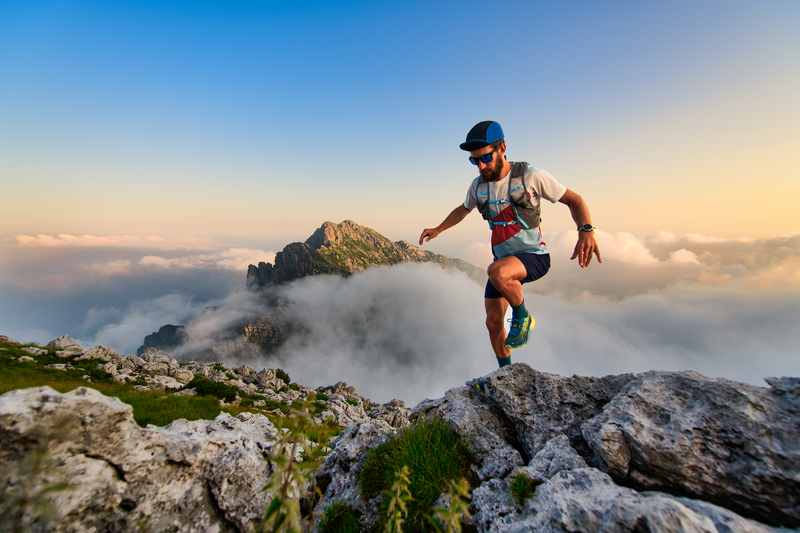
95% of researchers rate our articles as excellent or good
Learn more about the work of our research integrity team to safeguard the quality of each article we publish.
Find out more
ORIGINAL RESEARCH article
Front. Physiol. , 20 June 2024
Sec. Aquatic Physiology
Volume 15 - 2024 | https://doi.org/10.3389/fphys.2024.1410660
Somatic growth in vertebrates is mainly controlled by the growth hormone (GH)/insulin-like growth factor I (IGF-I) axis. The role of epigenetic mechanisms in regulating this axis in fish is far from being understood. This work aimed to optimize and evaluate the use of short-term culture of pituitary and liver explants from a farmed fish, the gilthead seabream Sparus aurata, for studying epigenetic mechanisms involved in GH/IGF-I axis regulation. Our results on viability, structure, proliferation, and functionality of explants support their use in short-term assays. Pituitary explants showed no variation in gh expression after exposure to the DNA methylation inhibitor decitabine (5-Aza-2′-deoxycytidine; DAC), despite responding to DAC by changing dnmt3bb and tet1 expression, and TET activity, producing an increase in overall DNA hydroxymethylation. Conversely, in liver explants, DAC had no effects on dnmts and tets expression or activity, but modified the expression of genes from the GH-IGF-I axis. In particular, the expression of igfbp2a was increased and that of igfbp4, ghri and ghrii was decreased by DAC as well as by genistein, which is suggestive of impaired growth. While incubation of liver explants with S-adenosylmethionine (SAM) produced no clear effects, it is proposed that nutrients must ensure the methylation milieu within the liver in the fish to sustain proper growth, which need further in vivo verification. Pituitary and liver explants from S. aurata can be further used as described herein for the screening of inhibitors or activators of epigenetic regulators, as well as for assessing epigenetic mechanisms behind GH-IGF-I variation in farmed fish.
Growth rate in farmed fish is among the phenotypic traits of major relevance for the aquaculture industry. Therefore, several strategies have been followed to increase fish growth rate such as genetic selection (Janssen et al., 2018) and improvements in diet formulation and feeding practices (Glencross et al., 2023). Somatic growth in fish, as in other vertebrates, is mainly controlled by the growth hormone (GH) - insulin-like growth factor I (IGF-I) axis. The molecular and physiological features of this axis in fish have been thoroughly studied and reviewed (Pérez-Sánchez et al., 2018; Bertucci et al., 2019; Triantaphyllopoulos et al., 2020; Ndandala et al., 2022). Briefly, GH is secreted by the pituitary under the control of the hypothalamus, and exerts systemic functions in metabolism, reproduction, physical activity, immunity and osmotic regulation (Vélez and Unniappan, 2021). Regarding growth, the main target for GH action is the liver, where it is recognized by GH receptors and induces the expression and release of IGF-I, which ultimately promote somatic growth. Indeed, circulating IGF-I stands as the most reliable biomarker of growth in fish (Pérez-Sánchez et al., 2018). However, secretion, half live, and actions of IGF-I are dependent on a suit of IGF binding proteins (IGFBPs) (Garcia de la Serrana and Macqueen, 2018). While some paralogs of IGFBPs are more clearly related with growth inhibition in fish, the understanding of the functions of these genes remains fragmented across fish (Garcia de la Serrana and Macqueen, 2018).
