- 1Postgraduate Program in Production Engineering, Federal University of Santa Maria, Santa Maria, Brazil
- 2Elastro Crete, LLC. Research and Development Department, Veyo, UT, United States
- 3Department of Administrative Sciences, Federal University of Santa Maria, Santa Maria, Brazil
- 4Production Engineering Department, Federal University of Santa Maria, Santa Maria, Brazil
Background: Carbon dioxide (CO2), traditionally viewed as a mere byproduct of cellular respiration, plays a multifaceted role in human physiology beyond simple elimination through respiration. CO2 may regulate the tumor microenvironment by significantly affecting the release of oxygen (O2) to tissues through the Bohr effect and by modulating blood pH and vasodilation. Previous studies suggest hypercapnia (elevated CO2 levels) might trigger optimized cellular mechanisms with potential therapeutic benefits. The role of CO2 in cellular stress conditions within tumor environments and its impact on O2 utilization offers a new investigative area in oncology.
Objectives: This study aims to explore CO2’s role in the tumor environment, particularly how its physiological properties and adaptive responses can influence therapeutic strategies.
Methods: By applying a structured translational approach using the Work Breakdown Structure method, the study divided the analysis into six interconnected work packages to comprehensively analyze the interactions between carbon dioxide and the tumor microenvironment. Methods included systematic literature reviews, data analyses, data integration for identifying critical success factors and exploring extracellular environment modulation. The research used SMART criteria for assessing innovation and the applicability of results.
Results: The research revealed that the human body’s adaptability to hypercapnic conditions could potentially inform innovative strategies for manipulating the tumor microenvironment. This could enhance O2 utilization efficiency and manage adaptive responses to cellular stress. The study proposed that carbon dioxide’s hormetic potential could induce beneficial responses in the tumor microenvironment, prompting clinical protocols for experimental validation. The research underscored the importance of pH regulation, emphasizing CO2 and carbonic acid’s role in modulating metabolic and signaling pathways related to cancer.
Conclusion: The study underscores CO2 as vital to our physiology and suggests potential therapeutic uses within the tumor microenvironment. pH modulation and cellular oxygenation optimization via CO2 manipulation could offer innovative strategies to enhance existing cancer therapies. These findings encourage further exploration of CO2’s therapeutic potential. Future research should focus on experimental validation and exploration of clinical applications, emphasizing the need for interdisciplinary and collaborative approaches to tackle current challenges in cancer treatment.
1 Introduction
Carbon dioxide (CO2), a basic metabolic byproduct of cellular respiration, has important roles beyond simple elimination through the lungs. Despite its small quantity, it greatly influences the efficiency of the respiratory system and aerobic metabolism. Through the Bohr effect, CO2 affects oxygen’s (O2) release to tissues, thereby altering hemoglobin’s affinity for O2. This, along with its role in adjusting blood pH and cardiovascular functions such as vasodilation and blood pressure, highlights its physiological significance (Hall and Hall, 2020; Feinstein et al., 2022; Doyle and Cooper, 2024). In the past, CO2 has been linked to various therapeutic techniques, including as one of the first anesthetics (Bohr et al., 1904; Rose, 1905; Henderson, 1940; Buess, 1953). Currently, statements such as Henderson’s (1940) claim, “CO2 is the primary parahormone of the whole body; it is the only one produced by all tissues and likely impacts every organ. CO2 is, in fact, a more essential component of living matter than O2,” are being reexamined in modern medicine. This underscores a renewed interest in understanding the various aspects of CO2, from its essential physiological functions to its potential negative implications. This has led to ongoing debates considering whether CO2 is a friend or foe in the context of human health.
Beyond its physiological role, CO2 has been studied in extreme situations where human adaptation to high CO2 levels, such as those experienced by deep-sea divers and mountaineers, showcases the body’s capacity to adapt to hypercapnia conditions. Studies show that this adaptation can lead to improved efficiency in O2 usage, indicating optimized cellular mechanisms for O2 delivery and use under stress (Florio et al., 1979; Earing et al., 2014; Bailey et al., 2017). This shows the human respiratory and cardiovascular system’s flexibility and resilience under intense physiological challenges.
These findings parallel the tumor microenvironment, characterized by hypoxemia, alkalosis, acidosis, and intense cellular stress (Bożyk et al., 2022). Human adaptability to high CO2 levels suggests possible strategies to manipulate the tumor microenvironment to enhance current treatments and devise new ones. Given the tumor microenvironment’s complexity and the central role of cellular stress in tumor growth (Redding and Gabocka, 2023), investigating the hormetic potential of CO2—the ability of stress at optimal levels to induce a beneficial response—becomes a promising area of investigation. This concept underscores the need to understand the fundamental biology behind the human body’s responses to CO2. This is especially important given that most therapies targeting the tumor microenvironment, excluding immunotherapies, continue to face challenges in proving efficacy (Xiao and Yu, 2021).
In line with this understanding, this study aims to analyze the role of CO2 in the context of the tumor microenvironment. Specifically, it will focus on how the physiological properties of CO2 and adaptive responses can be applied or simulated in therapeutic strategies. It seeks to uncover new approaches through an enhanced understanding of CO2’s complex role in cellular physiology and its impact on tumor cell behavior. This comprehensive backdrop guides our methodological strategy, designed to dissect CO2's nuanced roles within the tumor microenvironment and uncover groundbreaking therapeutic avenues.
2 Methods
This detailed understanding of CO2’s physiological roles sets the stage for our methodology, where we employ a structured translational approach to delve deeper into CO2's interaction with the tumor microenvironment, aiming to uncover its therapeutic potential. Translational Research was employed, using the Work Breakdown Structure (WBS) method as advised by the Project Management Institute. The translational research method, detailed below, is meticulously devised to unravel the intricate interactions of CO2 within the tumor microenvironment, directly addressing the inquiries posed in the Introduction and laying a foundation for the ensuing analysis. This approach subdivided the research process into six cohesive Work Packages (WPs), each addressing specific facets of CO2 interaction with the tumor microenvironment for a comprehensive, interdisciplinary view. A detailed qualitative assessment of the results will follow each WP to confirm their clinical relevance and application.
The goal of WP1 (Management and Supervision) was to run the project efficiently, adhere to the predefined goals and timeline, stimulate collaboration by utilizing Open Innovation to review and adjust each WP as the project unfolds, ensure effective communication among the WPs, and evaluate the significance of new medical hypotheses.
WP2 (CO2 Data Analysis) aimed to produce a current theoretical framework on CO2. This aim was achieved through a systematic literature review, which gathered data from the past decade in relation to the human species with descriptors such as “carbon dioxide therapy,” “carbon dioxide and inhalation,” “therapeutic hypercapnia,” “carbogen and breathing,” “carbonic acid,” “physiological adaptation and carbon dioxide or carbonic acid,” and “increased carbon dioxide respiration and cancer.” The results amounted to 108 articles for thorough analysis (Figure 1).
The specific objective of WP3 (Tumor Microenvironment Data Analysis) was to select primary study targets influencing new cancer therapies and prepare for WP4. This led to the selection of Fundamental Viewpoint Factors according to the multi-criteria decision approach of Bana e Costa et al. (1999). Another systematic literature review was conducted with descriptors, such as “tumor microenvironment,” using filters for the past 5 years, human species, and meta-analysis-type publications. The output was 46 articles for detailed analysis (Figure 2).
The primary aim of WP4 (Data Integration) was to identify Critical Success Factors (CSFs) for merging knowledge obtained from prior WPs based on a multicriteria decision-support method inspired by Bana e Costa et al. (1999). This process was vital for data synthesis and interpretation, establishing a foundation for practical applications. The CSFs were then authenticated through an integrative literature review (Table 1), with selection criteria adapted from Khan et al. (2011), which emphasized the wide-ranging inclusion of translational data.
The key objective of WP5 (Modulation of the Extracellular Environment) was to investigate how integrating theory and data could impact the tumor’s microenvironment. Design Thinking and Open Innovation were used to bridge theoretical discoveries with practical applications (Chesbrough, 2003; Brown, 2008). This phase includes a plan for empirically validating the findings through case studies.
The primary goal of WP6 (Publication of the Results) is to assess the outcomes’ inventiveness and applicability using SMART criteria (Drucker, 1954) and FINER criteria (Hulley et al., 2007) to create innovative clinical solutions. Additionally, we will address the methodological restrictions and ethical elements related to the study, especially concerning the use of human patient data, to ensure the study’s transparency and repeatability. This methodological design signifies an adherence to accuracy, repeatability, and ethics in research that lays a strong groundwork for investigating the therapeutic possibilities of CO2 in cancer treatment. Our structured analytical journey, outlined in the methodology, paves the way for the findings we present. These results not only validate our investigative approach but also open new vistas in understanding CO2's therapeutic dimension.
