- State Key Laboratory of Oral Diseases, National Center for Stomatology, National Clinical Research Center for Oral Diseases, West China Hospital of Stomatology, Sichuan University, Chengdu, China
Mesenchymal stem cells endow various functions, including proliferation, multipotency, migration, etc. Craniofacial bones originate from the cranial neural crest and are developed mainly through intramembranous ossification, which are different from long bones. There are varied mesenchymal stem cells existing in the craniofacial bone, including Gli1 + cells, Axin2 + cells, Prx1 + cells, etc. Nerves distributed in craniofacial area are also derived from the neural crest, and the trigeminal nerve is the major sensory nerve in craniofacial area. The nerves and the skeleton are tightly linked spatially, and the skeleton is broadly innervated by sensory and sympathetic nerves, which also participate in bone development, homeostasis and healing process. In this review, we summarize mesenchymal stem cells located in craniofacial bone or, to be more specific, in jaws, temporomandibular joint and cranial sutures. Then we discuss the research advance concerning neural regulation of mesenchymal stem cells in craniofacial bone, mainly focused on development, homeostasis and repair. Discovery of neural regulation of mesenchymal stem cells may assist in treatment in the craniofacial bone diseases or injuries.
1 Introduction
The concept of mesenchymal stem cell (MSC) suggests the existence of stem and/or progenitor cell populations in adult and neonatal tissues, which possess the capacity of providing de novo-specialized cells of mesodermal lineage (Naji et al., 2019). MSCs are able to differentiate into adipocytes, chondroblasts and osteoblasts under a definite stimulation in vitro (Naji et al., 2019). Typically, MSCs are derived from adult bone marrow and adipose tissue, and neonatal tissue such as umbilical cord is also utilized to obtain MSCs (Hass et al., 2011). Among the different sources of MSCs, bone marrow remains the most valued since it is better documented and widely used in preclinical and clinical studies (Yin et al., 2019). The function of MSCs include proliferation, multipotency, trophic ability, homing/migration and immunosuppression in fundamental aspects and their clinical implications (Naji et al., 2019).
The skeleton, comprised of specialized connective tissues, includes ossified/non-ossified elements, bone marrow stroma, and supportive tissues (Karsenty, 2003). These tissues are generated by diverse cell types, including osteocytes, chondrocytes, hematologic and stromal cells (Chan et al., 2015; Chan et al., 2018). Craniofacial bones are different from long bones concerning their developmental origins and osteogenic programs and structures (Chai et al., 2000). Long bones originate from trunk mesoderm, whereas most craniofacial bones are derived from the cranial neural crest (Chai et al., 2000). Craniofacial bones are flat and they are developed mainly through intramembranous ossification rather than endochondral ossification (Chai et al., 2000). In endochondral ossification, a cartilaginous template is formed, and then cartilage is gradually replaced with bone (Berendsen and Olsen, 2015). In intramembranous ossification, no cartilage is observed, and bony tissue is directly formed (Berendsen and Olsen, 2015). It should be noted that temporomandibular joint (TMJ) condyle undergoes endochondral ossification in the developmental stage (Stocum and Roberts, 2018).
Nerves distributed in craniofacial area are also derived from neural crest cells. During the migration, neural crest cells experience an epithelial-mesenchymal transition and differentiate into a wide range of derivatives, including neurons of the dorsal root ganglia of spinal nerves, some neurons of cranial ganglia, the autonomic nervous system (both sympathetic and parasympathetic ganglia) and Schwann cells which are responsible for the myelination of peripheral nerves (La Noce et al., 2014; Soldatov et al., 2019). The trigeminal nerve is the major sensory nerve in craniofacial area. The trigeminal ganglion (sensory branch) divides into three subdivisions, including ophthalmic (V1), maxillary (V2), and mandibular (V3) nerves (Freddi et al., 2022). Inferior alveolar nerve (IAN) is one of the branches of V3, which enters the mandibular foramen at the lingual surface of the mandibular ramus, travels within the inferior alveolar canal and exits through the mental foramen giving off the mental nerve (Freddi et al., 2022).
The nerves and the skeleton are tightly linked spatially, and the skeleton is broadly innervated by sensory and sympathetic nerves (Xu et al., 2022b). The nervous system’s role in regulating bone development, metabolism, homeostasis and remodeling has been confirmed (Assefa, 2023). However, our knowledge of the interaction between nervous system and skeletal system is still inadequate (Xu et al., 2022b). How the nerves regulate bone homeostasis suggests a brand-new avenue of research in bone biology (Wang et al., 2021). To date, researchers have been exploring neural regulation of stem cells in the skeletal microenvironment, gradually uncovering the roles of nerves in bone development, homeostasis and remodeling (Grässel, 2014; Chen et al., 2019; Wang et al., 2020a; Tomlinson et al., 2020). Nerve fibers are discovered to be involved in the formation of the bone marrow stem cell niche (Méndez-Ferrer et al., 2010).
In this review article, we list and delineate MSCs existing in jaws, TMJ and cranial sutures. Then we discuss the role of nervous system in craniofacial bone via the regulation of MSCs, mainly focused on development, homeostasis and repair, and hopefully shedding new light on modulation and treatment in the bone diseases and injuries.
2 MSCs in craniofacial bone
MSCs in craniofacial bones are diversified and display a series of markers. They primarily reside in jaws, TMJ and cranial sutures (Figure 1).
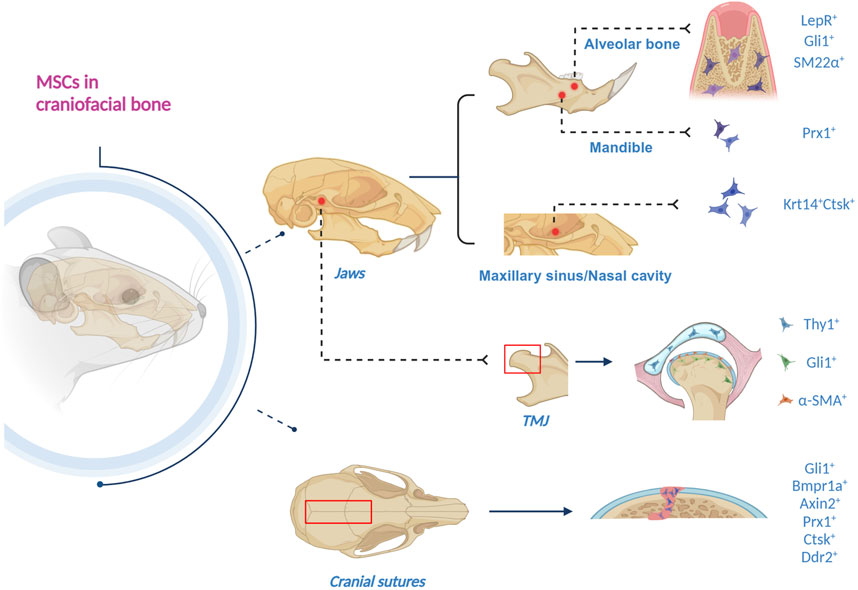
Figure 1. Schematics of craniofacial MSCs, residing in jaws, TMJ and cranial sutures. 1) In the alveolar bone, LepR+, Gli1+, and SM22α + MSCs have been discovered. 2) Gli1 + MSCs also reside in apical mesenchyme. 3) Prx1 + MSCs exist in the mandible. 4) Krt14 + Ctsk + MSCs are in the maxillary sinus and nasal cavity. 5) Gli1 + MSCs are in the TMJ subchondral bone, the periosteum and the perichondrium of TMJ condyle. 6) α-SMA + MSCs reside in the fibrous superficial zone tissue in the TMJ condyle. 7) Thy1 + MSCs are in the TMJ disc. 8) Gli1+, Bmpr1a+, Axin2+, Prx1+, Ctsk + and Ddr2 + MSCs have been found in the cranial sutures (Created with BioRender.com).
2.1 MSCs in jaws
Unlike long bones, our understanding of stem cells in jaws is insufficient (Li et al., 2022a). To date, seven markers labeling MSC residing in the jaws have been discovered, i.e., LepR, Gli1, SM22α, Fat4, Prx1, and Krt14/Ctsk.
Research on long bones has revealed that leptin receptor (LepR)-expressing bone marrow stromal cells are the major source of osteoprogenitor cells, which arose postnatally and differentiated into most bone and adipocytes (Zhou et al., 2014). Zhang et al. (2020a) identified tissue-resident LepR + MSCs in alveolar bone of the adult mice, which maintained in a quiescent state but rapidly got activated upon injury and were differentiated into osteoblasts. Further studies indicated that LepR + MSCs are indispensable for a normal jaw bone-healing process, in which PTH/PTH1R signal axis plays a critical role (Zhang et al., 2020a).
Gli1 + cells have been identified as stem cells in many tissues (Zhao and Chai, 2015; Schneider et al., 2017; Sena et al., 2017; Shi et al., 2017). Gli1 is a direct target gene of hedgehog signaling, and Indian hedgehog and Gli1 play a pivotal role in the regulation of craniofacial development (Sun et al., 2024). It has been discovered that Gli1 + cells surrounding the neurovascular bundle are MSCs that support the homeostasis and repair of incisor mesenchyme (Zhao et al., 2014). In addition, Gli1 + cells were identified as MSCs in the developing molar apical mesenchyme in a mouse model (Feng et al., 2017). Yi et al. (2022) found Gli1 + cells residing in alveolar bone marrow (ABM), and with the assistance of lineage tracing, they discovered that Gli1 + cells in ABM are activated and proliferate along blood vessels following tooth extraction, contributing to socket healing and implant osseointegration.