Main environmental factors regulating this system are those influencing the nutritional status (Pérez-Sánchez et al., 2018; Bertucci et al., 2019). Given that environmental factors modulate the activity of GH-IGF-I axis in fish, it is plausible that epigenetic mechanisms are involved, as epigenetic modifications serve as links between the environment and the genome to produce adaptive responses. Indeed, it is known that the P2 promoter of IGF-I is epigenetically regulated by DNA methylation in humans, with consequences in circulating IGF-I and growth (Ouni et al., 2016) and that DNA methylation also affects the activity of growth hormone response elements (GHREs) modulating IGF-I expression (Fu et al., 2015). However, the role of epigenetics in regulating GH-IGF-I axis in fish is far from being understood. GH putative promoter methylation in pituitary was observed in females of Nile tilapia compared with males, and it is negatively correlated with mRNA of GH and growth rate (Zhong et al., 2014). In liver, DNA methylation differences in igf2bp2 are linked to differences in growth in Nile tilapia (Podgorniak et al., 2019) while hydroxymethylation was found in other growth-related genes such as igfbp2 in this fish species (Konstantinidis et al., 2021). Also, changes in igf1 gene expression during development are negatively corelated with DNA methylation at promoter and first exon in Japanese flounder (Paralichthys olivaceus) (Huang et al., 2018). In the smooth tongue sole (Cynoglossus semilaevis), exons in the igf1 gene had different methylation levels in fish under osmotic stress compared to controls (Li et al., 2017). Results, in addition to sparse, are poorly consistent among fish species.
To further advance the understanding of the epigenetic regulation of the GH-IGF-I axis in farmed fish, and to evaluate environmental manipulations with putative positive effects on growth and other traits, suited experimental models are needed. While studies in live fish are unique to ultimately evaluate growth rate and other physiological responses, in vitro models are suited to screening and mechanistic studies. Different cell culture approaches have been used in epigenetic studies, but lack of similarity between the DNA methylation profiles of cultured cell lines and in vivo tissues have been reported (Antequera et al., 1990; Nestor et al., 2015). Conversely, it is known that models that better reproduce the architecture of the original tissue produce more realistic responses (Pampaloni et al., 2007), probably because these models enable the cell-cell interactions that occur in the animal. While these models have been developed such as spheroids, organoids, and precision cut liver slices (PCLS), the use of explants is still convenient because they are cheaper and can be implemented in any laboratory having only general lab equipment. Successful short-term explant culture of fish pituitaries (Helms et al., 1987; Astola et al., 2004; Cánepa et al., 2008; Fuentes et al., 2010) and liver (Yu et al., 2008; Beitel et al., 2015; Doering et al., 2015; Eisner et al., 2016; LeClair, 2021) have been reported.
This work aimed to optimize and evaluate the use of short-term culture of pituitary and liver explants from a farmed fish, the gilthead seabream Sparus aurata, for studying epigenetic mechanisms and GH/IGF-I axis regulation. We showed that pituitary and liver explants from S. aurata can be further used as described herein for the screening of inhibitors or activators of epigenetic regulators, as well as for assessing epigenetic mechanisms behind GH-IGF-I variation in farmed fish.
Gilthead seabream (S. aurata) fish (body weight 37.01 ± 0.83 g; mean ± sem) were obtained from University of Cadiz, and maintained at Institute for Marine Science of Andalusia (ICMAN-CSIC) facilities in aerated 120 L tanks in an open system under natural conditions of photoperiod and temperature (19–22oC), salinity of 34 ppt, oxygen level above 90%, pH 8.0 ± 0.1, and NH4 < 0.25 mg L−1. Animals were fed by hand to satiety twice a day with a commercial diet (Skretting, Burgos, Spain). All procedures complied with the Guidelines of the Spanish (RD53/2013) and European Union Council (2010/63/EU) for the use and experimentation of laboratory animals, and were reviewed and approved by the Spanish National Research Council (CSIC) Bioethical Committee and that of the Autonomous Andalusian Government (reference number 24/10/2023/092).
Fish were euthanized with overdose of 2-phenoxyethanol (Sigma-Aldrich, Merck, Germany) and decapitated. Pituitaries were collected and led stand for 1 h in 80% (v/v) Leibovitz L15 medium (L-15), pH 7.4, 10% fetal bovine serum (FBS), 10 mM HEPES, 100 IU/mL penicillin, 100 μg/mL of streptomycin, 2,5 ug/mL amphotericin B, 5.5 mM D(+)-glucose, 380 mOsm adjusted with NaCl, and filtered (before antibiotics addition) through Millipore 0.2 µm, henceforth referred to as culture media. Thereafter, glands were randomly distributed in a 96-wells microplate with one gland per well containing 100 µL of culture media. Five glands were taken from fish and immediately stored at −80oC as an initial sample. Pituitaries were cultured for 2 days at 23oC under 95% O2 and 5% CO2, with gently agitation. Culture media was removed after 24 h, stored at −80°C, and replaced by fresh media. At 24 and 48 h, five pituitaries were frozen at −80oC for biochemical assays and five glands stored in RNAlater for DNA and RNA extraction.
For liver explants, livers were placed on iced sterile petri dishes, and diced by hand initially into approximately 3–4 mm3 cubes. These explants were used to evaluate, during 48 h, the effect of the number of washing steps (two or five; every 10 min) and agitation during incubation in the viability of the explant. Later, after 5 washes, the effect of different volume of media (100 μL, 500 μL, 1 mL) and size of explant (1–2 mm3 or 3–4 mm3) were evaluated under gently and continuous agitation. A single 3–4 mm3 explant was placed in each well, while for 1–2 mm3 explants three explants were used per well. Sterile 96-well plates were used for 100 µL incubations and 24-wells plates for all other incubations. Next, the effect of temperature (ambient or 4oC) during washing steps of tissue was assessed in 1–2 mm3 explants washed five times and cultured in 1 mL of media under constant agitation. In all cases, five explants were taken from fish and immediately stored at −80oC as an initial sample. Finally, liver explants were cultured under the best conditions determined as above for 48 h at 23oC under 95% O2 and 5% CO2. Culture media was removed at 24 h, stored at −80°C, and replaced by fresh media. At 24 and 48 h, five liver explants were frozen at −80oC for biochemical assays and five explants stored in RNAlater for gene expression analyses.