3 Results
Applying the structured approach outlined, we systematically explored CO2's multifaceted impact on the tumor microenvironment. The following results illuminate the adaptability of human physiology to CO2 variations and its implications for therapeutic strategies. The findings presented herein vividly illustrate the influence of CO2 and carbonic acid on cellular physiology and the tumor microenvironment, directly correlating with our methodological objectives and bridging the gap to the comprehensive discussion that follows. To optimize the presentation of the results, the key points obtained through WPs one to five are listed in Table 2.
For WP2, it is crucial to understand the basic technical knowledge needed to grasp the potential of biological and therapeutic interaction with carbon dioxide, covered across various topics in Table 3.
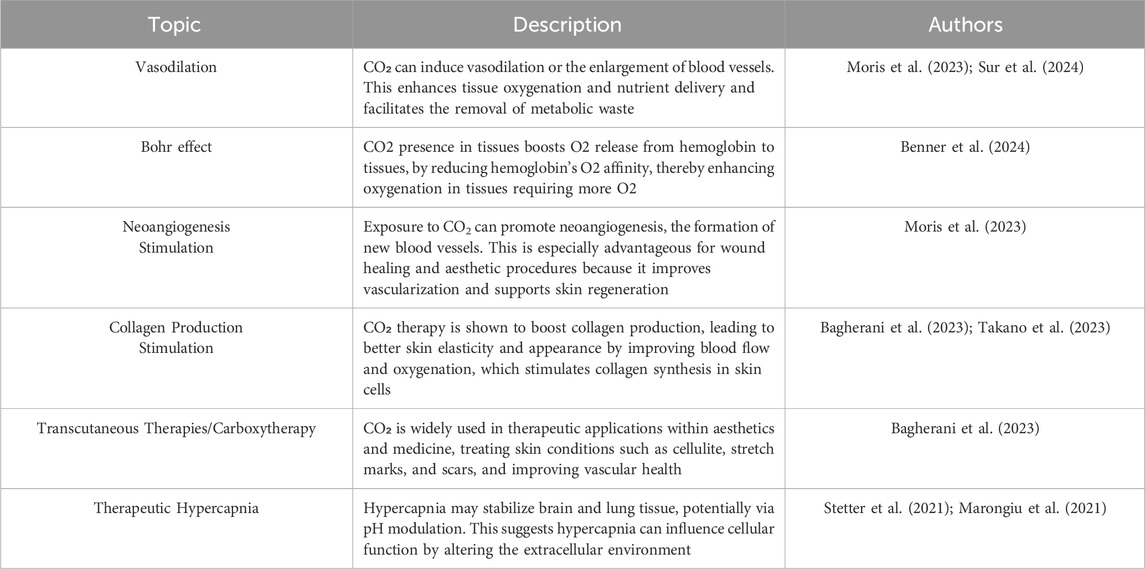
Table 3. List of basic technical knowledge required for understanding the biological and therapeutic interaction of CO₂.
Furthermore, WP2 underscores the use of carbogen (a gas mixture comprising approximately 5% CO2 and 95% O2) to augment cancer treatments. This blend is employed in numerous medical and scientific scenarios due to its unique properties that are advantageous for diagnosing and treating many conditions, as outlined in Table 4.
Regarding WP3, it is noteworthy to cite studies examining the tumor microenvironment, particularly those focusing on tumor metabolism (Benedetti et al., 2023; Luo and Liang, 2023; Micalet et al., 2023). These studies pay attention to the regulation of pH levels in tumor cells, including the distinct expression of ion transporters and proton pumps that may be more active or differently regulated in cancer cells compared to healthy ones (Qiao et al., 2017; Khalaf et al., 2021). Additional research shows that cancer cells’ intracellular pH (pHi) differ from normal cells. Typically, normal cells have a lower pHi than the extracellular pH (pHe), with pHi and pHe values ranging mainly from 7.0 to 7.2 and 7.3 to 7.4, respectively. Conversely, cancer cells tend to have an elevated pHi, between 7.12 and 7.65, and a reduced pHe range of 6.2–6.9, a reversal in intra-extracellular pH gradient indicative of cancerous tissue that promotes disease progression (Persi et al., 2018). This phenomenon, represented by extracellular acidosis and intracellular alkalinization, helps trigger cellular proliferation, evade apoptosis, initiate a metabolic shift to aerobic glycolysis (the Warburg effect), foster genetic instability, and potentially enhance multi-drug resistance within cancer cells. Increased pHi and decreased pHe can also augment invasion and metastasis. Hence, assessing the pHi in tumors could offer a promising way to monitor cancer progression and responses to treatment (Shirmanova et al., 2015). However, due to methodological limitations, there could be subject to vast variations (Table 5).
For WP3, it is also worth highlighting the Fundamental Viewpoint Points (FVPs) that were chosen based on predefined parameters related to the tumor microenvironment, as seen in Table 6.
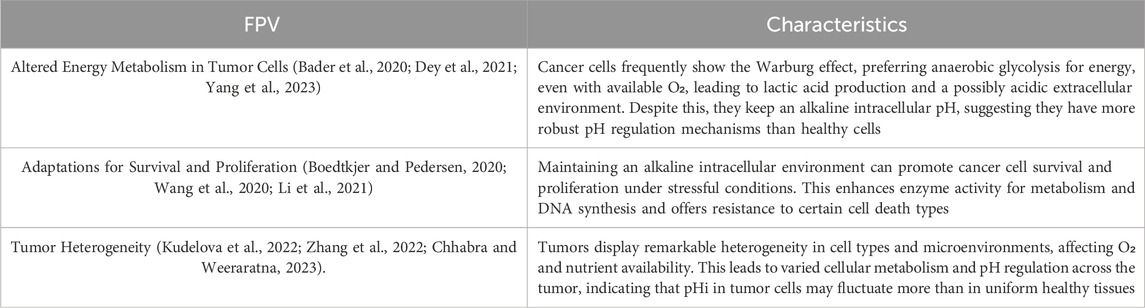
Table 6. Key viewpoints selected in physiology and cancer biology support possible discrepancies in intracellular pH between healthy and oncological cells.
With respect to WP4, Yew Wong and Aspinwall (2005) classified the CSFs due to their comparable approach to the FVPs. The CSFs, presented in Table 7, represent areas of significant integration between the tumor microenvironment and the biological impact of CO2/carbonic acid, which is derived from the integration process of the previously defined FVPs.
In WP5, we hypothesized how carbonic acid could facilitate intracellular O2 penetration by exploring fundamental physiology and cellular biochemistry concepts. This hypothesis derives from a complex system of adaptation in which hypercapnia, via increased circulating carbonic acid, could alter cellular function and O2 dynamics in diverse ways. These mechanisms may explain how extracellular environment manipulation could enhance O2 penetration into cells, providing a fresh perspective for therapies against cancer and other pathological conditions (Table 8). Further scrutinizing these mechanisms may reveal new research pathways and therapeutic targets.
The tumor microenvironment typically exhibits hypoxia and acidosis, attributes that foster cancer advancement, resistance to treatment, and immune suppression (Bożyk et al., 2022). Partial restoration of homeostasis can be achieved by manipulating the microenvironment’s pH through increased concentration of carbonic acid. This impacts tumor dynamics, improves tumor cell oxygenation, alters extracellular vesicle functionality, and enhances patient survival and quality of life. Improving oxygenation in tumor tissue indirectly could heighten cancer cell sensitivity to conventional therapies such as radiotherapy and chemotherapy, a phenomenon similar to carbogen use (as per Table 4). Therefore, anticipated outcomes from clinical exploration of the hormetic effects of CO2 on cancer include increased tumor oxygenation, increased tumor cell sensitivity to treatments, modulation of the tumor microenvironment, and development of combined treatment strategies. Guided by these insights, this relationship is depicted through the Fundamental Viewpoint Factor and their corresponding CSFs in Figure 3.
After the study, a SMART and FINER analysis was carried out in WP6 to assess the potential impact of this novel medical hypothesis. It was scrutinized against the goals of being specific, measurable, achievable, relevant to cancer research and having a defined timeline for accomplishment (Table 9).