SM22α is primarily expressed in mural cells, visceral smooth muscles and myofibroblasts, and the roles of SM22α + cells in niches of tissue development and tumor malignancy have been clarified (Zhang et al., 2020b; Muhl et al., 2020; Li et al., 2021). In alveolar bone, SM22α-lineage niche cells are activated upon injury (Zhou et al., 2022). Interestingly, SM22α-lineage niche cells did not exhibit MSC-like expression pattern, and instead regulated various processes of alveolar bone regeneration via PDGFRβ-driven H₂S generation (Zhou et al., 2022).
Utilizing single-cell RNA sequencing (scRNA-seq), Jin et al. (2023) uncovered a previously unidentified cell population exhibiting a high expression of protocadherin Fat4, which is specifically enriched around ABM cavities. It was demonstrated that Fat4 + cells possess colony-forming, osteogenic, and adipogenic capabilities, with the core transcriptional features regulating osteogenic differentiation, verifying that these cells belong to MSCs residing in the alveolar bone (Jin et al., 2023).
Prx1 is a homeobox gene expressed in the skeleton system, and its mutation has been discovered to be related to underdeveloped mandible (Esposito et al., 2020). A recent study has elucidated the crucial role of Prx1-expressing cells as stem cells for bone, which is mainly reflected in skeletal development, growth, homeostasis and aging (Liu et al., 2022). Jiang et al. established canine distraction osteogenesis (DO) models of the mandible, revealing the reversion of Prx1+MSCs to primitive neural-crest-cell-like state (Jiang et al., 2023). In addition, this study provided evidence demonstrating that Prx1 deficiency leads to impaired osteogenic ability in DO (Jiang et al., 2023).
A subset of cells co-expressing epithelial (Krt14) and mesenchymal (Ctsk) markers in maxillary sinus and the nasal cavity have been identified as osteoprogenitors, contributing to homeostasis and injury-induced osteogenesis of the maxillary sinus floor (Weng et al., 2022). Krt14 + Ctsk + cells exhibit both epithelial and mesenchymal properties, and specifically play a role in bone regeneration after maxillary sinus floor lifting (Weng et al., 2022). Lineage tracing with dual recombinases showed that descendants of Krt14 + Ctsk + progenitors, which are Krt14-Ctsk+, underwent robust osteogenesis, revealing Krt14 + Ctsk + cells serve as a novel lineage of osteoprogenitor cells in craniofacial bone regeneration (Weng et al., 2022).
2.2 MSCs in TMJ
The temporomandibular joint (TMJ) is a unique articulation of the craniofacial bone between the mandible and the temporal bone. The main components of TMJ are articular condyle, articular disc, articular fossa, fibrous capsule, and synovial membrane (Palla, 2016). TMJ develops from three separate mesenchymal condensations representing the glenoid fossa of the temporal bone, the condyle of the mandibular ramus, and the articular disc (Stocum and Roberts, 2018). The active growth element of the condyle is a secondary cartilage derived from the medial periosteal cells expressing tenascin-C of the mandibular ramus, which will undergo endochondral ossification. So far, three markers have been discovered that label the stem cells in the TMJ region, i.e., Gli1, α-SMA and Thy1.
Utilizing lineage tracing analysis, Gli1 + cells were found residing in the subchondral bone, serving as osteogenic progenitors for postnatal osteogenesis and homeostasis in the mouse mandibular condyle (Lei et al., 2022). Activation of Gli1 + cells contributes to increased and uneven distribution of osteoblastic lineage differentiation, interfering the homeostasis of subchondral bone region and accelerating pathological changes during the development of TMJ osteoarthritis (Lei et al., 2022). In another study, it was revealed that Gli1 + cells reside within both the periosteum and the perichondrium of condyle (Zhang et al., 2023a). Further, the condylar Gli1 + cells possess both osteogenic and chondrogenic potential while the periosteal-associated cells only have osteogenic potential (Zhang et al., 2023a).
In these years, a few studies have indicated that MSC population resides in the articular cartilage, playing a critical role in cell renewal and differentiation during cartilage modeling and repair (Tong et al., 2015; Levato et al., 2017; Wang et al., 2020b). Embree et al. discovered that the fibrous superficial zone tissue in the TMJ condyle is a niche harboring fibrocartilage stem cells (FCSCs), which were first isolated from the rat TMJ (Embree et al., 2016). Utilizing lineage tracing analysis, mouse FCSCs in the TMJ condyle were identified with the stem cell label α-SMA (Embree et al., 2016). FCSCs were found to possess the capacity of cartilage regeneration and repair upon injury (Embree et al., 2016).
It should be noted that Bi et al. (2020) verified a stem cell population residing in the superficial layer of human TMJ condylar cartilage, named human fibrocartilage stem cells (hFCSCs), which exhibit distinct stemness. In addition, research results from Yin et al. (2023) highlight that hFCSCs from condyle osteochondroma displayed a specific chondrogenic differentiation capacity and were liable to forming more mature and hypertrophic cartilage during the chondrogenic inducing process. Besides, abnormally activated PI3K/AKT signaling in condyle osteochondroma has the potential for leading to functional changes of hFCSCs by regulating cell apoptosis, bringing about TMJ cartilage lesion in condyle osteochondroma patients or animals (Yin et al., 2023). Nevertheless, no specific marker has been identified so far to label hFCSCs.
In order to deepen the understanding of cellular composition of TMJ discs, a comprehensive scRNA-seq analysis of mouse TMJ disc tissues was performed and four principal cell types were identified, which exhibited various transcript expression characteristics and cellular functions, including fibroblast, macrophage, endothelial cell and mural cell (Bi et al., 2023). It was confirmed that Thy1 + mural cells in TMJ articular discs manifest significantly stronger stem cell characteristics than other Thy1-disc cells in vitro and ex vivo, such as higher colony formation potential as well as proliferative capacities (Bi et al., 2023). Moreover, in virtue of tri-lineage transduced differentiation in vitro, Thy1 + cells were also found to possess markedly stronger potential for osteogenesis, adipogenesis and chondrogenesis (Bi et al., 2023). Mural cells, including Thy1 + cells, were demonstrated to transform toward non-chondrogenic fibroblasts in TMJ disc injury (Bi et al., 2023).
2.3 MSCs in cranial sutures
Cranial sutures have been identified as the major location of MSCs, revealing that the regeneration capacity of the craniofacial bones should reside within the sutures (Park et al., 2016). It has been found that sutures possess much stronger regeneration capacity than other regions of the calvaria (Park et al., 2016). So far, there are six markers verified to be labelled suture mesenchymal stem cells (SuSCs), including Gli1, Axin2, Bmpr1a, Prx1, Ctsk and Ddr2.
Gli1+ cells within the suture mesenchyme were first verified as the main SuSCs for adult craniofacial bones by Zhao et al. (2015). Assisted by lineage tracing analysis, this team delineated that Gli1 + SuSCs in the suture give rise to osteogenic fronts, periosteum and dura (Zhao et al., 2015). Subsequently, Park et al. (2016) further confirmed that Gli1 + SuSCs within the suture mesenchyme are the cellular source for injury repair and bone regeneration, emphasizing that bone healing capacity of calvaria is primarily restricted in sutures rather than evenly distributed on the calvarial surface. Another research established rapid maxillary expansion mouse models and mechanical stretch loading cell models, and it was uncovered that Gli1 + cells in maxillofacial sutures participate in mechanical force-induced bone formation (Huang et al., 2021). Additionally, Jing et al. (2022) utilized a standardized model for mouse suture expansion and first demonstrated that Gli1 + SuSCs are the stem cell source to facilitate craniofacial DO, in which canonical Wnt signaling expressed in Gli1 + SuSCs is essential.
Maruyama et al. (2016) first identified Axin2 + cells as SuSCs, providing solid evidence to prove their stemness, including longterm self-renewing, clonal expanding and differentiating abilities, and demonstrated their injury repair and regenerative capacity. It is important to note that unlike Gli1 + SuSCs, Axin2 + SuSCs are mainly located and concentrated in the midline of the suture mesenchyme at early stages after birth (Maruyama et al., 2016). Lineage tracing analysis suggested that Axin2 + SuSCs and their derivatives are widely distributed in all patent sutures and calvarial bones over a long tracing period (Maruyama et al., 2016). Gene-profiling analysis illustrated high levels of Gli1 expression in the Axin2-expressing cells, which implied an overlap of these two cell populations (Maruyama et al., 2016). It can be assumed that Axin2 + SuSCs likewise possess the potential for bone regeneration and injury repair.
Maruyama et al. (2021) conducted further research and discovered BMP ligands signal via the type Ⅰ receptor Bmpr1a to activate the pathway in Axin2 + SuSCs. They demonstrated that Bmpr1a plays a crucial role in the maintenance of SuSC stemness, and regulates not only SuSC self-renewal but also SuSC-mediated osteogenesis (Maruyama et al., 2021). It should be noted that in both mice and humans, Bmpr1a/BMPR1A functions as an SuSC marker, and Bmpr1a regulates stem cell stemness, which is critical for suture patency and craniosynostosis (Maruyama et al., 2021).
Postnatal cells expressing Prx1 of the calvarial sutures have been verified exhibiting qualities of MSCs, and calvarial sutures proved to be the exclusive niche of Prx1 + cells (Wilk et al., 2017). Prx1 + SuSCs proved residing exclusively in the posterior frontal, coronal, sagittal, and lambdoid sutures, whereas they were not detectable in other craniofacial sutures, the calvarial periosteum or the dura mater (Wilk et al., 2017). This team confirmed that Prx1 + SuSCs and their progeny contribute to the development of calvarial sutures and calvarial bones, and play a role in calvarial bone defect regeneration (Wilk et al., 2017). Interestingly, this team presumed that Prx1 + SuSCs are a subpopulation of Axin2 + SuSCs (Wilk et al., 2017).