Lactate dehydrogenase (LDH) activity was used to assess tissue integrity and measured by the LDH Activity Assay Kit (Sigma-Aldrich, St. Louis, MO, USA) in both explants and culture media. Catalase activity (CAT) was measured in post-mitochondrial supernatant (PMS) of explants as an indicator of oxidative stress during culture, by measuring the decomposition of H2O2 (Clairborne, 1985). Briefly, samples were homogenized in 50 μL of ultra-pure water, diluted (1:1) in K-phosphate buffer (0.2 M, pH 7.4) and centrifuged for 10 min at 10,000 g (4°C). The PMS was kept in −80°C until analysis. The enzymatic reaction was performed in 96-well microplates by the addition of 10 μL of the PMS to 140 μL of K-phosphate buffer (0.05 M, pH 7.0) and 150 μL of 30% H2O2. The decomposition of H2O2 was kinetically followed at 240 nm. To ensure that low level of CAT activity can be taken as indicative of low level of oxidative stress, explants were exposed to 100 µM of H2O2 (Ransy et al., 2020) for 15 min, and then collected and examined for CAT activity. GH and IGF-I secreted into the media by pituitaries and liver explants, respectively, were measured by the Fish GH and Fish IGF-I ELISA kits (CUSABIO, Wuhan, China). These ELISAs have been used before in different salmonids and non-salmonid fish, including the gilthead seabream (Amri et al., 2020; Rodríguez-Viera et al., 2022). All assays were performed in a microplate reader BioTek ELx808 (BioTek Instrument, Inc), except for catalase activity that was measured at 240 nm in a Synergy H1 microplate reader (Biotek).
Two experiments were performed as above and five explants of each tissue were collected at 0 h (native tissue) and 48 h in 4% formalin buffered to pH 7.0 with 0.1 M phosphate buffer for 24 h. Afterwards, samples were embedded in paraffin following a standard protocol to obtain 5–6 μm histological serial sections (Ortiz-Delgado et al., 2008). The Hematoxylin-Eosin technique (Luna, 1968) was used on deparaffinized slides to evaluate potential morphological differences among the treatment groups. The occurrence of cell proliferation was assessed by immunohistochemistry (PCNA staining) as an additional indicator of tissue functionality. A monoclonal antibody (PC10, Santa Cruz Biotechnology Inc., Heidelberg, Germany) against proliferating cell nuclear antigen (anti-PCNA) was utilized for this purpose, according to Piñuela et al. (2004).
S-adenosylmethionine (SAM) is the main donor of methyl groups for DNA methylation, genistein (GEN) is a methyltransferase inhibitor, and decitabine (5-Aza-2′-deoxycytidine; DAC) is a DNA demethylation agent. For expression of GH-IGF-I related genes, explants were processed as above and exposed during 24 h to three concentrations of SAM (100, 200, 300 µM), GEN (5, 10, 20 µM), and DAC (5, 15, 20 µM) for livers, while pituitaries to two concentrations of SAM (200, 300 µM), GEN (10, 20 µM), and DAC (15, 20 µM). In all cases, control explants were exposed to 0.2% of DMSO. Tissues were collected in RNAlater for gene expression analyses.
DNA and RNA were simultaneously extracted from each sample using the NucleoSpin TriPrep Kit (Macherey-Nagel, Düren, Germany), according to the manufacturer’s protocol. RNA concentration was measured with the Qubit 2.0 Fluorometer (Invitrogen, Life Technologies), and its quality assessed in an Agilent 2,100 Bioanalyzer (Agilent Technologies, Santa Clara, CA, USA) using RNA 6000 Nano Kits. Only samples with an RNA Integrity Number (RIN) higher than 8.5 were used. Total RNA (500 ng from liver and 10 ng from pituitaries) was reverse-transcribed using the qScript DNA synthesis kit (Quanta BioSciences). Gene expression was assessed by real-time qPCR for genes involved in the GH-IGF-I axis (Supplementary Table S1). All primers were synthesized by IDT (Integrated DNA Technologies, Leuven, Belgium). While these primer pairs were used previously (Supplementary Table S1, and references therein), assay linearity and amplification efficiency were corroborated under our conditions to be in all cases above 95% except for rpl27a (90%), by making 10-fold dilution curves of template starting from 10 ng. For qPCR, each 10 μL reaction contained 0.5 μL of 200 nM of each primer, 5 μL of PerfeCTa SYBR Green FastMix (Quanta Biosciences) and 4 μL of template (10 ng cDNA for liver and 1 ng for pituitaries). Control reactions with water and RNA instead of cDNA were included. qPCR was performed in a CFX Connect (BioRad) and cycling conditions for all genes were 95 °C, 10 min; [95°C, 15 s; 60°C, 30s] x 40 cycles; melting curve from 65°C to 95°C, with increments of 0.5°C, except for igfbp2b and igfbp4 for which 62°C and 58°C were used for annealing, respectively. Melting curves confirmed in all cases a single amplicon and the absence of primer-dimer artifacts. Relative gene expression was calculated by means of the 2−ΔΔCT method (Livak and Schmittgen, 2001). Six genes were evaluated as housekeeping genes (ef1a,18S, rps18, gapdh2, rpl27a, actb) all showing high stability among treatments according to NormFinder v20 (Andersen et al., 2004). The two most stable genes in each tissue (ef1a and rps18 in pituitary; ef1a and rpl27a in liver) were chosen for normalization.
