/full#supplementary-material
References
Adami A., Cao R., Porszasz J., Casaburi R., Rossiter H. B. (2018). Reproducibility of NIRS assessment of muscle oxidative capacity in smokers with and without COPD. Respir. Physiol. Neurobiol. 235, 18–26. doi:10.1016/j.resp.2016.09.008
Adami A., Corvino R. B., Calmelat R. A., Porszasz J., Casaburi R., Rossiter H. B. (2020). Muscle oxidative capacity is reduced in both upper and lower limbs in COPD. Med. Sci. Sports Exerc. 52, 2061–2068. doi:10.1249/MSS.0000000000002364
Arnold D. L., Matthews P. M., Radda G. K. (1984). Metabolic recovery after exercise and the assessment of mitochondrial function in vivo in human skeletal muscle by means of 31P NMR. Magnetic Reson. Med. 1, 307–315. doi:10.1002/mrm.1910010303
Bendahan D., Confort-Gouny S., Kozak-Reiss G., Cozzone P. J. (1990). Heterogeneity of metabolic response to muscular exercise in humans. New criteria of invariance defined by in vivo phosphorus-31 NMR spectroscopy. FEBS Lett. 272, 155–158. doi:10.1016/0014-5793(90)80472-u
Coen P. M., Musci R. V., Hinkley J. M., Miller B. F. (2019). Mitochondria as a target for mitigating sarcopenia. Front. Physiology 9, 1883. doi:10.3389/fphys.2018.01883
Erickson M. L., Ryan T. E., Young H. J., McCully K. K. (2013). Near-infrared assessments of skeletal muscle oxidative capacity in persons with spinal cord injury. Eur. J. Appl. Physiology 113, 2275–2283. doi:10.1007/s00421-013-2657-0
Fennell C. R. J., Mauger A. R., Hopker J. G. (2023). “Reproducibility of NIRS-derived mitochondrial oxidative capacity in highly active older adults,” in Exp. Gerontol. 175, 112156. doi:10.1016/j.exger.2023.112156
Forbes S. C., Paganini A. T., Slade J. M., Towse T. F., Meyer R. A. (2009). Phosphocreatine recovery kinetics following low- and high-intensity exercise in human triceps surae and rat posterior hindlimb muscles. Am. J. Physiology - Regul. Integr. Comp. Physiology 296, 161–170. doi:10.1152/ajpregu.90704.2008
Gomes M. J., Martinez P. F., Pagan L. U., Damatto R. L., Cezar M. D. M., Lima A. R. R., et al. (2017). Skeletal muscle aging: influence of oxidative stress and physical exercise. Oncotarget 8, 20428–20440. doi:10.18632/oncotarget.14670
Gonzalez-Freire M., Scalzo P., D’Agostino J., Moore Z. A., Diaz-Ruiz A., Fabbri E., et al. (2018). Skeletal muscle ex vivo mitochondrial respiration parallels decline in vivo oxidative capacity, cardiorespiratory fitness, and muscle strength: the Baltimore Longitudinal Study of Aging. Aging Cell 17, e12725. doi:10.1111/acel.12725
Jones S., Chiesa S., Chaturvedi N., Hughes A. (2016). Recent developments in near-infrared spectroscopy (NIRS) for the assessment of local skeletal muscle microvascular function and capacity to utilise oxygen. Artery Res. 16, 25–33. doi:10.1016/j.artres.2016.09.001
Jones S., Tillin T., Park C., Williams S., Rapala A., Al Saikhan L., et al. (2020). Cohort profile update: Southall and brent revisited (SABRE) study: a UK population-based comparison of cardiovascular disease and diabetes in people of European, south asian and african caribbean heritage. Int. J. Epidemiol. 49, 1441–1442E. doi:10.1093/ije/dyaa135
Kubota S., Endo Y., Kubota M., Ishizuka Y., Furudate T. (2015). Effects of trunk posture in Fowler’s position on hemodynamics. Aut. Neurosci. 189, 56–59. doi:10.1016/j.autneu.2015.01.002
Kubota S., Endo Y., Kubota M., Shigemasa T. (2017). Assessment of effects of differences in trunk posture during Fowler’s position on hemodynamics and cardiovascular regulation in older and younger subjects. Clin. Interventions Aging 12, 603–610. doi:10.2147/CIA.S132399