Debnath et al. (2018) identified a periosteal stem cell expressing Ctsk located in the long bones and calvaria of mice, and provided evidence of its clonal multipotency and self-renewal. Contrary to other MSCs which mediate endochondral ossification, Ctsk + stem cells choose intramembranous pathway for bone formation (Debnath et al., 2018). Ctsk + cells were discovered to acquire endochondral bone formation capacity in response to injury (Debnath et al., 2018). Subsequently, this team focused on craniosynostosis and calvarial mineralization, and they demonstrated the interaction of two separate stem cell lineages in the sutures: Ctsk + SuSCs and Ddr2 + SuSCs, which were first verified (Bok et al., 2023). Research data indicated that Ddr2 + SuSCs mediate a third fundamental form of bone formation, endochondral ossification with no haematopoiesis, alongside traditional intramembranous and endochondral ossification (Bok et al., 2023). It was revealed that interactions between these two cell types are essential for the pathogenesis of craniosynostosis and suture patency (Bok et al., 2023).
MSCs in craniofacial bone, despite different positions and diverse markers listed above, exhibit not only the capacity for long-term self-renewal, clonal expansion, differentiation and multipotency, but also the ability to respond to injuries and facilitate tissue regeneration.
3 Neural regulation of MSCs in craniofacial bone
The skeleton is mainly innervated by sensory and sympathetic nerves, and long bones and craniofacial bone are innervated by nerves originating at different locations (Xu et al., 2022b). Nerves play essential roles during the development of craniofacial tissues such as the salivary glands, teeth, and calvarial bones (Adameyko and Fried, 2016). The role of nervous system in bone homeostasis has been gradually realized in recent years (Chen et al., 2019; Meyers et al., 2020). Since nerves turned out to be involved in the stem cell microenvironment, the regulation of MSCs by the nervous system has become an increasing focus of research (Méndez-Ferrer et al., 2010; Zhao et al., 2014; Park et al., 2015; Maryanovich et al., 2018). Sensory and sympathetic nerves induce bone marrow MSCs (BMMSCs) migration to the osteogenesis front line through various neurotransmitters or neuropeptides, thus sustaining an active bone environment in osteogenesis (Wang et al., 2020a). In this section, we will discuss the role of neural regulation of MSCs in craniofacial bone development, homeostasis and repair.
3.1 Neural regulation of MSCs in craniofacial bone development
Research concerning neural regulation of MSCs in craniofacial bone development is still in its infancy, yet this topic is attracting increasing attention. Various cells, molecules or signaling pathways participate in this process, including Schwann cells, tropomyosin receptor kinase A (TrkA), FGF-SHH signaling, etc. Cranial bones, suture and teeth are the craniofacial elements demonstrated to be involved in the neural regulation.
Schwann cells and Schwann cell precursors (SCPs) were proved to be inactive neural-crest-like cells, which can be recruited from nerves, giving rise to dental MSCs and generating pulp cells and odontoblasts in adult teeth (Kaukua et al., 2014). It was further revealed that some SCPs detach from nerve fibers to become MSCs, contributing to chondrogenesis and osteogenesis (Xie et al., 2019). This process was demonstrated to appear exclusively during murine embryonic development, resulting in the formation of craniofacial elements without contribution to the development of the appendicular skeleton (Xie et al., 2019).
Gadomski et al. (2022) conducted research on mouse cranial and femoral bones and found that skeletal sympathetic cholinergic nerve fibers induced by interleukin-6 preserve osteocytes survival and function during postnatal development via a neurotrophic axis, which is mediated through GDNF (glial cell derived neurotrophic factor)-family receptor-α2 (GFRα2) and its ligand, neurturin (NRTN). GFRa2−/− mice showed reduced skeletal stem cells and bone formation, resulting in decreased bone mass and strength, enlarged cranial sutures and flatter skulls (Gadomski et al., 2022).
Tower et al. (2021) cast light on the role of TrkA + sensory nerves in suture patency of developing cranium. Research data clarified that TrkA signaling in peripheral afferent neurons is needed to sustain MSCs in sutures in a proliferative, undifferentiated state, and the loss of suture mesenchyme patency with a decline in cell proliferation contributes to the premature suture closure, leading to the failure of normal cranial vault expansion (Tower et al., 2021).
Fibroblast growth factor (FGF) plays a role in sensory nerves’ regulation of MSCs in craniofacial elements development, and it serves as a bridge connecting sensory nerves to Gli1 + MSCs. FGFs have been found to control a variety of physiological responses during embryonic development and in adult organisms, participating in patterning and morphogenesis via regulating cell survival, proliferation, migration and differentiation (Korsensky and Ron, 2016). Pei et al. (2024) discovered that FGF signaling modulates Gli1 + cells through its receptor FGFR2 during tooth root development in a mouse molar model, and FGF-SHH signaling axis participates in the regulation of tooth root morphogenesis. Loss of FGF signaling in Gli1 + cells results in shortened tooth roots with compromised root progenitor cell proliferation and differentiation (Pei et al., 2024).
The significance of neural regulation of MSCs in craniofacial bone development has been gradually uncovered. Nevertheless, except for the elements mentioned above, whether or how the development of other areas in the craniofacial bones is regulated by the nervous system requires further exploration.
3.2 Neural regulation of MSCs in craniofacial bone homeostasis and repair
3.2.1 Neural regulation in jaws
Jaws are the research hotspot representing neural regulation of MSCs in craniofacial bone homeostasis and repair, and sensory nerves are the major participants. Inferior alveolar nerve (IAN) is one of the principal sensory nerves in the mandible, and numerous studies revealed that IAN denervation results in disorders of bone homeostasis and repair. Wu et al. (2016b) showed that IAN transection results in mandibular bone loss in an age-dependent way. Hayano et al. (2018) demonstrated that mesenchymal stem/progenitor cell is related to IAN innervation, and its denervation leads to the impairment of incisor growth and formation, or specifically, a reduction in incisor eruption and enamel defects. Jones et al. (2019) showed that after IAN denervation, mandibular bone repair is impaired due to the functional defects in MSCs, which can be partially rescued by Schwann cell transplantation and by Schwann-derived growth factors platelet-derived growth factor-AA, oncostatin M and parathyroid hormone. Tevlin et al. (2023) discovered that mandibular DO with IAN denervation results in impaired osteogenesis and reduced MSC amplification and osteogenic potential in mice, which are arguments for the connection between nerves and mandible repair. Additionally, a human patient specimen was analyzed in this research and indicated that the histological, radiological, and transcriptional changes seen in the mouse DO model may be consistent in the context of denervated human mandibular DO (Tevlin et al., 2023). These studies in vivo demonstrated the point that the sensory nerve denervation influences stem cells, disturbing craniofacial bone homeostasis and repair.
Several nerve-related molecules have been confirmed to participate in sensory nerves’ regulation of MSCs in jaws homeostasis and repair, such as Calcitonin gene-related peptide (CGRP), Substance P (SP) and Semaphorin 3A (Sema 3A). CGRP is a polypeptide with 37 amino acids (Russell et al., 2014), and it is mainly synthesized in sensory nerve fibers (Choi and Di Nardo, 2018), stored and released as vesicles (Arkless et al., 2019). CGRP is secreted from the trigeminal semilunar neuron and transported to the bone in the maxillofacial region, performing its biological effects via binding to the main receptor transient receptor potential vanilloid 1 (TRPV1) (Grässel, 2014). It was revealed that innervation improves the osteogenic capacity of BMMSCs via CGRP to activate p38 MAPK and Wnt6/β-catenin pathways, thereby maintaining bone homeostasis (Wu et al., 2023b). Two independent groups have demonstrated that inhibition of CGRP impairs long bone fracture healing and regeneration (Appelt et al., 2020; Wee et al., 2023). Except for long bones, CGRP also makes contribution to jaws homeostasis and repair. Pang et al. studied the expression patterns of CGRP and SHH signaling in the tooth socket and deduced that in the socket healing process following tooth extraction, CGRP released by sensory nerves in the periodontal tissue stimulates MSCs’ proliferation and osteogenic differentiation, enhances osteoblast activation and attenuates osteoclasts’ function, hence facilitating osteogenesis and restricting bone resorption, which were found to be mediated by SHH signaling (Pang et al., 2015). Liu et al. (2018b) showed that IAN neurectomy erodes osteogenesis surrounding titanium implants with less CGRP fluorescence in the immunofluorescence assay, yet the consequence can be partially balanced via the injection of CGRP around the implant in vivo, in a process possibly mediated by canonical Wnt signaling pathway. Additionally, a research group have conducted a series of studies on the role of biodegradable magnesium implant in bone tissue regeneration and its connection with CGRP (Zhang et al., 2016; Tian et al., 2018; Ye et al., 2021). Recently, the significance of CGRP in the function of periodontal ligament cells has been firstly reported. It was indicated that CGRP stimulation induces the differentiation of a mouse periodontal ligament cell line (MPDL22 cells) into hard tissue-forming cells, underscoring the critical role of CGRP signaling in alveolar bone repair (Miki et al., 2024).