The gene expression of DNA methylation remodeling genes was performed as detailed above for GH-IGF-I axis related genes, in tissue explants exposed to 15 µM DAC. For these genes, new primers (Supplementary Table S1) were designed in Primer 3 so that they span one exon-exon junction in target mRNAs. These genes showed to be expressed at low levels and thus, six points of 1/5 serial dilutions (dnmt1, dnmt3a, dnmt3l, tet1, tet2, tet3) or 1/2 serial dilutions (dnmt3bb, dmap1) starting from 10 ng of cDNA were used to check for assay linearity (R2) and amplification efficiency (E). In all cases efficiencies were above 98% except for dmap1 which remained with 71%. Cycling conditions for all these genes were 95 °C, 10 min; [95°C, 15 s; 60°C, 30s] x 40 cycles; melting curve from 65°C to 95°C, with increments of 0.5°C.
DNA methylation and hydroxymethylation were assessed using the MethylFlash Global DNA Methylation (5-mC) ELISA Easy Kit and the MethylFlash Global DNA Hydroxymethylation (5-hmC) ELISA Easy Kit (both from Epigentek, Farmingdale, New York), respectively, in tissue explants exposed to 15 µM DAC. For enzyme activity, nuclear proteins were extracted with the EpiQuik Nuclear Extraction Kit (Epigentek), and activities of DNMT and TET enzymes were measured with the EpiQuik DNMT Activity/Inhibition ELISA Easy Kit and the Epigenase 5 mC-Hydroxylase TET Activity/Inhibition Assay Kit (both from Epigentek), respectively. In all cases, the colorimetric variants of kits were used according to the manufacturer protocols.
All data were checked for normality and homogeneity of variance using Kolmogorov-Smirnov and Levene’s tests, respectively, with p < 0.05. LDH data during optimization of explant culture and gene expression after exposure to different concentrations of SAM, GEN and DAC were analyzed by two-way ANOVA, while CAT activity was analyzed by one-way ANOVA. When data were compared with initial values the Dunnett’s test was employed. Differences among means were detected by the Tukey’s test (p < 0.05). All other variables (paired data) were compared by t-test (p < 0.05). The software package GraphPad Prism 9.0 (GraphPad Software, Inc., San Diego, California, US) was used for all tests performed.
We first evaluated the LDH activity as a tissue integrity indicator under our assay conditions by inducing damage in explants with a non-ionic surfactant. A 24 h exposure of pituitary (Figure 1A) and liver (Figure 2A) explants to 0.2% Triton X-100 reduced LDH activity in the tissues and increased it in the media, indicating that LDH release is a sensitive indicator of tissue damage in the explants. Also, to ensure that CAT activity can be used as a reliable indicator of oxidative stress under our conditions, both tissues were exposed to H2O2 and were able to respond increasing its catalase activity (Figure 1C; Figure 3A for pituitary and liver, respectively).
Figure 1. Pituitary explants culture optimization and functionality. (A) Lactate dehydrogenase (LDH) activity in pituitary (yellow) and culture media (pink) as suitable indicator of tissue integrity responding to induced damage by 0.2% Triton X-100. (B) LDH activity showing pituitary integrity for 48 h in culture. (C) Catalase (CAT) activity in pituitary as suitable indicator of oxidative stress responding to induced oxidative stress by H2O2 and showing low level of oxidative stress in culture. (D) Growth hormone (GH) secretion by pituitaries in culture.
Figure 2. Liver explants culture optimization. (A) Lactate dehydrogenase (LDH) activity in liver explants (red) and culture media (pink) as suitable indicator of tissue integrity responding to induced damage by 0.2% Triton X-100. (B) LDH activity in liver explants showing the effects on tissue integrity of number of previous washing steps (two and five) and agitation (static and dynamic) during 48 h in culture. (C) LDH activity in liver explants showing the effects on tissue integrity of volume of culture media (100 μL, 500 μL, 1 mL) and size of explant (1–2 mm3 and 3–4 mm3) during 48 h in culture. (D) LDH activity in liver explants showing the effects on tissue integrity of temperature (ambient, 4oC) during tissue processing prior culture for 48 h.
Figure 3. Liver explants functionality. (A) Catalase (CAT) activity in liver explants as suitable indicator of oxidative stress responding to induced oxidative stress by H2O2 and showing low level of oxidative stress in culture. (B) Insulin-like growth factor-I (IGF-I) secretion by liver explants in culture.
Conversely, in untreated pituitaries, LDH in the media did not increase, glands retained its LDH content (Figure 1B), and maintained the same CAT activity (Figure 1C) and continued secreting GH into the media (Figure 1D) during 48 h. On the other hand, initial attempts to maintain liver explant failed, with more than 50% of LDH recovered in the media after 24 h. Thus, we went through a series of optimization steps, and we followed the progression by means of tissue LDH as recommended for tissues that do not growth in culture and may exhibit spontaneous release of the enzyme (Cox et al., 2021). This approach also allowed comparisons between explants and liver samples taken directly from the animals. LDH retained in the tissue was higher under agitation after 24 h (Figure 2B). The effect of washing septs was more evident at 48 h, with liver explants washed five times retaining the highest LDH activity (Figure 2B), although in all cases it was lower than in the original tissue (Figure 2B). Further improvement in tissue integrity was achieved by decreasing the size of liver explants down to 1–2 mm3 and increasing the volume of media to 1 mL (Figure 2C). Finally, by processing the tissue at 4oC, the content of LDH in the explants was as in the original tissue after 48 h of culture (Figure 2D). Under these conditions, liver explants showed no signs of oxidative stress (Figure 3A), and kept stable the secretion of IGF-I into the media (Figure 3B).