Our findins support the understanding that there are connections between tumor microenvironment and carbon dioxide. The connection lies in the tumor microenvironment, which is characterized by a limited extracellular buffering capacity to handle lactic acid. The connection between this and carbon dioxide (CO2) involves several key points (Table 10).
Carbon dioxide (CO2) can play a role in regulating the acidic microenvironment of the tumor, though its effectiveness and impact are influenced by several factors, including genetic influences, intracellular pH changes, intracellular oxygen levels, and the activation of remaining mitochondria.
Production and Presence of Carbon Dioxide in Tumors. In typical cells, CO2 is produced as a byproduct of aerobic respiration in the mitochondria. However, tumor cells primarily rely on anaerobic glycolysis, which significantly reduces the production of CO2. This metabolic shift leads to a decrease in mitochondrial CO2 production, resulting in an altered role for CO2 in the tumor microenvironment compared to normal tissues. When the amount of CO2 is amplified in the tumor microenvironment through a protocol exposing the patient to an atmosphere of two%–5% CO2, the role of CO2 in regulating pH starts to take effect. This process occurs through the formation of carbonic acid (H2CO₃), which amplifies the buffering capacity. When CO2 in aqueous environments reacts with water, it forms H2CO₃, which then dissociates into bicarbonate (HCO₃⁻) and hydrogen ions (H⁺). This reaction is reversible and helps buffer changes in pH by either consuming or releasing H⁺, depending on the prevailing conditions. The presence of CO2 and its conversion to H2CO₃ provide a buffering system that can modulate the pH of the tumor microenvironment. This buffering action can help neutralize excess acids, such as lactic acid produced by glycolytic tumor cells, thereby mitigating extreme pH changes. Böning et al. (1991) documented that the influence on the Bohr effect caused by the release of lactic acid during physical exercise is entirely dependent on the buffering effect of CO2.
Influence of CO2 on Oxygen Levels and Mitochondrial Activation. The amplification of the Bohr effect in the region by buffering lactic acid, in turn, enhances an increase in oxygen levels and mitochondrial activation, indirectly influencing the presence of intracellular oxygen. Increasing the availability of CO2 and its subsequent conversion to bicarbonate can facilitate the transport and utilization of oxygen within tumor cells. Higher intracellular oxygen levels can shift the balance from glycolytic metabolism to more oxidative processes.
The activation of remaining mitochondria in tumor cells can also, in theory, be possible. These mitochondria can be activated in the presence of higher oxygen levels and appropriate genetic regulation. This can enhance oxidative phosphorylation and reduce reliance on glycolysis, potentially decreasing the production of lactic acid and altering the microenvironment. Of course, such activation is directly dependent on the quantity of remaining mitochondria in the tumor cell, which in turn is dependent on the genetic characteristics of each tumor type.
Genetic Influences and Cellular Responses. Certain genetic factors in tumor cells can influence the response to changes in intracellular oxygen levels. For example, nuclear genome-derived circular RNAs (circRNAs) like circPUM1 can localize in mitochondria and regulate oxidative phosphorylation. Gong et al. (2022) described that knockdown of circPUM1 results in lower intracellular oxygen concentration, downregulated oxidative phosphorylation, decreased mitochondrial membrane potential, increased ROS generation, and shrinking of mitochondria. This regulation is crucial for maintaining mitochondrial function and can be influenced by intracellular oxygen levels, indirectly influenced by the presence of CO2 in the extracellular environment.
Acid-Base Balance and the Bohr Effect. The presence of CO2 in the extracellular environment affects the acid-base balance through the formation of carbonic acid and bicarbonate, which can influence the pH. The Bohr effect, where CO2 and H⁺ reduce the affinity of hemoglobin for oxygen, facilitates oxygen release to tissues, indirectly affecting intracellular oxygen levels. However, this effect is more complex in tumor environments due to altered metabolic pathways.
The increase in intracellular CO2 can lead to the formation of H2CO₃ and subsequently HCO₃⁻ and H⁺, which can alter the intracellular pH. The tumor cells’ ability to manage these changes is critical for their survival and proliferation. Additionally, the weakly acidic microenvironment outside the cell can affect the intracellular pH by impacting the exchange and buffering systems within the cell.
Thus, it is possible to conclude that while carbon dioxide has the potential to regulate the acidic microenvironment of the tumor through its role in forming carbonic acid and buffering pH changes, its effectiveness is influenced by the metabolic and genetic characteristics of the tumor cells. The inherent metabolic shift in tumors towards glycolysis reduces CO2 production, limiting its direct impact. However, genetic factors and the potential to increase intracellular oxygen levels and activate remaining mitochondria suggest that CO2 can modulate the microenvironment to some extent. This regulation may help buffer the acidity and potentially alter the tumor cells’ metabolic dependencies, offering a nuanced approach to managing the tumor microenvironment.
4 Discussion
The insights gleaned from our results lay the foundation for a deeper discussion. We delve into how these findings correlate with and expand upon existing knowledge, driving forward the conversation on CO2's therapeutic frontier. The findings presented provide a nuanced view of CO2’s role within the tumor microenvironment. We now discuss these results in the context of existing literature and their potential to revolutionize oncological therapies, revisiting our initial hypotheses and expanding on the theoretical framework introduced earlier. Reflecting on the results, we delve into the potential of CO2 modulation within the tumor microenvironment as a groundbreaking approach to cancer therapy. This discussion builds upon our initial exploration of CO2's roles and their implications for treatment, as introduced, ensuring our conclusions directly stem from the foundational insights presented at the outset. In cancer research, manipulating CO2 shows promise in influencing the tumor microenvironment, as indicated by the 18 CSFs likely to be affected directly or indirectly. Modulating the tumor microenvironment’s pH via carbonic acid/carbon dioxide may significantly impact enzymatic activity, including heme oxygenase. This could affect tumor cell proliferation, survival, and migration, as well as the immune response. Adjusting the pH may improve immunosurveillance effectiveness and boost immunotherapy. Additionally, an acidic environment can promote angiogenesis and metastasis, implying that increasing the CO2 availability to alter pH may help limit tumor growth and dissemination. The production of CO2 in the TME can amplify these effects, modulating pH and promoting an environment that favors cell survival and tumor progression. Therapeutic strategies targeting pH regulation and HO-1 inhibition may offer promising approaches to disrupt these mechanisms and promote antitumor immune responses. Therefore, integrating knowledge about the hormetic potential of CO2 with HO-1 activity can provide new perspectives for the development of anticancer therapies.
Indirect evidence supporting the hypothesis that increasing CO2 in the tumor microenvironment could be a valid medical approach was identified in scientific literature. Liao et al. (2024) demonstrate that modifying the extracellular pH of the tumor microenvironment has an antitumor effect. They explain that the acidic tumor microenvironment is primarily caused by lactic acid production rather than CO2, due to abnormal mitochondrial activity in tumor cells. However, the role of pH regulators, such as monocarboxylate transporters (MCTs), carbonic anhydrase IX (CA IX), and Na+/H+ exchangers (NHE1), is critical in maintaining pH balance. These regulators can be overwhelmed by excessive acid production, which perpetuates an acidic environment. This suggests that manipulating these pH regulators, potentially through CO2 modulation, might help control acidification and, consequently, tumor invasion, migration, and drug resistance. The study by Ciccone et al. (2020) highlights that the inhibition of CA-IX induces antitumor effects more directly related to the regulation of biochemical and molecular processes within cancer cells. Recently, Cheng et al. (2024) stated that inhibitors of carbonic anhydrase IX (CA-IX) demonstrate inhibitory cancer activity in vitro. These studies provide laboratory data closely aligned with what this research aims to demonstrate, as the inhibition of CA-IX results in an increase of carbon dioxide in the tumor microenvironment as a major result. The findings from both studies indirectly underscore the potential therapeutic implications of manipulating CO2 levels in the tumor microenvironment, thereby supporting the broader hypothesis of harnessing the hormetic potential of CO2 for innovative cancer treatments, providing further impetus for research exploring this approach. De Palma et al. (2017) describe the tumor microenvironment as characterized by hypoxia, low pH, low glucose, and high ROS. They find that pH changes influence tumor angiogenesis and immune responses, linking the acidification of the TME to enhanced tumor progression. This implies that interventions aiming to regulate TME pH, possibly through CO2 modulation, could enhance anti-angiogenic and immunological responses, presenting new therapeutic strategies. Persi et al. (2018) highlight the importance of intracellular pH (pHi) in cancer metabolism, proposing that modulating pHi could be a novel therapeutic strategy. The presence of CO2 in the tumor microenvironment could influence intracellular oxygen influx, which in turn might contribute to rearranging pHi by stimulating existing mitochondrial activity and reducing lactic acid production. This complements strategies targeting extracellular pH and suggests that combining these approaches with CO2 modulation could enhance anti-cancer treatments, particularly for aggressive cancers, by inhibiting tumor cell proliferation and survival. Takano et al. (2023) document that CO2-induced extracellular pH decrease activates CREB and upregulates TGF-β1, influencing enzymatic activity involved in extracellular matrix production. This supports the idea that CO2 can modulate crucial signaling pathways in the TME. Furthermore, Niland et al. (2021) describe how matrix metalloproteinases (MMPs), particularly MMP-14, play a crucial role in tissue remodeling and cancer progression by degrading extracellular matrix components and influencing cell-matrix communication. Given that pH changes can affect MMP activity, the modulation of CO2 levels in the TME might indirectly impact the proteolytic activity of MMPs, providing additional support for the potential therapeutic role of CO2 in cancer treatment. With these findings in hand, in addition to modulating the tumor microenvironment’s pH, CO2 can potentially affect the activity of key enzymes, impacting various biological processes. Further, CO2 may influence activities such as autophagy, and pathways involving sirtuins and AMPK, which are essential for cellular stress response and energy homeostasis. By modulating these pathways, CO2 could affect cell survival and alter the tumor microenvironment, consequently impacting tumor growth and progression.