SP is a member of the tachykinin family comprised of 11 amino acids, which is widely distributed in CNS and PNS (Assefa, 2023). SP mainly binds to the neurokinin 1 receptor (NK1R) on non-neuronal cells, including MSCs, osteoblasts and osteoclasts (Wang et al., 2009). Systemic injection of SP promotes the migration of CD29+ MSCs to the wound region, facilitating bone remodeling through the activation of Erk1/2 signaling pathway (Hong et al., 2009; Zhang et al., 2014). Previous research has indicated that local administration of SP in a rat mandibular DO model can accelerate bone remodeling and enhance MSC migration during osteogenesis (Zhang et al., 2014). Another research team revealed that gelatin microspheres containing CGRP or SP effectively accelerate bone formation, yet its therapeutic effects on human alveolar ridge defects still need further investigation (Chen et al., 2017).
Sema 3A is a neuroinformatic protein molecule widely expressed in various tissues and organs, exerting its influence via binding to two co-transmembrane receptors, neuropilin-1 (Nrp-1) and PlexinA family, which has drawn attention to its protective function in bone homeostasis (Wu et al., 2023a). It has been discovered that Sema 3A and Nrp1 binding inhibited osteoclast differentiation via the inhibition of the ITAM and RhoA signaling pathways and stimulated osteogenic differentiation through Wnt/β-catenin signaling pathway (Hayashi et al., 2012). It was revealed that Sema 3A is a fundamental positive regulator in human alveolar BMMSCs (hABMMSCs) osteogenic differentiation (Liu et al., 2018a). Studies from several independent groups revealed that Sema 3A may contribute to bone remodeling during orthodontic tooth movement (OTM) (Şen et al., 2021; Kamei et al., 2022; Mei et al., 2024). Şen et al. (2021) showed that human osteoblasts from alveolar bone responded to Sema 3A stimulation with a Rac1 and β-catenin-dependent induction of differentiation markers. Kamei et al. (2022) found that osteoblast-derived Sema 3A regulates alveolar bone remodeling during OTM by accelerating osteoblast calcification on the tension side and inhibiting osteoclastogenesis on the compression side. Besides, Mei et al. (2024) emphasized the important role of neurons-derived Sema 3A in loads-mediated bone formation during OTM. Exogenous Sema3A accelerated alveolar bone formation and the osteogenic differentiation of human periodontal ligament cells (hPDLCs) induced by mechanical overload (Mei et al., 2024).
Except for the nerve-related molecules mentioned above, FGF also plays a role in sensory nerves’ regulation of MSCs in jaws or, to be more specific, in incisor mesenchyme tissue homeostasis, and it serves as a bridge connecting sensory nerves to Gli1 + MSCs. It was revealed that sensory nerves activate Gli1 + MSCs via secreting Shh protein, which contribute to all mesenchymal derivatives in a mouse incisor model (Zhao et al., 2014). Recently, a study highlighted that FGF signaling is essential for the interaction between sensory nerves and the proximal mesenchyme of the incisor (Pei et al., 2023). Secreted from sensory neurons, FGF1 directly acts on Gli1+MSCs via binding with its receptor FGFR1 to sustain the mesenchyme tissue homeostasis, which influences MSC maintenance, odontoblast differentiation and migration (Pei et al., 2023). In terms of the mechanism, it was demonstrated that activated FGF/p-JNK signaling regulates mTOR-dependent autophagy activation in Gli1 + MSCs (Pei et al., 2023). This research, together with the study reported in 2024 (Pei et al., 2024), reveals FGF serves as a bridge connecting sensory nerves to Gli1 + MSCs and confirms the interaction between sensory nerves and Gli1 + MSCs existing in the alveolar bone area.
Sensory nerves are not the only type of nerves present in the craniofacial region. Sympathetic nerves are also involved in craniofacial bone homeostasis and repair. It has been reported in these years that sympathetic signaling regulates bone remodeling in a negative fashion (Elefteriou et al., 2014; Corr et al., 2017; Elefteriou, 2018). Chen et al. (2019) discovered that sensory nerve activation suppresses sympathetic tone and facilitates osteoblast proliferation and differentiation. Norepinephrine (NE), as the main neurotransmitter of the sympathetic nervous system, is synthesized from the amino acid tyrosine by the action of tyrosine hydroxylase (TH) (Elefteriou, 2018). Sympathetic nerves release NE to modulate the metabolism and function of osteoblasts and osteoclasts via α-adrenergic and β-adrenergic receptors (ARs), and NE participates in the regulation mainly through β2AR (Xiao et al., 2023). A study showed that propranolol, a β-AR blocker, effectively enhances implant osseointegration in vivo, facilitates proliferation of osteoblasts and promotes osteogenic differentiation of osteoblasts and MSCs (Wu et al., 2021). It was indicated that sympathetic denervation-induced MSC mobilization in rat mandibular DO is correlated with inhibition of MSC migration and osteogenic differentiation via NE/adrb3 signaling pathway (Du et al., 2014). Furthermore, Wu et al. (2016a) discovered that in the rat mandibular DO model, NE may upregulate the SDF-1 secretion by vascular endothelial cells through NE/adrb3/JNK pathway, thus inhibiting the MSC chemotaxis migration towards bone trabecular frontlines during bone regeneration.
3.2.2 Neural regulation in suture/calvaria
The suture or calvaria is another position where nerves and MSCs are tightly connected and draws attention of researchers focusing on craniofacial bone homeostasis and repair. Nerve growth factor (NGF), vasoactive intestinal peptide (VIP) and molecules mentioned in the previous section (CGRP, SP and Sema 3A) have been discovered to be involved in the neural regulation of MSCs in suture/calvaria homeostasis and repair. NGF was previously considered as an indispensable neurotrophic factor related to sensory and sympathetic nerve growth (Hempstead et al., 1991). NGF binds to its high-affinity receptor, TrkA, which can be located on the surface of sensory nerves, and NGF is then transmitted by endosomes and experiences long-distance retrograde transport from the distal axon to the cell body of dorsal root ganglia (Skaper, 2017). A previous study demonstrated that the overexpression of TrkA, receptor of NGF, can promote Schwann-like cell differentiation of bone marrow stromal stem cells (Zheng et al., 2016). TrkA improves the survival and regenerative capacity of bone marrow stromal stem cells via the upregulation of the Erk/Bcl-2 pathway (Zheng et al., 2019). Tomlinson et al. (2016) showed that interruption of perichondrium-derived NGF expression or sensory nerve-associated TrkA activity prejudices long bone ossification. They then demonstrated an acute upregulation of NGF expression following long bone fracture in mice (Li et al., 2019). Research above is limited in long bone ossification and repair. This team then studied sensory nerves and NGF-TrkA signaling in mouse cranial bone healing. Abundant NGF is marked in mesenchymal cells of all patent cranial sutures, whereas NGF is scarce in fused posterofrontal suture (Meyers et al., 2020). Consistent with long bone (Li et al., 2019), NGF is sharply increased after calvarial bone injury. At early time points post-injury, NGF-expressing cell during this time were mainly PDGFRα + mesenchymal cells and F4/80 + macrophages, and bone-lining osteocalcin-positive osteoblasts were the major source of NGF during the later stages (Meyers et al., 2020). In addition, like the role of TrkA in long bone fracture, TrkA signaling also proved necessary for calvarial regeneration, and disruption of TrkA activity hindered re-innervation and delayed cranial bone defect healing (Meyers et al., 2020). Except for NGF-TrkA signaling pathway, this team also studied another NGF-related pathway. NGF-p75 signaling within mesenchymal lineage cells is crucial for cell migration and osteogenic differentiation during bone repair (Xu et al., 2022a). Additionally, Zhang et al. (2023b) proposed a neuromodulation approach to orchestrate critical-sized defects (CSDs) healing via the construction of engineered sensory nerves, and NGF released from sensory nerve-inductive scaffolds effectively induces sensory neuron differentiation and promotes CGRP secretion, leading to osteogenic differentiation of BMMSCs. The engineered sensory nerves accelerate osteogenesis via NGF-TrkA signaling and thus facilitate CSDs healing, providing an alternative solution for calvaria repair (Zhang et al., 2023b).
VIP is a neuropeptide which belongs to a glucagon/secretin superfamily, playing crucial roles in a broad spectrum of biological functions (Jiang et al., 2016). VIP binding with its receptor VPAC1 enhances BMMSCs osteogenic differentiation through Wnt/β-catenin signaling pathway in vitro, and VIP-conjugated functionalized hydrogel facilitates calvaria defect repair with increased osteogenesis and angiogenesis (Shi et al., 2020). In addition, CGRP, SP and Sema 3A also participate in calvarial repair. With recombinant lentiviral vector overexpressing CGRP, CGRP gene-modified BMMSCs were designed and found to be effective seed cells in tissue engineering to repair skull defects (Yu et al., 2019). Zhang et al. (2018) highlighted that intravenous SP attenuates inflammation both systemically and locally in calvarial injured mice. Intravenous SP can effectively facilitate the osteogenesis of MSCs and contribute to the calvarial CSD repair (Zhang et al., 2018). Besides, it was discovered that co-overexpression of Sema 3A and HIF1α promoted induced pluripotent stem cell-derived mesenchymal stem cells (iPSC-MSCs) osteogenesis and angiogenesis and bone regeneration (Li et al., 2020). They then applied Sema 3A-HIF1α co-overexpressed iPSC-MSCs seeded on HA scaffold to calvarial defect mouse model, and it turned out to be effective in bone repair (Li et al., 2020). Liu et al. (2016) showed that Sema 3A in BMMSCs is crucial for its potent osteogenic capacity, and transferring Sema 3A into adipose-derived stem cells (ASCs) partially reprograms the cells towards BMMSCs, thereby enhancing the osteogenic capacity. They further demonstrated that transplantation of Sema 3A infected ASCs significantly facilitate the bone regenerative capacities in the calvarial CSD model (Liu et al., 2016).