Histological evaluation of hepatic and pituitary explants revealed that they also maintained their normal architecture and cellular organization during the 48-h culture period. Figure 4 displays representative images of the explants from all the groups. Overall, the hepatic tissue maintained its structural integrity and cellular characteristics during the 48-h culture period (Figures 4A–D). At time 0, the hepatic tissue sections exhibited a normal parenchymal architecture. The hepatocytes appeared polygonal in shape and were arranged in cords, with a thickness of 2 cells, situated among the sinusoids. Interestingly, pancreatic acinar cells were also observed within the hepatic tissue. After 48 h of culture, the parenchymal arrangement of the liver remained preserved. The hepatocytes maintained their roundish polygonal cell body, and their nuclei were clearly visible, typically containing one nucleolus. However, in the exocrine pancreas, there was a discrete disorganization of the pancreocytes, although they still retained their zymogen granules (Figure 4A–D). Likewise, the histological evaluation of the pituitary tissue showed no significant changes or alterations in its structure during the 48-h culture period. At time 0, the pituitary tissue exhibited two main regions: the adenohypophysis composed of glandular tissue and the neurohypophysis composed of nervous tissue containing nerve fibers from the hypothalamus. After 48 h of culture, the pituitary tissue maintained its standard structural characteristics. Both the adenohypophysis and neurohypophysis remained intact, and the cell types present in these regions retained their characteristic features (Figure 4E–J).
Figure 4. Representative images of the histological analysis of native tissue (directly from fish) and 48 h cultured explants. (A,B) Liver native explants. (C,D) Liver explants at 48 h. (E, F) Pituitary native explants. (G–J) Pituitary explants at 48 h. Staining: (A, C, E, G, and I), Haematoxylin and Eosin; (B,D,F,H,J), PCNA immunostaining. Scale bar = 50 µm.
We utilized Proliferating cell nuclear antigen (PCNA) immunostaining to investigate the presence of actively dividing cells in the hepatic and pituitary explants. PCNA is a protein that plays a crucial role in DNA replication and repair, and its expression is indicative of cells in the active phase of the cell cycle, specifically during DNA synthesis (S phase). After 48 h of culture, positive PCNA cells were detected in both the hepatic and pituitary explants, indicating that DNA synthesis and cell proliferation were still occurring at this time point. The presence of positive PCNA cells after 48 h of culture indicates that the explanted hepatic and pituitary tissues remained viable and capable of cellular division during the experimental period (Figure 4B,D,F,H,I).
The exposure of pituitaries to the assayed concentrations of SAM, GEN and DAC produced no changes in gh gene expression (Supplementary Table S2).
The exposure of liver explants to SAM for 24 h did not produce variations in the expression of the genes studied regardless of the SAM concentration used (Supplementary Table S2). Likewise, the exposure of liver explants to DAC, at the concentrations tested, did not alter the expression of igf1, igfbp1a, igfbp1b, and igfbp2b (Supplementary Table S2). However, DAC produced a significant increase in the expression of igfpb2a, and a significant decrease in the expression of igfpb4, ghri, and ghrii, respect to SAM treated explants and control livers taken directly from the animal (Table 1; Figure 5). These effects were consistently observed irrespective of the DAC concentration (Figure 5). The effects of GEN were, in general, similar to those of DAC (Figure 5). No interaction was found between the DNA methylation remodeling agents evaluated (SAM, GEN, DAC) and their concentration for the affected (Table 1) and unaffected (Supplementary Table S2) genes.
Table 1. Statistics for gene expression in liver explants after 24 h exposure to DNA methylation remodeling agents.
Figure 5. Effects of DNA methylation remodeling factors [S-adenosylmethionine (SAM), genistein (GEN) and 5-Aza-2′-deoxycytidine (DAC)] on the expression of growth-related genes in liver explants. (A) igfbp2a, (B) igfbp4, (C) ghri, (D) ghrii. Concentrations denoted as 1, 2 and 3 because different for each substance: SAM (100, 200, 300 µM), GEN (5, 10, 20 µM), and DAC (5, 15, 20 µM). Treatments compared with Two-way ANOVA (p < 0.05) followed by the Turkey test, and all treatments against the control with one-way ANOVA (p < 0.05) followed by a Dunnett multiple comparison test. *p < 0.05, **p < 0.01.
The expression of different genes related with DNA methylation and de-methylation was examined after 24 h exposure of explants to 15 µM DAC. We observed a significant increase in the expression of dnmt3bb and tet1 genes in pituitary (Figure 6A). While no variations were observed in the pituitary expression of other DNA methylation related genes (i.e., dnmt1, dnmt3a, dnmt3l and dmap1), a non-significative trend was noticed for dnmt1, tet2 and tet3, with increments in their expression values after DAC exposure (Figure 6A). Conversely, DAC produced no variations in the expression of the methylation-related genes analyzed in liver explants (Figure 6B).
Figure 6. Effects of 24 h exposure of explants to 5-Aza-2′-deoxycytidine (DAC) on the expression of DNA methylation remodeling related genes (dnmt1, dnmt3a, dnmt3bb, dnmt3l, dmap1, tet1, tet2, tet3). (A) pituitary. (B) liver explants Control versus treated explants compared by t-test (p < 0.05). *p < 0.05.