Whether this hypothesis can become a reality will depend on several critical factors, including funding availability, forming a multidisciplinary team with the required expertise, and gaining access to advanced research infrastructure. The inherent difficulties in translating preclinical findings to clinical applications due to disparities between experimental models and the complexity of human biological systems pose additional challenges. Despite the ambitious and promising nature of the medical hypothesis, its success hinges on thoughtful planning, ample resources, and collaboration among scientists, clinicians, and research institutions. Adapting the research plan based on interim results and newfound challenges will also be crucial for success (Chesbrough, 2003; Flessa and Huebner, 2021).
The controlled use of CO2, either through inhalation or other forms of administration, may be a viable strategy to modulate this microenvironment, augmenting conventional therapies like radiotherapy, chemotherapy, and immunotherapy. This highlights the importance of CO2 balance due to the central role of carbonic acid in regulating pH in body fluids. The enzyme carbonic anhydrase, which facilitates CO2’s reversible conversion to carbonic acid, is key in this process. Alterations in CO2 levels can affect the acid-base balance and the concentration of ROS (Garcia and Ramirez, 2017; Hoiland et al., 2019; Hall and Hall, 2020).
Having established the groundwork through our systematic analysis, we now turn to the implications of these findings, exposure to a CO2-enriched environment (3%–5%) could potentially affect cancer progression by modulating pathways. In September 2023, the AuBento Institute—Center for Teaching, Clinic, and Research in Orthomolecular and Integrative Practice, affiliated with the Federal University of Santa Maria, was inaugurated as a business model managed by the value-based healthcare tool to serve as a case study. Since its inauguration, the clinical practice has provided promising results that may encourage further exploration of this hypothesis. For instance, in three documented cases, integrative approaches, including the hormetic therapeutic potential protocol of carbon dioxide in oncological treatments, have been applied. The first case involves a 64-year-old patient with stage IV prostate cancer post-surgery, who experienced an increase in PSA levels (6.07) at the end of October. Approximately 6 months after the integrative protocol was applied, the PSA level dropped to 0.6, with no systemic signs of disease activity. Another patient, a 72-year-old with mantle cell lymphoma, had an estimated survival of 15 days according to local oncologists. After adhering to the integrative protocol, the patient has survived for more than 8 months, with no systemic signs of disease activity. A 48-year-old woman with a 1.1 cm nodule in the left upper quadrant of her breast, diagnosed with invasive ductal carcinoma in the right breast, started the integrative protocol. Upon review 45 days later, the nodule was no longer detected on bilateral breast MRI, with no systemic signs of disease activity. At no point are patients informed that their cancer is cured; rather, they are told that their biological terrain has adjusted and that an oncostatic effect is being achieved. Patients are advised to continuously maintain the integrative practices. The current aim of this research is to be a review article, supporting the presented hypothesis directly or indirectly with the available scientific literature. Therefore, delving into the details of these treatments that provide empirical data is beyond the scope of the current article. However, these clinical cases offer preliminary empirical support for the proposed hypotheses and suggest that further controlled studies and experimental designs are warranted to validate these findings comprehensively. Persistent research is needed to fully understand the therapeutic potential of CO2 in cancer treatment, challenging conventional paradigms and offering new paths for disease prevention and treatment. Nevertheless, no experimental model could validate this research through in vitro studies. This limitation is due to the difficulty of an in vitro model, such as a cell culture, to accurately replicate all the complex adaptive processes triggered by the human body in response to breathing a CO2-enriched atmosphere.
Building on the insights from our methodological approach, under WP5, we proposed a protocol used in our integrative approaches and suggested for clinical research that involves evaluating participants’ physiological adaptation to increased CO2 levels. Participants, chosen based on specific inclusion criteria, will be exposed to an environment enriched with a gradually increasing concentration of CO2 (starting at 1% and possibly reaching up to 5% based on individual tolerance). This exposure will occur for extended periods, ranging from 8 to 24 h daily, over a full week, to stimulate a standard adaptive response rather than an immediate one. Although CO2 plays crucial roles in human physiology, its regulation and the potential negative impacts associated with elevated CO2 levels must be carefully considered for a balanced understanding. Therefore, it is essential to consider the hormetic potential of carbon dioxide and the importance of gradual exposure to increasing concentrations as outlined in the suggested clinical protocol. Symptoms at exposures above 5% in the atmosphere can include confusion, lethargy, respiratory difficulty, and in severe cases, it can lead to coma and death. However, the initial reaction triggered when CO2 levels in the blood rise above normal can lead to respiratory acidosis. This condition occurs when CO2 accumulates and reacts with water in the blood to form carbonic acid (H2CO₃), which dissociates into bicarbonate (HCO₃⁻) and hydrogen ions (H⁺), resulting in a decrease in blood pH. Nevertheless, with prolonged exposure—understanding the high controlled exposure level of CO2 as a stress—the body tends to compensate, potentially bringing about the expected clinical benefits.
Besides all the data presented, the study aims to stimulate the exploration of the potential of stress-induced telomere signaling with hormetic effects, a concept originally highlighted by Jacome Burbano and Gilson (2021). In this case, the stress is predefined high levels of CO2. The body responds to continuous exposure to high levels of CO2 by attempting to restore homeostasis. This is because a CO2-rich environment triggers a stress response in the body that disrupts cellular, systemic, and organismal homeostasis. In the early stages, the increase in CO2 exposure and subsequently higher carbonic acid concentration may alter the body’s acid-base balance and possibly increase oxidative stress. Oxidative stress can accelerate telomere shortening and damage DNA. However, the body’s antioxidant systems, buffer systems, and DNA repair mechanisms can consistently restore homeostasis despite ongoing exposure to elevated CO2 levels. Building upon this understanding, this adaptation might require significant adjustments to preserve genomic stability. Our theory is that physiological adaptation to increased exposure to CO2 and carbonic acid could result in changes in the expression of genes associated with oxidative stress metabolism, DNA repair, and telomere maintenance. For instance, the activity of telomerase, a telomeric DNA-adding enzyme, might be modulated as a response to the altered environment, influencing DNA’s regenerative capacity. Therefore, long-term exposure to a high CO2 environment and the related increase in the body’s carbonic acid concentration might impact genomic stability, aging, and disease susceptibility. The body’s capability to adjust and control telomere maintenance and repair in response to these environmental challenges is the essence of CO2’s hormetic effects. This, ultimately, would be the scientific validation of statements such as Henderson’s (1940) claim, “CO2 is the primary parahormone of the whole body.
As suggested by our results, the proposed clinical protocol offers a novel approach to studying the impacts of long-term exposure to CO2-rich environments on human physiology. It specifically examines the hormetic potential of CO2 and possible therapeutic implications. However, logistical considerations such as creating a controlled environment for CO2 exposure, recruiting willing participants for a demanding regimen, as well as obtaining necessary approvals from a research ethics committee to ensure ethical and regulatory compliance are clear necessities. Our discussion underscores the significant implications of manipulating CO2 and carbonic acid for cancer therapy. Drawing upon these insights, we conclude by reflecting on the study’s contributions to the field and outlining avenues for future research. Our discussion elucidates the transformative potential of CO2 manipulation in oncology, setting the stage for concluding reflections on this journey from theoretical exploration to tangible therapeutic prospects.