Lagerwaard B., Nieuwenhuizen A. G., de Boer V. C. J., Keijer J. (2020). In vivo assessment of mitochondrial capacity using NIRS in locomotor muscles of young and elderly males with similar physical activity levels. GeroScience 42, 299–310. doi:10.1007/s11357-019-00145-4
Lanza I. R., Nair K. S. (2010). Mitochondrial metabolic function assessed in vivo and in vitro. Curr. Opin. Clinincal Nutr. Metabolic Care 13, 511–517. doi:10.1097/MCO.0b013e32833cc93d
Larsen R. G., Callahan D. M., Foulis S. A., Kent-Braun J. A. (2012). Age-related changes in oxidative capacity differ between locomotory muscles and are associated with physical activity behavior. Appl. Physiology, Nutr. Metabolism 37, 88–99. doi:10.1139/h11-135
Marzetti E., Leeuwenburgh C. (2006). Skeletal muscle apoptosis, sarcopenia and frailty at old age. Exp. Gerontol. 41, 1234–1238. doi:10.1016/j.exger.2006.08.011
McCully K. K., Fielding R. A., Evans W. J., Leigh J. S., Posner J. D. (1993). Relationships between in vivo and in vitro measurements of metabolism in young and old human calf muscles. J. Appl. Physiology 75, 813–819. doi:10.1152/jappl.1993.75.2.813
McCully K. K., Iotti S., Kendrick K., Wang Z., Posner J. D., Leigh J., et al. (1994). Simultaneous in vivo measurements of HbO2 saturation and PCr kinetics after exercise in normal humans. J. Appl. Physiology 77, 5–10. doi:10.1152/jappl.1994.77.1.5
Menon R. G., Xia D., Katz S., Regatte R. (2021). “Dynamic 31P-MRI and 31P-MRS of lower leg muscles in heart failure patients,” Sci. Rep. 11, 1–10. doi:10.1038/s41598-021-86392-y
Meyerspeer M., Boesch M., Cameron M., Dezortová M., Forbes S., Heerschap A., et al. (2020). “31P magnetic resonance spectroscopy in skeletal muscle: experts ' consensus recommendations.” NMR Biomed 34, e4246. doi:10.1002/nbm.4246
Nagasawa T., Hamaoka T., Sako T., Murakami M., Kime R., Homma T., et al. (2003). A practical indicator of muscle oxidative capacity determined by recovery of muscle O2 consumption using NIR spectroscopy. Eur. J. Sport Sci. 3, 1–10. doi:10.1080/17461390300073207
Ryan T. E., Brizendine J. T., McCully K. K. (2013b). A comparison of exercise type and intensity on the noninvasive assessment of skeletal muscle mitochondrial function using near-infrared spectroscopy. J. Appl. Physiology 114, 230–237. doi:10.1152/japplphysiol.01043.2012
Ryan T. E., Brophy P., Lin C. T., Hickner R. C., Neufer P. D. (2014b). Assessment of in vivo skeletal muscle mitochondrial respiratory capacity in humans by near-infrared spectroscopy: a comparison with in situ measurements. J. Physiology 592, 3231–3241. doi:10.1113/jphysiol.2014.274456
Ryan T. E., Erickson M. L., Brizendine J. T., Young H. J., McCully K. K. (2012). Noninvasive evaluation of skeletal muscle mitochondrial capacity with near-infrared spectroscopy: correcting for blood volume changes. J. Appl. Physiology 113, 175–183. doi:10.1152/japplphysiol.00319.2012
Ryan T. E., Erickson M. L., Verma A., Chavez J., Rivner M. H., Mccully K. K. (2014a). Skeletal muscle oxidative capacity in amyotrophic lateral sclerosis. Muscle Nerve 50, 767–774. doi:10.1002/mus.24223
Ryan T. E., Southern W. M., Brizendine J. T., McCully K. K. (2013c). Activity-induced changes in skeletal muscle metabolism measured with optical spectroscopy. Med. Sci. Sports Exerc. 45, 2346–2352. doi:10.1249/MSS.0b013e31829a726a