DNMT activity in pituitary and liver exhibited no significant variations due to DAC, but a trend for DNMT activity to be higher in treated pituitaries (Figure 7A) was observed. However, TET activity did increase after DAC exposure in pituitary (Figure 7A), while remained the same in liver explants (Figure 7B).
Figure 7. Effects of 24 h exposure of pituitary and liver explants to 5-Aza-2′-deoxycytidine (DAC) on the activity of DNA methylation remodeling enzymes. (A) DNA methyltransferase (DNMT) activity. (B) Ten-eleven translocation (TET) methylcytosine dioxygenases activity. Control versus treated explants compared by t-test (p < 0.05). *p < 0.05.
DAC produced opposing effects on DNA methylation and hydroxymethylation within each tissue (Figure 8), though statistical differences were found only for hydroxymethylation in pituitary, which increased due to DAC treatment (Figure 8C).
Figure 8. Effects of 24 h exposure of pituitary and liver explants to 5-Aza-2′-deoxycytidine (DAC) on DNA methylation (%5 mC) and in hydroxymethylation (%5 hm C). (A) pituitary, 5 mC. (B) liver explants, 5 mC. (C) pituitary, 5 hm C. (D) liver explants, 5 hm C. Control versus treated explants compared by t-test (p < 0.05). *p < 0.05.
Although temperature, photoperiod, salinity, pollutants, stocking density, among other factors, affect GH and IGF-I expression and growth rate in fish (Triantaphyllopoulos et al., 2020), the main environmental factor regulating their GH/IGF-I axis is the nutritional status (Pérez-Sánchez et al., 2018; Bertucci et al., 2019). Nutrition may affect DNA methylation by altering the availability of methyl donors (e.g., SAM), by changing the activity of enzymes responsible for the production of SAM, or by activating or inhibiting DNMTs and TETs enzymes (Kadayifci et al., 2018; Skjærven et al., 2022). There are now several lines of evidence indicating that DNA methylation/hydroxymethylation are involved in the modulation of GH/IGF-I axis in fish (Li et al., 2017; Huang et al., 2018; Podgorniak et al., 2019; Konstantinidis et al., 2021). While nutritional interventions targeting the epigenome are a new avenue to improve growth and other phenotypes in farmed fish (Gavery and Roberts, 2017), little is known in most species. In this work, we evaluated the value of tissue explants as tools for broadening our understanding of GH/IGF-I axis regulation in a farmed fish, the gilthead seabream, and to study the effects of epigenetic modulators on this axis.
We demonstrated that fish pituitaries and liver explants are viable and functional during 48 h. This experimental window is equal or longer than that used in 78% of the research published in the last decade with PCLS (Dewyse et al., 2021), while explants are cheaper and easier to implement than PCLS. Also, despite the long history of explant culture in fish, many articles never indicate why specific culture conditions were chosen and others provide incomplete descriptions of explant health (LeClair, 2021). Here, we described in detail the optimization process for pituitary and, in particular, liver explants, which resulted problematic in first attempts. LDH is a cytosolic enzyme released into the cell culture medium upon plasma membrane leakage and it has been widely used to assess viability in cultured cells and explants (Cox et al., 2021). Catalase, on the other hand, is one of the most important antioxidant enzymes and it is a reliable marker of oxidative stress (Kocalar et al., 2023). The value of both biomarkers in both tissues was demonstrated before their use during explant optimization. We proved that under our conditions, the cytoplasmic enzyme LDH is retained in the tissue, and that explants are able to respond to induced oxidative stress by increasing CAT activity, hence low CAT activity in untreated explants indicated low level of oxidative stress during culture. Also, we showed that explants were able to express and secrete hormones (GH in pituitary and IGF-I in liver explants) which make them suited to study GH-IGF-I axis.
As histological examination of organ culture is essential to ensure a healthy tissue (LeClair, 2021), our observations were complemented by histology, which revealed that both explants retain their structural integrity and organization. Moreover, we showed that cell proliferation occurred in the explants as in the live fish. This represents additional evidence of viability, being also relevant in the context of epigenetic studies as it may determine the balance between the two modes of action of DNA methylation inhibitors such as DAC. DAC needs cell proliferation to be passively incorporated into DNA and block further DNA methylation by acting as a suicide substrate for DNMT, but can also decrease methylation in the absence of cell division by replication-independent DNMT depletion (Pleyer and Grei, 2015). In this sense, the absence or excess of cell proliferation, which do not occur in our explants, may lead to in vitro result that cannot be used for in vivo extrapolation.
By exposing explants to SAM, GEN and DAC, we explored the possibility to modulate GH-IGF-I axis genes through interventions targeting the epigenome, by affecting overall DNA methylation potential or inhibiting DNMTs. SAM is the main methyl donor in cells, GEN is a plant-derived phytoestrogen that decreases DNMT activity probably by interacting with the catalytic domain of the enzymes (Xie et al., 2014), and DAC is a demethylating agent targeting more strongly DNMT1, although also affecting DNMT3s (Pleyer and Grei, 2015).