5 Conclusion
In conclusion, drawing from the evidence gathered and analyzed, we ascertain that meticulous manipulation of CO2 and carbonic acid in the tumor microenvironment holds promising therapeutic potential. This completes our research journey, bridging the introductory premise with empirical findings and theoretical discussions. Building on this foundation, our medical hypothesis proposes an innovative strategy to significantly alter oncological treatment approaches by exploring the impact of CO2 and carbonic acid on the tumor microenvironment. Planned pilot clinical studies will further examine hypercapnia’s effects on cellular oxygenation and tumor environment dynamics. This critical initial phase aims to establish a robust understanding that can inform and guide future oncological therapies, potentially unveiling new treatment avenues through enhanced oxygen penetration into tumor cells by increased carbonic acid.
Transitioning this hypothesis into clinical application necessitates numerous careful steps involving thorough preclinical studies and the development of biomarkers to gauge intervention efficacy. It is also crucial to design clinical trials with defined inclusion and exclusion criteria and precise endpoints, bearing in mind the ethical implications of altering cancer metabolism and potential side effects.
Manipulating the tumor microenvironment using carbonic acid offers a novel perspective for improving cancer therapies and necessitates meticulous investigation. This groundbreaking approach in oncology underscores the value of interdisciplinary and collaborative methods, pledging transformative insights and considerable progress in the cancer fight. To conclude, the study of CO2 and carbonic acid’s role in the tumor environment presents a potentially groundbreaking approach in oncology, offering new and more effective strategies for tackling cancer. This concept, which carefully transitions from theory to clinical practice, promises to enhance the field of oncology by paving unparalleled paths for cancer treatment development, all while acknowledging current experimental models’ limitations in fully understanding physiological adaptations to continuous CO2-enriched atmosphere stressors.
Author contributions
LE: Conceptualization, Data curation, Formal Analysis, Investigation, Methodology, Validation, Writing–original draft, Writing–review and editing, LD: Conceptualization, Data curation, Formal Analysis, Supervision, Validation, Visualization, Writing–original draft. CR: Conceptualization, Formal Analysis, Investigation, Validation, Writing–review and editing, JM: Conceptualization, Validation, Writing–review and editing.
Funding
The author(s) declare that no financial support was received for the research, authorship, and/or publication of this article.
Conflict of interest
Author LE was employed by Elastro Crete.
The remaining authors declare that the research was conducted in the absence of any commercial or financial relationships that could be construed as a potential conflict of interest.
Publisher’s note
All claims expressed in this article are solely those of the authors and do not necessarily represent those of their affiliated organizations, or those of the publisher, the editors and the reviewers. Any product that may be evaluated in this article, or claim that may be made by its manufacturer, is not guaranteed or endorsed by the publisher.
References
Alishahi M., Kamali R. (2019). A novel molecular dynamics study of CO2 permeation through aquaporin-5. Eur. Phys. J. E Soft Matter 42 (11), 151. PMID: 31773315. doi:10.1140/epje/i2019-11912-x
Angeli A., Carta F., Nocentini A., Winum J. Y., Zalubovskis R., Akdemir A., et al. (2020). Carbonic anhydrase inhibitors targeting metabolism and tumor microenvironment. Metabolites 10 (10), 412. PMID: 33066524; PMCID: PMC7602163. doi:10.3390/metabo10100412
Bader J. E., Voss K., Rathmell J. C. (2020). Targeting metabolism to improve the tumor microenvironment for cancer immunotherapy. Mol. Cell 78 (6), 1019–1033. PMID: 32559423; PMCID: PMC7339967. doi:10.1016/j.molcel.2020.05.034
Bagherani N., Smoller B. R., Tavoosidana G., Ghanadan A., Wollina U., Lotti T. (2023). An overview of the role of carboxytherapy in dermatology. J. Cosmet. Dermatol 22 (9), 2399–2407. Epub 2023 Mar 31. PMID: 36999460. doi:10.1111/jocd.15741
Bana e Costa C. A., Ensslin L., Corrêa E. C., Vansnick J.-C. (1999). Decision support systems in action: integrated application in a multicriteria decision aid process. Eur. J. Operational Res. 113 (2), 315–335. doi:10.1016/s0377-2217(98)00219-7
Benedetti E., Liu E. M., Tang C., Kuo F., Buyukozkan M., Park T., et al. (2023). A multimodal atlas of tumour metabolism reveals the architecture of gene-metabolite covariation. Nat. Metab. 5 (6), 1029–1044. Epub 2023 Jun 19. PMID: 37337120; PMCID: PMC10290959. doi:10.1038/s42255-023-00817-8
Benner A., Patel A. K., Singh K., Dua A. (2024). “Physiology, Bohr effect,” in StatPearls (Treasure Island (FL): StatPearls Publishing). PMID: 30252284.
Bertholet A. M., Kirichok Y. (2022). Mitochondrial H+ leak and thermogenesis. Annu. Rev. Physiol. 84, 381–407. Epub 2021 Nov 10. PMID: 34758268; PMCID: PMC8976115. doi:10.1146/annurev-physiol-021119-034405
Boedtkjer E., Pedersen S. F. (2020). The acidic tumor microenvironment as a driver of cancer. Annu. Rev. Physiol. 82, 103–126. Epub 2019 Nov 15. PMID: 31730395. doi:10.1146/annurev-physiol-021119-034627
Bohr C., Hasselbalch K., Krogh A. (1904). On a biologically significant influence that the carbon dioxide tension of the blood exerts on its oxygen binding. Skand. Arch. Physiol. 16, 401–412. doi:10.1111/j.1748-1716.1904.tb01382.x
Böning D., Hollnagel C., Boecker A., Göke S. (1991). Bohr shift by lactic acid and the supply of O2 to skeletal muscle. Respir. Physiol. 85 (2), 231–243. PMID: 1947461. doi:10.1016/0034-5687(91)90064-p
Bożyk A., Wojas-Krawczyk K., Krawczyk P., Milanowski J. (2022). Tumor microenvironment-A short review of cellular and interaction diversity. Biol. (Basel). 11 (6), 929. PMID: 35741450; PMCID: PMC9220289. doi:10.3390/biology11060929
Buess H. J. (1953). Joh. Friedrich Miescher and the contribution of Basle physicians to the biology of the nineteenth century. Yale J. Biol. Med. 25 (4), 250–261. PMID: 13049881; PMCID: PMC2599305.
Cheng T., Wang N., Wen R., Wang S., Zhang H., Cheng M. (2024). Discovery of non-sulfonamide carbonic anhydrase IX inhibitors through structure-based virtual screening. Phys. Chem. Chem. Phys. 26 (11), 8767–8774. PMID: 38420672. doi:10.1039/d3cp05846h
Chesbrough H. (2003). Open innovation: the new imperative for creating and profiting from technology. Boston, MA: Harvard Business School Publishing.
Chhabra Y., Weeraratna A. T. (2023). Fibroblasts in cancer: unity in heterogeneity. Cell 186 (8), 1580–1609. PMID: 37059066. doi:10.1016/j.cell.2023.03.016
Chung C., Seo W., Silwal P., Jo E. K. (2020). Crosstalks between inflammasome and autophagy in cancer. J. Hematol. Oncol. 13 (1), 100. PMID: 32703253; PMCID: PMC7376907. doi:10.1186/s13045-020-00936-9
Ciccone V., Filippelli A., Angeli A., Supuran C. T., Morbidelli L. (2020). Pharmacological inhibition of CA-IX impairs tumor cell proliferation, migration and invasiveness. Int. J. Mol. Sci. 21 (8), 2983. PMID: 32340282; PMCID: PMC7215745. doi:10.3390/ijms21082983
Cummins E. P., Selfridge A. C., Sporn P. H., Sznajder J. I., Taylor C. T. (2014). Carbon dioxide-sensing in organisms and its implications for human disease. Cell Mol. Life Sci. 71 (5), 831–845. Epub 2013 Sep 18. PMID: 24045706; PMCID: PMC3945669. doi:10.1007/s00018-013-1470-6
Cummins E. P., Strowitzki M. J., Taylor C. T. (2020). Mechanisms and consequences of oxygen and carbon dioxide sensing in mammals. Physiol. Rev. 100 (1), 463–488. Epub 2019 Sep 20. PMID: 31539306. doi:10.1152/physrev.00003.2019
De Palma M., Biziato D., Petrova T. V. (2017). Microenvironmental regulation of tumour angiogenesis. Nat. Rev. Cancer 17 (8), 457–474. doi:10.1038/nrc.2017.51
Dey P., Kimmelman A. C., DePinho R. A. (2021). Metabolic codependencies in the tumor microenvironment. Cancer Discov. 11 (5), 1067–1081. Epub 2021 Jan 27. PMID: 33504580; PMCID: PMC8102306. doi:10.1158/2159-8290.CD-20-1211
Doyle J., Cooper J. S. (2024). “Physiology, carbon dioxide transport,” in StatPearls (Treasure Island (FL): StatPearls Publishing). Available at: https://www.ncbi.nlm.nih.gov/books/NBK532988/.