Similarly, except for sensory nerves and the nerve-related molecules, sympathetic nerves play a part in the regulation of MSCs in suture/calvaria homeostasis and repair. Li et al. (2022b) studied whether inhibition of sympathetic signaling assists in repair of CSDs. They designed a 3D printed hydrogel scaffold to imitate the microenvironment of bone regeneration, which is loaded with the calcium channel blocker nifedipine, thus decreasing the concentration of catecholamine and suppressing sympathetic activity in the bone defect region (Li et al., 2022b). They confirmed that this scaffold inhibited osteoclastogenesis, facilitated the migration and osteogenic differentiation of MSCs, and succeeded in rat cranial bone regeneration, implying the potential of sympathetic signaling inhibition for bone repair (Li et al., 2022b).
The main studies on neural regulation of MSCs in craniofacial bone homeostasis and repair are listed in Table 1. In brief, sensory nerves and sympathetic nerves with the nerve-related molecules collaborate in the regulation of MSCs in craniofacial bone homeostasis and repair. Sensory nerves are more inclined to promote osteogenesis while sympathetic nerves tend to regulate bone remodeling in a negative way.
4 Conclusion and perspective
In this review article, we summarized several MSCs located in craniofacial bones, including Gli1 + cells, Axin2 + cells, Ctsk + cells, Prx1 + cells, LepR + cells, etc. Then we discussed studies on the role of nervous system in craniofacial bones via the regulation of varied MSCs, primarily focused on bone development, homeostasis and repair. The regulation mainly depends on nerves with a series of molecules, such as NGF, CGRP, FGF, and NE, and several signaling pathways. As we can see, neural regulation of MSCs in the skeletal tissue is a hot topic of research currently. Reports in the last decade on nerve innervation and its participation in bone development and metabolism in craniofacial bones have been mentioned in the section “Neural regulation in craniofacial bone.” Nevertheless, several aspects in this field remain to be completed.
First, studies concerning neural regulation of MSCs with specific markers are limited. The recent advance in craniofacial bone MSCs is reported in the second part “MSCs in craniofacial bone,” and varied markers labeling MSC in jaws, TMJ and sutures have been identified. The third part “Neural regulation of MSCs in craniofacial bone” is based on the second part, introducing recent studies on the neural regulation of MSCs. However, the connection between nerves and MSCs with specific markers mentioned in the second part has not been verified completely. We highlighted Gli1 + cells which are widely studied in craniofacial biology in the third part of this review article, and emphasized that Gli1 + cells in mouse incisor and molar mesenchyme were demonstrated to be regulated by sensory nerves, in which FGF serves as the bridge (Pei et al., 2023; Pei et al., 2024). Besides, Yu et al. (2021) verified that suture Gli1 + MSCs with resorbable biomaterials support regeneration of cranial sutures in craniosynostosis mouse model, and dura mater cells contribute to Gli1 + cells in suture regeneration. Although no direct evidence of neural relevance in this process was found, sensory or sympathetic nerves may intervene in the regulation of Gli1 + MSCs, considering the dura mater is richly innervated by afferent nerve fibers from the trigeminal ganglion and by sympathetic fibers (Lv et al., 2014). In addition to Gli1 + MSCs, neural regulation of other MSC populations is worth further exploration. Second, most of the current reports did not further elucidate the exact mechanisms mediating neural regulation of MSCs in craniofacial bones. The majority of research explored the mechanisms merely on the level of specific nerves or molecules, while few studies figured out the signaling pathways underlying neural regulation of MSCs in craniofacial bone. Third, since the role of nerve fibers in the regulation of MSC in craniofacial bones has been gradually explored, research on the inverse regulation from stem cells on nerves may be valuable, which is relatively inadequate. Outside the area of craniofacial bones, nerve-stem cell crosstalk in skin regeneration and diseases has been studied. Signals from skin stem cell lineages specify a niche during development, inducing peripheral nerve fibers to innervate varied stem cell populations, and nerve fibers coordinate with skin stem cells to support tissue homeostasis and regeneration in adults (Peng et al., 2022). Fourth, previous research on the clinical application of craniofacial bone homeostasis and repair mainly focused on MSCs alone. Further exploration of neural regulation of MSCs may assist in modulation and treatment in the craniofacial bone diseases or injuries, such as the healing of craniosynostosis and the resolution of calvarial CSD repair.
Author contributions
H-JP: Conceptualization, Writing–original draft, Writing–review and editing. BH: Writing–original draft. QY: Funding acquisition, Writing–review and editing. J-JJ: Conceptualization, Funding acquisition, Writing–review and editing.
Funding
The author(s) declare that financial support was received for the research, authorship, and/or publication of this article. This work was supported by National Natural Science Foundation of China (82125006 to QY and 82370915 to J-JJ).
Conflict of interest
The authors declare that the research was conducted in the absence of any commercial or financial relationships that could be construed as a potential conflict of interest.
Publisher’s note
All claims expressed in this article are solely those of the authors and do not necessarily represent those of their affiliated organizations, or those of the publisher, the editors and the reviewers. Any product that may be evaluated in this article, or claim that may be made by its manufacturer, is not guaranteed or endorsed by the publisher.
References
Adameyko I., Fried K. (2016). The nervous system orchestrates and integrates craniofacial development: a review. Front. Physiol. 7, 49. doi:10.3389/fphys.2016.00049
Appelt J., Baranowsky A., Jahn D., Yorgan T., KöHLI P., Otto E., et al. (2020). The neuropeptide calcitonin gene-related peptide alpha is essential for bone healing. EBioMedicine 59, 102970. doi:10.1016/j.ebiom.2020.102970
Arkless K., Argunhan F., Brain S. D. (2019). CGRP discovery and timeline. Handb. Exp. Pharmacol. 255, 1–12. doi:10.1007/164_2018_129
Assefa F. (2023). The role of sensory and sympathetic nerves in craniofacial bone regeneration. Neuropeptides 99, 102328. doi:10.1016/j.npep.2023.102328
Berendsen A. D., Olsen B. R. (2015). Bone development. Bone 80, 14–18. doi:10.1016/j.bone.2015.04.035
Bi R., Yin Q., Li H., Yang X., Wang Y., Li Q., et al. (2023). A single-cell transcriptional atlas reveals resident progenitor cell niche functions in TMJ disc development and injury. Nat. Commun. 14, 830. doi:10.1038/s41467-023-36406-2
Bi R., Yin Q., Mei J., Chen K., Luo X., Fan Y., et al. (2020). Identification of human temporomandibular joint fibrocartilage stem cells with distinct chondrogenic capacity. Osteoarthr. Cartil. 28, 842–852. doi:10.1016/j.joca.2020.02.835
Bok S., Yallowitz A. R., Sun J., Mccormick J., Cung M., Hu L., et al. (2023). A multi-stem cell basis for craniosynostosis and calvarial mineralization. Nature 621, 804–812. doi:10.1038/s41586-023-06526-2
Chai Y., Jiang X., Ito Y., Bringas P., Han J., Rowitch D. H., et al. (2000). Fate of the mammalian cranial neural crest during tooth and mandibular morphogenesis. Development 127, 1671–1679. doi:10.1242/dev.127.8.1671
Chan C. K., Seo E. Y., Chen J. Y., Lo D., Mcardle A., Sinha R., et al. (2015). Identification and specification of the mouse skeletal stem cell. Cell 160, 285–298. doi:10.1016/j.cell.2014.12.002
Chan C. K. F., Gulati G. S., Sinha R., Tompkins J. V., Lopez M., Carter A. C., et al. (2018). Identification of the human skeletal stem cell. Cell 175, 43–56. doi:10.1016/j.cell.2018.07.029
Chen H., Hu B., Lv X., Zhu S., Zhen G., Wan M., et al. (2019). Prostaglandin E2 mediates sensory nerve regulation of bone homeostasis. Nat. Commun. 10, 181. doi:10.1038/s41467-018-08097-7
Chen J., Liu W., Zhao J., Sun C., Chen J., Hu K., et al. (2017). Gelatin microspheres containing calcitonin gene-related peptide or substance P repair bone defects in osteoporotic rabbits. Biotechnol. Lett. 39, 465–472. doi:10.1007/s10529-016-2263-4
Choi J. E., Di Nardo A. (2018). Skin neurogenic inflammation. Semin. Immunopathol. 40, 249–259. doi:10.1007/s00281-018-0675-z
Corr A., Smith J., Baldock P. (2017). Neuronal control of bone remodeling. Toxicol. Pathol. 45, 894–903. doi:10.1177/0192623317738708
Debnath S., Yallowitz A. R., Mccormick J., Lalani S., Zhang T., Xu R., et al. (2018). Discovery of a periosteal stem cell mediating intramembranous bone formation. Nature 562, 133–139. doi:10.1038/s41586-018-0554-8
Du Z., Wang L., Zhao Y., Cao J., Wang T., Liu P., et al. (2014). Sympathetic denervation-induced MSC mobilization in distraction osteogenesis associates with inhibition of MSC migration and osteogenesis by norepinephrine/adrb3. PLoS One 9, e105976. doi:10.1371/journal.pone.0105976
Elefteriou F. (2018). Impact of the autonomic nervous system on the skeleton. Physiol. Rev. 98, 1083–1112. doi:10.1152/physrev.00014.2017