Overall, GEN and DAC produced similar effects on gene expression in this study, but we acknowledge that the mechanisms are likely not the same, and in the case of GEN, not necessarily linked with changes in DNA methylation. Although both are inhibitors of DNA methylation, genistein modulates the expression of DNMTs and other chromatin modifiers such as histone deacetylases (HDAC), inhibits the enzymatic activity of DNMTs, HDACs, and histone methyltransferase (HMTs), and can also bind to estrogen receptors activating different pathways (Sundaram et al., 2019). In contrast, DAC targets directly the DNA and binds to the catalytic binding domain of DNMTs (Pleyer and Grei, 2015). Thus, at least for DAC, the observed effects on gene expression are likely to be caused mainly through altering DNA methylation. We found that GEN and DAC increased the expression of igfbp2a, and decreased the expression of igfbp4 and both GH receptors. In rainbow trout (Oncorhynchus mykiss), the intraperitoneal injection of GEN also reduced expression of both GH receptors (Cleveland and Manor, 2015). However, in that study, no differences were found for igfbp4 and igfbp2a, which were responsive in our in vitro assays in gilthead seabream. Changes in expression of all igfbp2a, igfbp4, ghri and ghrii after DAC treatment suggest that these genes are modulated, directly or indirectly, by DNA methylation in seabream. Other studies have found evidences of this regulation for igfbp2 in fish (Podgorniak et al., 2019), mice (Kammel et al., 2016) and humans (Boughanem et al., 2021), for igfbp4 in sheep (Tian et al., 2022) and humans (Diaz et al., 2020) and for ghrs in fish (Zhao et al., 2015) and broilers (Cong et al., 2023). Finally, while changes in DNA methylation at the igf1 gene of some fish were reported (Li et al., 2017; Huang et al., 2018), we found no evidence of such regulation in the gilthead seabream as judged by the lack of response of this gene to DAC. However, it is worth mentioning that in this study, total igf1 was measured (i.e., primers annealing to all three forms; Tiago et al., 2008), and the effects on the three igf1 should be further assessed. IGF-Ic is the most highly expressed form in the liver and it is thought to play systemic roles, while the other transcripts may have a local action (Tiago et al., 2008).
It is difficult to predict the consequences for the fish of the expression pattern produced in vitro by DAC. In higher vertebrates, IGFBP2 is believed to bind both IGF-I and IGF-II, modulates IGFs systemic actions, but also acts locally preventing adipogenesis (Boughanem et al., 2021). However, igfbp2b, which comprise more that 70% of igfbp mRNAs in the gilthead sea bream (Pérez-Sánchez et al., 2018) was unaffected in our study, and the role of igfbp2a paralog, which was upregulated by GEN and DAC, is poorly understood though suspected to have also growth inhibitory activity. Yet, igfbp4 is also abundant in this species, accounting for more than 20% of igfbp mRNAs (Pérez-Sánchez et al., 2018), and was downregulated by GEN and DAC in this study. This igfbp has growth promoting effects in some fish (Garcia de la Serrana and Macqueen, 2018) thus its reduction might compromise normal growth. Also, in this line, the other two genes downregulated by GEN and DAC were GH receptors, which are crucial for initiating GH signal transduction pathways (Pérez-Sánchez et al., 2018). Besides, because of the functional diversification of ghr in fish, both GEN and DAC may affect different physiological processes in the fish, as ghri has been more clearly related with growth while ghrii is more related with stress response (Saera-Vila et al., 2007; 2009; Pérez-Sánchez et al., 2018). As the gene expression pattern obtained after treatments with DNA methylation inhibitors is suggestive of impaired growth, it is proposed that a proper methylation environment within the liver may ensure growth in this fish species as shown in salmon (Saito et al., 2021; Adam et al., 2022). An in vivo grow out trial is underway to clarify this issue, which would have practical implications in feed design for this fish species.
Nutritional interventions such as the inclusion of DNA methylation inhibitors or enhancers imply that all tissues become exposed, though at different concentrations, to the agents. Thus, it is important to establish if they have synergist or overriding effects on different tissues. SAM supplementation of culture media is known to surprisingly causes both increase and decrease in DNA methylation in different liver cell lines (Wang et al., 2017). We did not address this issue because no effects of SAM exposure on the expression of GH-IGF-I axis genes analyzed were found in liver and pituitary explants. However, this does not mean that SAM had no effect on DNA methylation of explants, which is worthy to be investigated in further studies. Direct evidence of the impact of DNA methylation changes, if occurs, on a gene expression is often difficult to obtain. Indeed, it has been shown in salmon that one-carbon metabolism nutrients affected DNA methylation profiles of genes involved in various cellular processes without directly changing their gene expression in the liver (Saito et al., 2021), and a similar lack of clear relationship was observed for different growth-related genes in large yellow croaker (Larimichthys crocea) (Zhang et al., 2019). Also, it should be considered that in addition to DNA, SAM can transfer its methyl group to a large variety of acceptor substrates, including RNA and proteins (Cederbaum, 2010), and other nutrients may be more effective to produce an increase in the methylation potential of tissues (Kharbanda et al., 2005; Saito et al., 2021). Liver explants described here would be useful for screening the potential of those nutrients to produce changes in DNA methylation.