Drucker P. F. (1954). The Practice of Management, Nova Iorque, Harper and Row. Traduzido em português: Prática de Administração de Empresas, 2 volumes. Rio de Janeiro: Editora Fundo de Cultura, 1962.
Fan G. L., Deng F. A., Zhou X., Liu L. S., Huang J. Q., Zhang D. W., et al. (2021). Plasma membrane targeted photodynamic O2 economizer for hypoxic tumor therapy. Biomaterials 273, 120854. Epub 2021 Apr 26. PMID: 33932703. doi:10.1016/j.biomaterials.2021.120854
Flessa S., Huebner C. (2021). Innovations in health care-A conceptual framework. Int. J. Environ. Res. Public Health 18 (19), 10026. PMID: 34639328; PMCID: PMC8508443. doi:10.3390/ijerph181910026
Garcia A. J., Ramirez J. M. (2017). Keeping carbon dioxide in check. Elife 6, e27563. PMID: 28513432; PMCID: PMC5435460. doi:10.7554/eLife.27563
Gondaliya P., Sayyed A. A., Driscoll J., Patel K., Patel T. (2023). Extracellular vesicle RNA signaling in the liver tumor microenvironment. Cancer Lett. 558, 216089. Epub 2023 Feb 8. PMID: 36758739; PMCID: PMC9992346. doi:10.1016/j.canlet.2023.216089
Gong W., Xu J., Wang Y., Min Q., Chen X., Zhang W., et al. (2022). Nuclear genome-derived circular RNA circPUM1 localizes in mitochondria and regulates oxidative phosphorylation in esophageal squamous cell carcinoma. Signal Transduct. Target Ther. 7 (1), 40. PMID: 35153295; PMCID: PMC8841503. doi:10.1038/s41392-021-00865-0
Gonzalez-Avila G., Sommer B., García-Hernández A. A., Ramos C. (2020). Matrix metalloproteinases' role in tumor microenvironment. Adv. Exp. Med. Biol. 1245, 97–131. PMID: 32266655. doi:10.1007/978-3-030-40146-7_5
Hall J., Hall M. (2020). Guyton and Hall textbook of medical physiology (guyton physiology) 14th edition. 14th edition. Publisher: Elsevier.
Henderson Y. (1940). Carbon dioxide. Cyclopedia of medicine (vol. 3). Philadelphia, PA: F. A. Davis.
Hoiland R. L., Fisher J. A., Ainslie P. N. (2019). Regulation of the cerebral circulation by arterial carbon dioxide. Compr. Physiol. 9 (3), 1101–1154. PMID: 31187899. doi:10.1002/cphy.c180021
Holick M. F. (2022). The CO-vid D-lemma: a call for action. Nutrients 14 (5), 963. PMID: 35267938; PMCID: PMC8912765. doi:10.3390/nu14050963
Huang W., Liu Y., Luz A., Berrong M., Meyer J. N., Zou Y., et al. (2021). Calcium/calmodulin dependent protein kinase kinase 2 regulates the expansion of tumor-induced myeloid-derived suppressor cells. Front. Immunol. 12, 754083. PMID: 34712241; PMCID: PMC8546266. doi:10.3389/fimmu.2021.754083
Hughes M. P. (2024). The cellular zeta potential: cell electrophysiology beyond the membrane. Integr. Biol. (Camb). 16, zyae003. Erratum in: Integr Biol (Camb). 2024 Jan 23;16: PMID: 38291769. doi:10.1093/intbio/zyae003
Hulley S. B., Cummings S. R., Browner W. S., Grady D. G., Newman T. B. (2007). Designing clinical research. 3nd ed. Philadelphia: Lippincott Williams and Wilkins.
Jacome Burbano M. S., Gilson E. (2021). The power of stress: the telo-hormesis hypothesis. Cells 10 (5), 1156. PMID: 34064566; PMCID: PMC8151059. doi:10.3390/cells10051156
Jaworska A., Malek K. K. A. (2021). Intracellular pH - advantages and pitfalls of surface-enhanced Raman scattering and fluorescence microscopy - a review. A Rev. Spectrochimica Acta Part A Mol. Biomol. Spectrosc. 251, 119410. doi:10.1016/j.saa.2020.119410
Kang R., Zeh H., Lotze M., Tang D. (2020). The multifaceted effects of autophagy on the tumor microenvironment. Adv. Exp. Med. Biol. 1225, 99–114. PMID: 32030650. doi:10.1007/978-3-030-35727-6_7
Katsyuba E., Romani M., Hofer D., Auwerx J. (2020). NAD+ homeostasis in health and disease. Nat. Metab. 2 (1), 9–31. Epub 2020 Jan 20. PMID: 32694684. doi:10.1038/s42255-019-0161-5
Keerthana C. K., Rayginia T. P., Shifana S. C., Anto N. P., Kalimuthu K., Isakov N., et al. (2023). The role of AMPK in cancer metabolism and its impact on the immunomodulation of the tumor microenvironment. Front. Immunol. 14, 1114582. doi:10.3389/fimmu.2023.1114582
Khalaf K., Hana D., Chou J. T., Singh C., Mackiewicz A., Kaczmarek M. (2021). Aspects of the tumor microenvironment involved in immune resistance and drug resistance. Front. Immunol. 12, 656364. PMID: 34122412; PMCID: PMC8190405. doi:10.3389/fimmu.2021.656364
Khan K. S., Kunz R., Kleijnen J., Antes G. (2011). “Systematic reviews to support evidence-based medicine,” in How to review and apply findings of health care research. 2nd ed. Abington: RSM Press.