Elefteriou F., Campbell P., Ma Y. (2014). Control of bone remodeling by the peripheral sympathetic nervous system. Calcif. Tissue Int. 94, 140–151. doi:10.1007/s00223-013-9752-4
Embree M. C., Chen M., Pylawka S., Kong D., Iwaoka G. M., Kalajzic I., et al. (2016). Exploiting endogenous fibrocartilage stem cells to regenerate cartilage and repair joint injury. Nat. Commun. 7, 13073. doi:10.1038/ncomms13073
Esposito A., Wang L., Li T., Miranda M., Spagnoli A. (2020). Role of Prx1-expressing skeletal cells and Prx1-expression in fracture repair. Bone 139, 115521. doi:10.1016/j.bone.2020.115521
Feng J., Jing J., Li J., Zhao H., Punj V., Zhang T., et al. (2017). BMP signaling orchestrates a transcriptional network to control the fate of mesenchymal stem cells in mice. Development 144, 2560–2569. doi:10.1242/dev.150136
Freddi T. A. L., Ottaiano A. C., Lucio L. L., CorrêA D. G., Hygino Da Cruz L. C. (2022). The trigeminal nerve: anatomy and pathology. Semin. Ultrasound CT MR 43, 403–413. doi:10.1053/j.sult.2022.04.002
Gadomski S., Fielding C., GarcíA-GarcíA A., Korn C., Kapeni C., Ashraf S., et al. (2022). A cholinergic neuroskeletal interface promotes bone formation during postnatal growth and exercise. Cell Stem Cell 29, 528–544.e9. doi:10.1016/j.stem.2022.02.008
GräSSEL S. G. (2014). The role of peripheral nerve fibers and their neurotransmitters in cartilage and bone physiology and pathophysiology. Arthritis Res. Ther. 16, 485. doi:10.1186/s13075-014-0485-1
Hass R., Kasper C., BöHM S., Jacobs R. (2011). Different populations and sources of human mesenchymal stem cells (MSC): a comparison of adult and neonatal tissue-derived MSC. Cell Commun. Signal 9, 12. doi:10.1186/1478-811X-9-12
Hayano S., Fukui Y., Kawanabe N., Kono K., Nakamura M., Ishihara Y., et al. (2018). Role of the inferior alveolar nerve in rodent lower incisor stem cells. J. Dent. Res. 97, 954–961. doi:10.1177/0022034518758244
Hayashi M., Nakashima T., Taniguchi M., Kodama T., Kumanogoh A., Takayanagi H. (2012). Osteoprotection by semaphorin 3A. Nature 485, 69–74. doi:10.1038/nature11000
Hempstead B. L., Martin-Zanca D., Kaplan D. R., Parada L. F., Chao M. V. (1991). High-affinity NGF binding requires coexpression of the trk proto-oncogene and the low-affinity NGF receptor. Nature 350, 678–683. doi:10.1038/350678a0
Hong H. S., Lee J., Lee E., Kwon Y. S., Lee E., Ahn W., et al. (2009). A new role of substance P as an injury-inducible messenger for mobilization of CD29(+) stromal-like cells. Nat. Med. 15, 425–435. doi:10.1038/nm.1909
Huang X., Li Z., Liu P., Wu M., Liu A. Q., Hu C., et al. (2021). Gli1(+) cells residing in bone sutures respond to mechanical force via IP(3)R to mediate osteogenesis. Stem Cells Int. 2021, 8138374. doi:10.1155/2021/8138374
Jiang W. D., Zhu P. Q., Zhang T., Liao F. C., Jiang P. P., Zhou N., et al. (2023). PRRX1(+)MSCs enhance mandibular regeneration during distraction osteogenesis. J. Dent. Res. 102, 1058–1068. doi:10.1177/00220345231176522
Jiang W., Wang H., Li Y. S., Luo W. (2016). Role of vasoactive intestinal peptide in osteoarthritis. J. Biomed. Sci. 23, 63. doi:10.1186/s12929-016-0280-1
Jin A., Xu H., Gao X., Sun S., Yang Y., Huang X., et al. (2023). ScRNA-seq reveals a distinct osteogenic progenitor of alveolar bone. J. Dent. Res. 102, 645–655. doi:10.1177/00220345231159821
Jing D., Chen Z., Men Y., Yi Y., Wang Y., Wang J., et al. (2022). Response of Gli1(+) suture stem cells to mechanical force upon suture expansion. J. Bone Min. Res. 37, 1307–1320. doi:10.1002/jbmr.4561
Jones R. E., Salhotra A., Robertson K. S., Ransom R. C., Foster D. S., Shah H. N., et al. (2019). Skeletal stem cell-schwann cell circuitry in mandibular repair. Cell Rep. 28, 2757–2766. doi:10.1016/j.celrep.2019.08.021
Kamei H., Ishii T., Nishii Y. (2022). Semaphorin 3A regulates alveolar bone remodeling on orthodontic tooth movement. Sci. Rep. 12, 9243. doi:10.1038/s41598-022-13217-x
Karsenty G. (2003). The complexities of skeletal biology. Nature 423, 316–318. doi:10.1038/nature01654
Kaukua N., Shahidi M. K., Konstantinidou C., Dyachuk V., Kaucka M., Furlan A., et al. (2014). Glial origin of mesenchymal stem cells in a tooth model system. Nature 513, 551–554. doi:10.1038/nature13536
Korsensky L., Ron D. (2016). Regulation of FGF signaling: recent insights from studying positive and negative modulators. Semin. Cell Dev. Biol. 53, 101–114. doi:10.1016/j.semcdb.2016.01.023
La Noce M., Mele L., Tirino V., Paino F., De Rosa A., Naddeo P., et al. (2014). Neural crest stem cell population in craniomaxillofacial development and tissue repair. Eur. Cell Mater 28, 348–357. doi:10.22203/ecm.v028a24
Lei J., Chen S., Jing J., Guo T., Feng J., Ho T. V., et al. (2022). Inhibiting hh signaling in Gli1(+) osteogenic progenitors alleviates TMJOA. J. Dent. Res. 101, 664–674. doi:10.1177/00220345211059079
Levato R., Webb W. R., Otto I. A., Mensinga A., Zhang Y., Van Rijen M., et al. (2017). The bio in the ink: cartilage regeneration with bioprintable hydrogels and articular cartilage-derived progenitor cells. Acta Biomater. 61, 41–53. doi:10.1016/j.actbio.2017.08.005
Li J., Wang T., Li C., Wang Z., Wang P., Zheng L. (2020). Sema3A and HIF1α co-overexpressed iPSC-MSCs/HA scaffold facilitates the repair of calvarial defect in a mouse model. J. Cell Physiol. 235, 6754–6766. doi:10.1002/jcp.29569
Li Q., Xu R., Lei K., Yuan Q. (2022a). Insights into skeletal stem cells. Bone Res. 10, 61. doi:10.1038/s41413-022-00235-8
Li S., Li Z., Yang J., Ha Y., Zhou X., He C. (2022b). Inhibition of sympathetic activation by delivering calcium channel blockers from a 3D printed scaffold to promote bone defect repair. Adv. Healthc. Mater 11, e2200785. doi:10.1002/adhm.202200785
Liu H., Li P., Zhang S., Xiang J., Yang R., Liu J., et al. (2022). Prrx1 marks stem cells for bone, white adipose tissue and dermis in adult mice. Nat. Genet. 54, 1946–1958. doi:10.1038/s41588-022-01227-4
Liu L., Wang J., Song X., Zhu Q., Shen S., Zhang W. (2018a). Semaphorin 3A promotes osteogenic differentiation in human alveolar bone marrow mesenchymal stem cells. Exp. Ther. Med. 15, 3489–3494. doi:10.3892/etm.2018.5813
Liu X., Tan N., Zhou Y., Zhou X., Chen H., Wei H., et al. (2016). Semaphorin 3A shifts adipose mesenchymal stem cells towards osteogenic phenotype and promotes bone regeneration in vivo. Stem Cells Int. 2016, 2545214. doi:10.1155/2016/2545214
Liu Y., Zheng G., Liu L., Wang Z., Wang Y., Chen Q., et al. (2018b). Inhibition of osteogenesis surrounding the titanium implant by CGRP deficiency. Connect. Tissue Res. 59, 147–156. doi:10.1080/03008207.2017.1317759
Li Z., Li D., Rao Y., Wei L., Liu M., Zheng G., et al. (2021). SCAP knockout in SM22α-Cre mice induces defective angiogenesis in the placental labyrinth. Biomed. Pharmacother. 133, 111011. doi:10.1016/j.biopha.2020.111011
Li Z., Meyers C. A., Chang L., Lee S., Li Z., Tomlinson R., et al. (2019). Fracture repair requires TrkA signaling by skeletal sensory nerves. J. Clin. Invest. 129, 5137–5150. doi:10.1172/JCI128428
Lv X., Wu Z., Li Y. (2014). Innervation of the cerebral dura mater. Neuroradiol. J. 27, 293–298. doi:10.15274/NRJ-2014-10052
Maruyama T., Jeong J., Sheu T. J., Hsu W. (2016). Stem cells of the suture mesenchyme in craniofacial bone development, repair and regeneration. Nat. Commun. 7, 10526. doi:10.1038/ncomms10526
Maruyama T., Stevens R., Boka A., Dirienzo L., Chang C., Yu H. I., et al. (2021). BMPR1A maintains skeletal stem cell properties in craniofacial development and craniosynostosis. Sci. Transl. Med. 13, eabb4416. doi:10.1126/scitranslmed.abb4416
Maryanovich M., Zahalka A. H., Pierce H., Pinho S., Nakahara F., Asada N., et al. (2018). Adrenergic nerve degeneration in bone marrow drives aging of the hematopoietic stem cell niche. Nat. Med. 24, 782–791. doi:10.1038/s41591-018-0030-x
Mei H., Li Z., Lv Q., Li X., Wu Y., Feng Q., et al. (2024). Sema3A secreted by sensory nerve induces bone formation under mechanical loads. Int. J. Oral Sci. 16, 5. doi:10.1038/s41368-023-00269-6
MéNDEZ-Ferrer S., Michurina T. V., Ferraro F., Mazloom A. R., Macarthur B. D., Lira S. A., et al. (2010). Mesenchymal and haematopoietic stem cells form a unique bone marrow niche. Nature 466, 829–834. doi:10.1038/nature09262
Meyers C. A., Lee S., Sono T., Xu J., Negri S., Tian Y., et al. (2020). A neurotrophic mechanism directs sensory nerve transit in cranial bone. Cell Rep. 31, 107696. doi:10.1016/j.celrep.2020.107696
Miki K., Takeshita N., Yamashita M., Kitamura M., Murakami S. (2024). Calcitonin gene-related peptide regulates periodontal tissue regeneration. Sci. Rep. 14, 1344. doi:10.1038/s41598-024-52029-z
Muhl L., Genové G., Leptidis S., Liu J., He L., Mocci G., et al. (2020). Single-cell analysis uncovers fibroblast heterogeneity and criteria for fibroblast and mural cell identification and discrimination. Nat. Commun. 11, 3953. doi:10.1038/s41467-020-17740-1
Naji A., Eitoku M., Favier B., Deschaseaux F., Rouas-Freiss N., Suganuma N. (2019). Biological functions of mesenchymal stem cells and clinical implications. Cell Mol. Life Sci. 76, 3323–3348. doi:10.1007/s00018-019-03125-1
Palla S. (2016). “Chapter 6 - anatomy and pathophysiology of the temporomandibular joint,” in Functional occlusion in restorative dentistry and prosthodontics. Editors I. KLINEBERG, and S. E. ECKERT (Mosby).