Ryan T. E., Southern W. M., Reynolds M. A., McCully K. K. (2013a). A cross-validation of near-infrared spectroscopy measurements of skeletal muscle oxidative capacity with phosphorus magnetic resonance spectroscopy. J. Appl. Physiology 115, 1757–1766. doi:10.1152/japplphysiol.00835.2013
Santanasto A., Coen P. M., Glynn N. W., Conley K. E., Jubrias S. A., Amati F., et al. (2016). The relationship between mitochondrial function and walking performance in older adults with a wide range of physical function. Exp. Gerontol. 81, 1–7. doi:10.1016/j.exger.2016.04.002
Scheeren T. W. L., Schober P., Schwarte L. A. (2012). Monitoring tissue oxygenation by near infrared spectroscopy (NIRS): background and current applications. J. Clin. Monit. Comput. 26, 279–287. doi:10.1007/s10877-012-9348-y
Šedivý P., Kipfelsberger M., Dezortová M., Krššák M., Drobný M., Chmelík M. (2015). “Dynamic 31P MR spectroscopy of plantar flexion: influence of ergometer design, magnetic field strength (3 and 7 T), and RF-coil design,” Med. Phys. 42, 1678–1689. doi:10.1118/1.4914448
Southern W. M., Ryan T. E., Reynolds M. A., McCully K. (2014). Reproducibility of near-infrared spectroscopy measurements of oxidative function and postexercise recovery kinetics in the medial gastrocnemius muscle. Appl. Physiology, Nutr. Metabolism 39 (5), 521–529. doi:10.1139/apnm-2013-0347
Sumner M. D., Beard S., Pryor E. K., Das I., McCully K. K. (2020). Near infrared spectroscopy measurements of mitochondrial capacity using partial recovery curves. Front. Physiology 11, 111–119. doi:10.3389/fphys.2020.00111
Trevino M. B., Zhang X., Standley R. A., Wang M., Han X., Reis F. C. G., et al. (2019). Loss of mitochondrial energetics is associated with poor recovery of muscle function but not mass following disuse atrophy. Am. J. Physiology - Endocrinol. Metabolism 317, E899–E910. doi:10.1152/ajpendo.00161.2019
Van Beekvelt M. C., Colier W. N., Wevers R. A., Van Engelen B. G. (2001). Performance of near-infrared spectroscopy in measuring local O2 consumption and blood flow in skeletal muscle. J. Appl. physiology 90 (2), 511–519. doi:10.1152/jappl.2001.90.2.511
Keywords: near-infrared spectroscopy, skeletal muscle, oxidative capacity, exercise near-infrared spectroscopy, exercise
Citation: Tandirerung FJ, Jamieson A, Hendrick E, Hughes AD and Jones S (2024) Near-infrared spectroscopy (NIRS) in vivo assessment of skeletal muscle oxidative capacity: a comparison of results from short versus long exercise protocols and reproducibility in non-athletic adults. Front. Physiol. 15:1429673. doi: 10.3389/fphys.2024.1429673
Received: 08 May 2024; Accepted: 26 June 2024;
Published: 23 July 2024.
Edited by:
Stephane Perrey, Université de Montpellier, FranceReviewed by:
Sémah Tagougui, Université de Lille, FranceTakafumi Hamaoka, Tokyo Medical University, Japan
Copyright © 2024 Tandirerung, Jamieson, Hendrick, Hughes and Jones. This is an open-access article distributed under the terms of the Creative Commons Attribution License (CC BY). The use, distribution or reproduction in other forums is permitted, provided the original author(s) and the copyright owner(s) are credited and that the original publication in this journal is cited, in accordance with accepted academic practice. No use, distribution or reproduction is permitted which does not comply with these terms.
*Correspondence: Alexandra Jamieson, YWxleGFuZHJhLmphbWllc29uLjE2QHVjbC5hYy51aw==
†These authors have contributed equally to this work and share first authorship