Krasniqi B., Thaçi S., Dërmaku-Sopjani M., Rifati-Nixha A., Abazi S., Sopjani M. (2020). Insight into the mechanisms underlying the tracheorelaxant properties of the sideritis raeseri extract. Evid. Based Complement. Altern. Med. 2020, 6510708. PMID: 32908564; PMCID: PMC7475741. doi:10.1155/2020/6510708
Kudelova E., Smolar M., Holubekova V., Hornakova A., Dvorska D., Lucansky V., et al. (2022). Genetic heterogeneity, tumor microenvironment and immunotherapy in triple-negative breast cancer. Int. J. Mol. Sci. 23 (23), 14937. PMID: 36499265; PMCID: PMC9735793. doi:10.3390/ijms232314937
Leavitt R. J., Almeida A., Grilj V., Montay-Gruel P., Godfroid C., Petit B., et al. (2024). Acute hypoxia does not alter tumor sensitivity to FLASH radiotherapy. Int. J. Radiat. Oncol. Biol. Phys. 20 (24), S0360–S3016. Epub ahead of print. PMID: 38387809. doi:10.1016/j.ijrobp.2024.02.015
Li X., Wang S., Mu W., Barry J., Han A., Carpenter R. L., et al. (2022). Reactive oxygen species reprogram macrophages to suppress antitumor immune response through the exosomal miR-155-5p/PD-L1 pathway. J. Exp. Clin. Cancer Res. 41 (1), 41. PMID: 35086548; PMCID: PMC8793215. doi:10.1186/s13046-022-02244-1
Li Y., Zhao L., Li X. F. (2021). Hypoxia and the tumor microenvironment. Technol. Cancer Res. Treat. 20, 15330338211036304. PMID: 34350796; PMCID: PMC8358492. doi:10.1177/15330338211036304
Liao S., Wu G., Xie Z., Lei X., Yang X., Huang S., et al. (2024). pH regulators and their inhibitors in tumor microenvironment. Eur. J. Med. Chem. 267, 116170. Epub 2024 Feb 1. PMID: 38308950. doi:10.1016/j.ejmech.2024.116170
Liu J., Wei Y., Wu Y., Li J., Sun J., Ren G., et al. (2021). ATP2C2 has potential to define tumor microenvironment in breast cancer. Front. Immunol. 12, 657950. PMID: 33936088; PMCID: PMC8079766. doi:10.3389/fimmu.2021.657950
Loiselle F., Casey J. (2010). Measurement of intracellular pH. Methods Mol. Biol. Clift.( N.J.) 637, 311–331. doi:10.1007/978-1-60761-700-6_17
Luo Y., Liang H. (2023). Single-cell dissection of tumor microenvironmental response and resistance to cancer therapy. Trends Genet. 39 (10), 758–772. Epub 2023 Aug 30. PMID: 37658004; PMCID: PMC10529478. doi:10.1016/j.tig.2023.07.005
Luu Hoang K. N., Anstee J. E., Arnold J. N. (2021). The diverse roles of heme oxygenase-1 in tumor progression. Front. Immunol. 12, 658315. PMID: 33868304; PMCID: PMC8044534. doi:10.3389/fimmu.2021.658315
Marongiu I., Spinelli E., Scotti E., Mazzucco A., Wang Y. M., Manesso L., et al. (2021). Addition of 5% CO2 to inspiratory gas prevents lung injury in an experimental model of pulmonary artery ligation. Am. J. Respir. Crit. Care Med. 204 (8), 933–942. PMID: 34252009; PMCID: PMC8534619. doi:10.1164/rccm.202101-0122OC
Menchikov L. G., Shestov A. A., Popov A. V. (2023). Warburg effect revisited: embodiment of classical biochemistry and organic chemistry. Current state and prospects. Biochem. (Mosc). 88 (Suppl. 1), S1–S20. PMID: 37069111. doi:10.1134/S0006297923140018
Mendivil-Alvarado H., Limon-Miro A. T., Carvajal-Millan E., Lizardi-Mendoza J., Mercado-Lara A., Coronado-Alvarado C. D., et al. (2023). Extracellular vesicles and their zeta potential as future markers associated with nutrition and molecular biomarkers in breast cancer. Int. J. Mol. Sci. 24 (7), 6810. PMID: 37047783; PMCID: PMC10094966. doi:10.3390/ijms24076810
Micalet A., Moeendarbary E., Cheema U. (2023). 3D in vitro models for investigating the role of stiffness in cancer invasion. ACS Biomater. Sci. Eng. 9 (7), 3729–3741. Epub 2021 Jun 3. PMID: 34081437; PMCID: PMC10336749. doi:10.1021/acsbiomaterials.0c01530
Moris J. M., Cardona A., Hinckley B., Mendez A., Blades A., Paidisetty V. K., et al. (2023). A framework of transient hypercapnia to achieve an increased cerebral blood flow induced by nasal breathing during aerobic exercise. Cereb. Circ. Cogn. Behav. 5, 100183. PMID: 37745894; PMCID: PMC10514094. doi:10.1016/j.cccb.2023.100183
Naik A., Jensen V., Bakketun C. B., Enger R., Hrabetova S., Hrabe J. (2023). BubbleDrive, a low-volume incubation chamber for acute brain slices. Sci. Rep. 13 (1), 20005. Erratum in: Sci Rep. 2024 Jan 23;14(1):1981. PMID: 37973847; PMCID: PMC10654715. doi:10.1038/s41598-023-45949-9
Nicholls D. G. (2021). Mitochondrial proton leaks and uncoupling proteins. Biochim. Biophys. Acta Bioenerg. 1862 (7), 148428. Epub 2021 Mar 31. PMID: 33798544. doi:10.1016/j.bbabio.2021.148428
Niland S., Riscanevo A. X., Eble J. A. (2021). Matrix metalloproteinases shape the tumor microenvironment in cancer progression. Int. J. Mol. Sci. 23 (1), 146. PMID: 35008569; PMCID: PMC8745566. doi:10.3390/ijms23010146
Palmer B. F., Clegg D. J. (2023). Respiratory acidosis and respiratory alkalosis: core curriculum 2023. Am. J. Kidney Dis. 82 (3), 347–359. doi:10.1053/j.ajkd.2023.02.004
Parayath N. N., Padmakumar S., Amiji M. M. (2020). Extracellular vesicle-mediated nucleic acid transfer and reprogramming in the tumor microenvironment. Cancer Lett. 482, 33–43. Epub 2020 Apr 11. PMID: 32289440. doi:10.1016/j.canlet.2020.04.009
Persi E., Duran-Frigola M., Damaghi M., Roush W. R., Aly P., Cleveland J. L., et al. (2018). Systems analysis of intracellular pH vulnerabilities for cancer therapy. Nat. Commun. 9, 2997. doi:10.1038/s41467-018-05261-x
Phelan D. E., Mota C., Strowitzki M. J., Shigemura M., Sznajder J. I., Crowe L., et al. (2023). Hypercapnia alters mitochondrial gene expression and acylcarnitine production in monocytes. Immunol. Cell Biol. 101 (6), 556–577. Epub 2023 Apr 28. PMID: 36967673; PMCID: PMC10330468. doi:10.1111/imcb.12642
Putnam R. W., Filosa J. A., Ritucci N. A. (2004). Cellular mechanisms involved in CO(2) and acid signaling in chemosensitive neurons. Am. J. Physiol. Cell Physiol. 287 (6), C1493–C1526. PMID: 15525685. doi:10.1152/ajpcell.00282.2004
Qi C., Lei L., Hu J., Wang G., Liu J., Ou S. (2022). Identification of a five-gene signature deriving from the vacuolar ATPase (V-ATPase) sub-classifies gliomas and decides prognoses and immune microenvironment alterations. Cell Cycle 21 (12), 1294–1315. Epub 2022 Mar 10. PMID: 35266851; PMCID: PMC9132400. doi:10.1080/15384101.2022.2049157
Qiao Z. Y., Zhao W. J., Gao Y. J., Cong Y., Zhao L., Hu Z., et al. (2017). Reconfigurable peptide nanotherapeutics at tumor microenvironmental pH. ACS Appl. Mater Interfaces 9 (36), 30426–30436. Epub 2017 Aug 31. PMID: 28828864. doi:10.1021/acsami.7b09033
Quade B. N., Parker M. D., Occhipinti R. (2021). The therapeutic importance of acid-base balance. Biochem. Pharmacol. 183, 114278. Epub 2020 Oct 9. PMID: 33039418; PMCID: PMC7544731. doi:10.1016/j.bcp.2020.114278
Quintana-Cabrera R., Scorrano L. (2023). Determinants and outcomes of mitochondrial dynamics. Mol. Cell 83 (6), 857–876. Epub 2023 Mar 7. PMID: 36889315. doi:10.1016/j.molcel.2023.02.012
Rivers R. J., Meininger C. J. (2023). The tissue response to hypoxia: how therapeutic carbon dioxide moves the response toward homeostasis and away from instability. Int. J. Mol. Sci 24 (6), 5181. PMID: 36982254; PMCID: PMC10048965. doi:10.3390/ijms24065181
Rose A. (1905). Carbonic acid in medicine/carbon dioxide in medicine. New York: Funk and Wagnalls Company. Available at: https://wellcomecollection.org/works/ja67xkw4
Salihi A., Al-Naqshabandi M. A., Khudhur Z. O., Housein Z., Hama H. A., Abdullah R. M., et al. (2022). Gasotransmitters in the tumor microenvironment: impacts on cancer chemotherapy (Review). Mol. Med. Rep. 26 (1), 233. Epub 2022 May 26. PMID: 35616143; PMCID: PMC9178674. doi:10.3892/mmr.2022.12749