Pang P., Shimo T., Takada H., Matsumoto K., Yoshioka N., Ibaragi S., et al. (2015). Expression pattern of sonic hedgehog signaling and calcitonin gene-related peptide in the socket healing process after tooth extraction. Biochem. Biophys. Res. Commun. 467, 21–26. doi:10.1016/j.bbrc.2015.09.139
Park M. H., Jin H. K., Min W. K., Lee W. W., Lee J. E., Akiyama H., et al. (2015). Neuropeptide Y regulates the hematopoietic stem cell microenvironment and prevents nerve injury in the bone marrow. Embo J. 34, 1648–1660. doi:10.15252/embj.201490174
Park S., Zhao H., Urata M., Chai Y. (2016). Sutures possess strong regenerative capacity for calvarial bone injury. Stem Cells Dev. 25, 1801–1807. doi:10.1089/scd.2016.0211
Pei F., Ma L., Guo T., Zhang M., Jing J., Wen Q., et al. (2024). Sensory nerve regulates progenitor cells via FGF-SHH axis in tooth root morphogenesis. Development 151, dev202043. doi:10.1242/dev.202043
Pei F., Ma L., Jing J., Feng J., Yuan Y., Guo T., et al. (2023). Sensory nerve niche regulates mesenchymal stem cell homeostasis via FGF/mTOR/autophagy axis. Nat. Commun. 14, 344. doi:10.1038/s41467-023-35977-4
Peng J., Chen H., Zhang B. (2022). Nerve-stem cell crosstalk in skin regeneration and diseases. Trends Mol. Med. 28, 583–595. doi:10.1016/j.molmed.2022.04.005
Russell F. A., King R., Smillie S. J., Kodji X., Brain S. D. (2014). Calcitonin gene-related peptide: physiology and pathophysiology. Physiol. Rev. 94, 1099–1142. doi:10.1152/physrev.00034.2013
Schneider R. K., Mullally A., Dugourd A., Peisker F., Hoogenboezem R., Van Strien P. M. H., et al. (2017). Gli1(+) mesenchymal stromal cells are a Key driver of bone marrow fibrosis and an important cellular therapeutic target. Cell Stem Cell 20, 785–800. doi:10.1016/j.stem.2017.03.008
Sena I. F. G., Prazeres P., Santos G. S. P., Borges I. T., Azevedo P. O., Andreotti J. P., et al. (2017). Identity of Gli1(+) cells in the bone marrow. Exp. Hematol. 54, 12–16. doi:10.1016/j.exphem.2017.06.349
Şen S., Lux C. J., Erber R. (2021). A potential role of semaphorin 3A during orthodontic tooth movement. Int. J. Mol. Sci. 22, 8297. doi:10.3390/ijms22158297
Shi L., Feng L., Zhu M. L., Yang Z. M., Wu T. Y., Xu J., et al. (2020). Vasoactive intestinal peptide stimulates bone marrow-mesenchymal stem cells osteogenesis differentiation by activating wnt/β-catenin signaling pathway and promotes rat skull defect repair. Stem Cells Dev. 29, 655–666. doi:10.1089/scd.2019.0148
Shi Y., He G., Lee W. C., Mckenzie J. A., Silva M. J., Long F. (2017). Gli1 identifies osteogenic progenitors for bone formation and fracture repair. Nat. Commun. 8, 2043. doi:10.1038/s41467-017-02171-2
Skaper S. D. (2017). Nerve growth factor: a neuroimmune crosstalk mediator for all seasons. Immunology 151, 1–15. doi:10.1111/imm.12717
Soldatov R., Kaucka M., Kastriti M. E., Petersen J., Chontorotzea T., Englmaier L., et al. (2019). Spatiotemporal structure of cell fate decisions in murine neural crest. Science 364, eaas9536. doi:10.1126/science.aas9536
Stocum D. L., Roberts W. E. (2018). Part I: development and physiology of the temporomandibular joint. Curr. Osteoporos. Rep. 16, 360–368. doi:10.1007/s11914-018-0447-7
Sun Q., Huang J., Tian J., Lv C., Li Y., Yu S., et al. (2024). Key roles of Gli1 and ihh signaling in craniofacial development. Stem Cells Dev. 33, 251–261. doi:10.1089/scd.2024.0036
Tevlin R., Griffin M., Chen K., Januszyk M., Guardino N., Spielman A., et al. (2023). Denervation during mandibular distraction osteogenesis results in impaired bone formation. Sci. Rep. 13, 2097. doi:10.1038/s41598-023-27921-9
Tian L., Sheng Y., Huang L., Chow D. H., Chau W. H., Tang N., et al. (2018). An innovative Mg/Ti hybrid fixation system developed for fracture fixation and healing enhancement at load-bearing skeletal site. Biomaterials 180, 173–183. doi:10.1016/j.biomaterials.2018.07.018
Tomlinson R. E., Christiansen B. A., Giannone A. A., Genetos D. C. (2020). The role of nerves in skeletal development, adaptation, and aging. Front. Endocrinol. (Lausanne) 11, 646. doi:10.3389/fendo.2020.00646
Tomlinson R. E., Li Z., Zhang Q., Goh B. C., Li Z., Thorek D. L. J., et al. (2016). NGF-TrkA signaling by sensory nerves coordinates the vascularization and ossification of developing endochondral bone. Cell Rep. 16, 2723–2735. doi:10.1016/j.celrep.2016.08.002
Tong W., Geng Y., Huang Y., Shi Y., Xiang S., Zhang N., et al. (2015). In vivo identification and induction of articular cartilage stem cells by inhibiting NF-κB signaling in osteoarthritis. Stem Cells 33, 3125–3137. doi:10.1002/stem.2124
Tower R. J., Li Z., Cheng Y. H., Wang X. W., Rajbhandari L., Zhang Q., et al. (2021). Spatial transcriptomics reveals a role for sensory nerves in preserving cranial suture patency through modulation of BMP/TGF-β signaling. Proc. Natl. Acad. Sci. U. S. A. 118, e2103087118. doi:10.1073/pnas.2103087118
Wang X. D., Li S. Y., Zhang S. J., Gupta A., Zhang C. P., Wang L. (2020a). The neural system regulates bone homeostasis via mesenchymal stem cells: a translational approach. Theranostics 10, 4839–4850. doi:10.7150/thno.43771
Wang Y. X., Zhao Z. D., Wang Q., Li Z. L., Huang Y., Zhao S., et al. (2020b). Biological potential alterations of migratory chondrogenic progenitor cells during knee osteoarthritic progression. Arthritis Res. Ther. 22, 62. doi:10.1186/s13075-020-2144-z
Wang L., Han L., Xue P., Hu X., Wong S. W., Deng M., et al. (2021). Dopamine suppresses osteoclast differentiation via cAMP/PKA/CREB pathway. Cell Signal 78, 109847. doi:10.1016/j.cellsig.2020.109847
Wang L., Zhao R., Shi X., Wei T., Halloran B. P., Clark D. J., et al. (2009). Substance P stimulates bone marrow stromal cell osteogenic activity, osteoclast differentiation, and resorption activity in vitro. Bone 45, 309–320. doi:10.1016/j.bone.2009.04.203
Wee N. K. Y., Novak S., Ghosh D., Root S. H., Dickerson I. M., Kalajzic I. (2023). Inhibition of CGRP signaling impairs fracture healing in mice. J. Orthop. Res. 41, 1228–1239. doi:10.1002/jor.25474
Weng Y., Wang H., Wu D., Xu S., Chen X., Huang J., et al. (2022). A novel lineage of osteoprogenitor cells with dual epithelial and mesenchymal properties govern maxillofacial bone homeostasis and regeneration after MSFL. Cell Res. 32, 814–830. doi:10.1038/s41422-022-00687-x
Wilk K., Yeh S. A., Mortensen L. J., Ghaffarigarakani S., Lombardo C. M., Bassir S. H., et al. (2017). Postnatal calvarial skeletal stem cells expressing PRX1 reside exclusively in the calvarial sutures and are required for bone regeneration. Stem Cell Rep. 8, 933–946. doi:10.1016/j.stemcr.2017.03.002
Wu B., Wang L., Yang X., Mao M., Ye C., Liu P., et al. (2016a). Norepinephrine inhibits mesenchymal stem cell chemotaxis migration by increasing stromal cell-derived factor-1 secretion by vascular endothelial cells via NE/abrd3/JNK pathway. Exp. Cell Res. 349, 214–220. doi:10.1016/j.yexcr.2016.09.007
Wu K., Huang D., Huang X. (2023a). The effects of semaphorin 3A in bone and cartilage metabolism: fundamental mechanism and clinical potential. Front. Cell Dev. Biol. 11, 1321151. doi:10.3389/fcell.2023.1321151