Sarkar P., Chattopadhyay A. (2021). Insights into cellular signaling from membrane dynamics. Arch. Biochem. Biophys. 701, 108794. Epub 2021 Feb 9. PMID: 33571482. doi:10.1016/j.abb.2021.108794
Scharping N. E., Rivadeneira D. B., Menk A. V., Vignali P. D. A., Ford B. R., Rittenhouse N. L., et al. (2021). Mitochondrial stress induced by continuous stimulation under hypoxia rapidly drives T cell exhaustion. Nat. Immunol. 22 (2), 205–215. Epub 2021 Jan 4. PMID: 33398183; PMCID: PMC7971090. doi:10.1038/s41590-020-00834-9
Sekyonda Z., An R., Goreke U., Man Y., Monchamp K., Bode A., et al. (2024). Rapid measurement of hemoglobin-oxygen dissociation by leveraging Bohr effect and Soret band bathochromic shift. Analyst 149, 2561–2572. Epub ahead of print. PMID: 38501195. doi:10.1039/d3an02071a
Shariffi B., Lloyd I. N., Cessac M. E., Harper J. L., Limberg J. K. (2023). Reproducibility and diurnal variation in middle cerebral artery blood velocity in healthy humans. Exp. Physiol. 108 (5), 692–705. Epub 2023 Mar 23. PMID: 36951536; PMCID: PMC10148902. doi:10.1113/EP090873
Shirmanova M. V., Druzhkova I. N., Lukina M. M., Matlashov M. E., Belousov V. V., Snopova L. B., et al. (2015). Intracellular pH imaging in cancer cells in vitro and tumors in vivo using the new genetically encoded sensor SypHer2. Biochimica Biophysica Acta (BBA) - General Subj. 1850 (9), 1905–1911. ISSN 0304-4165. doi:10.1016/j.bbagen.2015.05.001
Stahl-Meyer J., Stahl-Meyer K., Jäättelä M. (2021). Control of mitosis, inflammation, and cell motility by limited leakage of lysosomes. Curr. Opin. Cell Biol. 71, 29–37. Epub 2021 Mar 6. PMID: 33684809. doi:10.1016/j.ceb.2021.02.003
Stetter C., Weidner F., Lilla N., Weiland J., Kunze E., Ernestus R. I., et al. (2021). Therapeutic hypercapnia for prevention of secondary ischemia after severe subarachnoid hemorrhage: physiological responses to continuous hypercapnia. Sci. Rep. 11 (1), 11715. PMID: 34083595; PMCID: PMC8175721. doi:10.1038/s41598-021-91007-7
Sur S., Lin Z., Li Y., Yasar S., Rosenberg P. B., Moghekar A., et al. (2024). CO2 cerebrovascular reactivity measured with CBF-MRI in older individuals: association with cognition, physical function, amyloid, and tau proteins. J. Cereb. Blood Flow. Metab. 15, 271678X241240582. doi:10.1177/0271678X241240582
Surh Y. J., Chung H. T., Na H. K., Dulak J., Stec D. E. (2020). Progress in heme oxygenase research. Arch. Biochem. Biophys. 685, 108321. Epub 2020 Feb 24. PMID: 32105660; PMCID: PMC7344117. doi:10.1016/j.abb.2020.108321
Takano K., Kasamatsu S., Aoki M., Takahashi Y. (2023). Carbon dioxide-induced decrease in extracellular pH enhances the production of extracellular matrix components by upregulating TGF-β1 expression via CREB activation in human dermal fibroblasts. Exp. Dermatol 32 (10), 1651–1662. Epub 2023 Jun 28. PMID: 37377319. doi:10.1111/exd.14867
Venkateswaran G., Dedhar S. (2020). Interplay of carbonic anhydrase IX with amino acid and acid/base transporters in the hypoxic tumor microenvironment. Front. Cell Dev. Biol. 8, 602668. PMID: 33240897; PMCID: PMC7680889. doi:10.3389/fcell.2020.602668
Wang J. X., Choi S. Y. C., Niu X., Kang N., Xue H., Killam J., et al. (2020). Lactic acid and an acidic tumor microenvironment suppress anticancer immunity. Int. J. Mol. Sci. 21 (21), 8363. PMID: 33171818; PMCID: PMC7664620. doi:10.3390/ijms21218363
Wang Y., Song F., Zhang X., Yang C. (2022). Mitochondrial-related transcriptome feature correlates with prognosis, vascular invasion, tumor microenvironment, and treatment response in hepatocellular carcinoma. Oxid. Med. Cell Longev. 2022, 1592905. PMID: 35535359; PMCID: PMC9078845. doi:10.1155/2022/1592905
Wu Q. J., Zhang T. N., Chen H. H., Yu X. F., Lv J. L., Liu Y. Y., et al. (2022). The sirtuin family in health and disease. Signal Transduct. Target Ther. 7 (1), 402. PMID: 36581622; PMCID: PMC9797940. doi:10.1038/s41392-022-01257-8
Xia C., Liu C., Ren S., Cai Y., Zhang Q., Xia C. (2023). Potassium channels, tumorigenesis and targeted drugs. Biomed. Pharmacother. 162, 114673. Epub 2023 Apr 7. PMID: 37031494. doi:10.1016/j.biopha.2023.114673
Xu Q., Liu X., Mohseni G., Hao X., Ren Y., Xu Y., et al. (2022). Mechanism research and treatment progress of NAD pathway related molecules in tumor immune microenvironment. Cancer Cell Int. 22 (1), 242. PMID: 35906622; PMCID: PMC9338646. doi:10.1186/s12935-022-02664-1
Yang K., Wang X., Song C., He Z., Wang R., Xu Y., et al. (2023). The role of lipid metabolic reprogramming in tumor microenvironment. Theranostics 13 (6), 1774–1808. PMID: 37064872; PMCID: PMC10091885. doi:10.7150/thno.82920
Yew Wong K., Aspinwall E. (2005). An empirical study of the important factors for knowledge-management adoption in the SME sector. J. Knowl. Manag. 9 (3), 64–82. doi:10.1108/13673270510602773
Zhan Q., Jeon J., Li Y., Huang Y., Xiong J., Wang Q., et al. (2022). CAMK2/CaMKII activates MLKL in short-term starvation to facilitate autophagic flux. Autophagy 18 (4), 726–744. Epub 2021 Jul 20. PMID: 34282994; PMCID: PMC9037428. doi:10.1080/15548627.2021.1954348
Zhang A., Miao K., Sun H., Deng C. X. (2022). Tumor heterogeneity reshapes the tumor microenvironment to influence drug resistance. Int. J. Biol. Sci. 18 (7), 3019–3033. PMID: 35541919; PMCID: PMC9066118. doi:10.7150/ijbs.72534
Zhang H., Shao X., Dehez F., Cai W., Chipot C. (2020a). Modulation of membrane permeability by carbon dioxide. J. Comput. Chem. 41 (5), 421–426. Epub 2019 Sep 3. PMID: 31479166. doi:10.1002/jcc.26063
Zhang J., Han J., Li L., Zhang Q., Feng Y., Jiang Y., et al. (2020b). Inwardly rectifying potassium channel 5.1: structure, function, and possible roles in diseases. Genes Dis. 8 (3), 272–278. PMID: 33997174; PMCID: PMC8093645. doi:10.1016/j.gendis.2020.03.006
Zhou L., Zhang Z., Nice E., Huang C., Zhang W., Tang Y. (2022). Circadian rhythms and cancers: the intrinsic links and therapeutic potentials. J. Hematol. Oncol. 15 (1), 21. PMID: 35246220; PMCID: PMC8896306. doi:10.1186/s13045-022-01238-y
Zhou X., Jiang M., Liu Z., Xu M., Chen N., Wu Z., et al. (2021). Na+/H+-Exchanger family as novel prognostic biomarkers in colorectal cancer. J. Oncol. 2021, 3241351. PMID: 34759967; PMCID: PMC8575632. doi:10.1155/2021/3241351
Keywords: carbon dioxide therapy, acidic microenvironment modulation, oxygenation in cancer cells, hypercapnia in cancer treatment, pH regulation in tumor cells, immunomodulation in oncology, hormesis in cancer therapy, CO2-induced adaptive responses
Citation: Gaspary JFP, Edgar L, Lopes LFD, Rosa CB and Siluk JCM (2024) Translational insights into the hormetic potential of carbon dioxide: from physiological mechanisms to innovative adjunct therapeutic potential for cancer. Front. Physiol. 15:1415037. doi: 10.3389/fphys.2024.1415037
Received: 09 April 2024; Accepted: 18 June 2024;
Published: 17 July 2024.
Edited by:
Ernesto Alejandro Aiello, National University of La Plata, ArgentinaReviewed by:
Yusheng Lu, Minjiang University, ChinaAlejandro Orlowski, Universidad Nacional de La Plata, Argentina
Copyright © 2024 Gaspary, Edgar, Lopes, Rosa and Siluk. This is an open-access article distributed under the terms of the Creative Commons Attribution License (CC BY). The use, distribution or reproduction in other forums is permitted, provided the original author(s) and the copyright owner(s) are credited and that the original publication in this journal is cited, in accordance with accepted academic practice. No use, distribution or reproduction is permitted which does not comply with these terms.
*Correspondence: Luis Felipe Dias Lopes, bGZsb3BlczY3QHlhaG9vLmNvbS5icg==