Wu Q., Yang B., Cao C., Guang M., Gong P. (2016b). Age-dependent impact of inferior alveolar nerve transection on mandibular bone metabolism and the underlying mechanisms. J. Mol. Histol. 47, 579–586. doi:10.1007/s10735-016-9697-9
Wu Y., Zhang Q., Zhao B., Wang X. (2021). Effect and mechanism of propranolol on promoting osteogenic differentiation and early implant osseointegration. Int. J. Mol. Med. 48, 191. doi:10.3892/ijmm.2021.5024
Wu Z., Wang X., Shi J., Gupta A., Zhang Y., Zhang B., et al. (2023b). Identification of functional modules and Key pathways associated with innervation in graft bone-CGRP regulates the differentiation of bone marrow mesenchymal stem cells via p38 MAPK and wnt6/β-catenin. Stem Cells Int. 2023, 1154808. doi:10.1155/2023/1154808
Xiao Y., Han C., Wang Y., Zhang X., Bao R., Li Y., et al. (2023). Interoceptive regulation of skeletal tissue homeostasis and repair. Bone Res. 11, 48. doi:10.1038/s41413-023-00285-6
Xie M., Kamenev D., Kaucka M., Kastriti M. E., Zhou B., Artemov A. V., et al. (2019). Schwann cell precursors contribute to skeletal formation during embryonic development in mice and zebrafish. Proc. Natl. Acad. Sci. U. S. A. 116, 15068–15073. doi:10.1073/pnas.1900038116
Xu J., Li Z., Tower R. J., Negri S., Wang Y., Meyers C. A., et al. (2022a). NGF-p75 signaling coordinates skeletal cell migration during bone repair. Sci. Adv. 8, eabl5716. doi:10.1126/sciadv.abl5716
Xu J., Zhang Z., Zhao J., Meyers C. A., Lee S., Qin Q., et al. (2022b). Interaction between the nervous and skeletal systems. Front. Cell Dev. Biol. 10, 976736. doi:10.3389/fcell.2022.976736
Ye L., Xu J., Mi J., He X., Pan Q., Zheng L., et al. (2021). Biodegradable magnesium combined with distraction osteogenesis synergistically stimulates bone tissue regeneration via CGRP-FAK-VEGF signaling axis. Biomaterials 275, 120984. doi:10.1016/j.biomaterials.2021.120984
Yin J. Q., Zhu J., Ankrum J. A. (2019). Manufacturing of primed mesenchymal stromal cells for therapy. Nat. Biomed. Eng. 3, 90–104. doi:10.1038/s41551-018-0325-8
Yin Q., Bi R., Li H., Li Q., Li P., Wang R., et al. (2023). Regulatory role of human fibrocartilage stem cells in condyle osteochondroma. Cell Prolif. 56, e13342. doi:10.1111/cpr.13342
Yi Y., Stenberg W., Luo W., Feng J. Q., Zhao H. (2022). Alveolar bone marrow Gli1+ stem cells support implant osseointegration. J. Dent. Res. 101, 73–82. doi:10.1177/00220345211013722
Yu M., Ma L., Yuan Y., Ye X., Montagne A., He J., et al. (2021). Cranial suture regeneration mitigates skull and neurocognitive defects in craniosynostosis. Cell 184, 243–256.e18. doi:10.1016/j.cell.2020.11.037
Yu X., Liu S., Chen X., Du Y., Yin X., Du Y., et al. (2019). Calcitonin gene related peptide gene-modified rat bone mesenchymal stem cells are effective seed cells in tissue engineering to repair skull defects. Histol. Histopathol. 34, 1229–1241. doi:10.14670/HH-18-102
Zhang Y. B., Wang L., Jia S., Du Z. J., Zhao Y. H., Liu Y. P., et al. (2014). Local injection of substance P increases bony formation during mandibular distraction osteogenesis in rats. Br. J. Oral Maxillofac. Surg. 52, 697–702. doi:10.1016/j.bjoms.2014.07.002
Zhang D., Zhang S., Wang J., Li Q., Xue H., Sheng R., et al. (2020a). LepR-expressing stem cells are essential for alveolar bone regeneration. J. Dent. Res. 99, 1279–1286. doi:10.1177/0022034520932834
Zhang N., Barrell W. B., Liu K. J. (2023a). Identification of distinct subpopulations of Gli1-lineage cells in the mouse mandible. J. Anat. 243, 90–99. doi:10.1111/joa.13858
Zhang X., Yan X., Cao J., Yang Z., Cao X., Zhang Y., et al. (2020b). SM22α(+) vascular mural cells are essential for vessel stability in tumors and undergo phenotype transition regulated by Notch signaling. J. Exp. Clin. Cancer Res. 39, 124. doi:10.1186/s13046-020-01630-x
Zhang Y., An S., Hao J., Tian F., Fang X., Wang J. (2018). Systemic injection of substance P promotes murine calvarial repair through mobilizing endogenous mesenchymal stem cells. Sci. Rep. 8, 12996. doi:10.1038/s41598-018-31414-5
Zhang Y., Xu J., Ruan Y. C., Yu M. K., O’Laughlin M., Wise H., et al. (2016). Implant-derived magnesium induces local neuronal production of CGRP to improve bone-fracture healing in rats. Nat. Med. 22, 1160–1169. doi:10.1038/nm.4162
Zhang Z., Wang F., Huang X., Sun H., Xu J., Qu H., et al. (2023b). Engineered sensory nerve guides self-adaptive bone healing via NGF-TrkA signaling pathway. Adv. Sci. (Weinh) 10, e2206155. doi:10.1002/advs.202206155
Zhao H., Chai Y. (2015). Stem cells in teeth and craniofacial bones. J. Dent. Res. 94, 1495–1501. doi:10.1177/0022034515603972
Zhao H., Feng J., Ho T. V., Grimes W., Urata M., Chai Y. (2015). The suture provides a niche for mesenchymal stem cells of craniofacial bones. Nat. Cell Biol. 17, 386–396. doi:10.1038/ncb3139
Zhao H., Feng J., Seidel K., Shi S., Klein O., Sharpe P., et al. (2014). Secretion of shh by a neurovascular bundle niche supports mesenchymal stem cell homeostasis in the adult mouse incisor. Cell Stem Cell 14, 160–173. doi:10.1016/j.stem.2013.12.013
Zheng M. G., Sui W. Y., He Z. D., Liu Y., Huang Y. L., Mu S. H., et al. (2019). TrkA regulates the regenerative capacity of bone marrow stromal stem cells in nerve grafts. Neural Regen. Res. 14, 1765–1771. doi:10.4103/1673-5374.257540
Zheng M., Duan J., He Z., Wang Z., Mu S., Zeng Z., et al. (2016). Overexpression of tropomyosin receptor kinase A improves the survival and Schwann-like cell differentiation of bone marrow stromal cells in nerve grafts for bridging rat sciatic nerve defects. Cytotherapy 18, 1256–1269. doi:10.1016/j.jcyt.2016.06.015
Zhou B. O., Yue R., Murphy M. M., Peyer J. G., Morrison S. J. (2014). Leptin-receptor-expressing mesenchymal stromal cells represent the main source of bone formed by adult bone marrow. Cell Stem Cell 15, 154–168. doi:10.1016/j.stem.2014.06.008
Keywords: craniofacial bone, mesenchymal stem cell, nerve, development, homeostasis, repair
Citation: Pi H-J, Huang B, Yuan Q and Jing J-J (2024) Neural regulation of mesenchymal stem cells in craniofacial bone: development, homeostasis and repair. Front. Physiol. 15:1423539. doi: 10.3389/fphys.2024.1423539
Received: 26 April 2024; Accepted: 15 July 2024;
Published: 29 July 2024.
Edited by:
Gael Y. Rochefort, Université de Tours, FranceReviewed by:
Jiewen Dai, Shanghai Jiao Tong University, ChinaJingwen Yang, Wuhan University, China
Liqiang Zhang, The Second Affiliated Hospital of Xi’an Jiaotong University, China
Hironori Hojo, The University of Tokyo, Japan
Qinggang Dai, Shanghai Jiao Tong University, China
Copyright © 2024 Pi, Huang, Yuan and Jing. This is an open-access article distributed under the terms of the Creative Commons Attribution License (CC BY). The use, distribution or reproduction in other forums is permitted, provided the original author(s) and the copyright owner(s) are credited and that the original publication in this journal is cited, in accordance with accepted academic practice. No use, distribution or reproduction is permitted which does not comply with these terms.
*Correspondence: Quan Yuan, eXVhbnF1YW5Ac2N1LmVkdS5jbg==; Jun-Jun Jing, ampqODg2NTQzQDEyNi5jb20=
†These authors have contributed equally to this work and share last authorship