Conversely, here it is showed that DAC produces different effects on liver and pituitary DNA methylation remodeling. While no variations were observed in DAC-treated liver explants in the expression of the DNA methylation remodeling genes studied, in DNMT or TET activities, and accordingly, nor in overall 5 mC and 5 h m, pituitary explant were more responsive. DAC increased the expression of dnmt3bb and tet1 in pituitary, producing increments in both DNMT and TET activity, though more pronounced for TET. Likewise, in other models (i.e., colorectal cancer cells), DAC increased DNMT3A and TETs gene expression (Gerecke et al., 2018). A non-statistically significant increase in dnmt1 was observed in pituitary due to DAC exposure. In mouse this gene contains a methylation-sensitive DNA element, which after being demethylated by 5-azadeoxycytidine, results in the induction of dnmt1 expression (Slack et al., 1999), although opposed results have been obtained in colon cancer cells (Gerecke et al., 2018). Despite DAC increased both hypermethylation and demethylation potential in fish pituitary as reported before in mice (Colwell et al., 2021), the overall result in our assay was only an increase in DNA hydroxymethylation (5 hm C), likely due to a more pronounced increase in TET activity. These enzymes regulate active turnover of DNA methylation by oxidizing 5 mC iteratively to yield first 5 hm C and then two other intermediates (5-formylcytosine, 5 fC; 5-carboxycytosine, 5caC) that can be excised and return to unmethylated cytosines by thymine-DNA glycosylase (TDG)-mediated base excision repair (Zhang et al., 2023). However, full demethylation is not always accomplished, and there are strong evidences supporting that 5 hm C is also a stable epigenetic mark, and not only a demethylation intermediate (Zhang et al., 2023). Indeed, it has been proposed that cytosine hydroxymethylation may contribute to the phenotypic plasticity of growth through epigenetic regulation of the somatotropic axis in Nile tilapia (Konstantinidis et al., 2021). Thus, pituitary explant cultures reported here proved to be useful to gain more insights on this regulation in fish. In addition, it is worth mentioning that site-specific de novo hypermethylation due to the action of dnmt3bb would be physiologically relevant in pituitary and deserves further examination.
Collectively, our results demonstrate that exposure to a common DNA methylation remodeling agent produces tissue-specific responses in their endogenous DNA methylation machinery, and this may in turn determine the effects in each tissue. Regarding the gilthead seabream tissues examined, pituitary seems to respond stronger to DAC and to have more broad effects on DNA methylation/hydroxymethylation than liver. Yet, although less evident at the overall level, changes in liver seems to be functionally relevant as DAC had clear effects on the expression of some genes from the IGF-I axis. This study highlights the need for genome-wide single-base resolution approaches to truly assess the processes modulated through epigenetics and their regulatory mechanisms in both tissues, for which tissue explants stand as a suitable option, also in line with the principles of the 3Rs (Replacement, Reduction and Refinement).
This study demonstrates that tissue explants of liver and pituitary are useful for studying epigenetic mechanisms and GH/IGF-I axis regulation in fish. Explants can be used in further studies for the screening of nutrients, additives, and environmental conditions with putative epigenetic effects on important physiological processes performed by pituitary and liver. Evidences provided in this study suggest that DNA methylation or hydroxymethylation may be involved in the regulation of some genes of the GH/IGF-I axis in gilthead seabream. However, it must be argued that we did not attempt to establish a direct connection between DNA methylation and gene expression with our experiment or results. This relationship is hard to disentangle by target-specific approaches without previous information on the genomic regions affected. For instance, changes in DNA methylation several kilobases away from a gene may impact its expression, have different effects depending on the genomic feature affected, or may have occurred not in the studied genes but in other genes upstream in their regulation cascade. An ongoing study at our laboratory is addressing this issue by genome-wide single-base resolution approaches, using herein described pituitary and liver explants as experimental models.
The raw data supporting the conclusion of this article will be made available by the authors, without undue reservation.
The animal study was approved by Spanish National Research Council (CSIC) Bioethical Committee and Autonomous Andalusian Government (reference number 24/10/2023/092). The study was conducted in accordance with the local legislation and institutional requirements.
EP: Conceptualization, Data curation, Formal Analysis, Funding acquisition, Investigation, Methodology, Project administration, Resources, Software, Supervision, Validation, Visualization, Writing–original draft, Writing–review and editing. JR-P: Formal Analysis, Investigation, Writing–review and editing. JH-P: Formal Analysis, Investigation, Writing–review and editing. RH-C: Formal Analysis, Investigation, Writing–review and editing. MY: Formal Analysis, Investigation, Writing–review and editing. JM: Formal Analysis, Investigation, Writing–review and editing. JM-S: Formal Analysis, Investigation, Writing–review and editing. GM-R: Formal Analysis, Investigation, Writing–review and editing. JO-D: Formal Analysis, Investigation, Writing–review and editing. CN-G: Formal Analysis, Investigation, Writing–review and editing. JR-C: Formal Analysis, Investigation, Writing–review and editing.
The author(s) declare that financial support was received for the research, authorship, and/or publication of this article. This research was funded by the project BreamEpiMap (Grant: PID 2021-128540OA-I00) funded by MCIN/AEI/10.13039/501100011033 and by “ERDF A way of making Europe”, and by project epiMODEL2 (ref 202240I011, CSIC), both granted to EP. CN-G was supported by the researcher contract DOC_01203 funded by the Andalusian Plan for Research Development and Innovation (PAIDI 2020). JR-P was supported by ALEHOOP Project N° 887259 H2020-BBI-JTI-2019. JH-P was supported by program Garantía Juvenil from European Union through Junta de Andalucía, Spain, and RH-C by program Investigo financed by the European Union, NextGenerationEU through the Spanish Recovery, Transformation and Resilience Plan (RRTP). The funders had no role in study design, data collection and analysis, decision to publish, or preparation of the manuscript.
The authors declare that the research was conducted in the absence of any commercial or financial relationships that could be construed as a potential conflict of interest.
All claims expressed in this article are solely those of the authors and do not necessarily represent those of their affiliated organizations, or those of the publisher, the editors and the reviewers. Any product that may be evaluated in this article, or claim that may be made by its manufacturer, is not guaranteed or endorsed by the publisher.
The Supplementary Material for this article can be found online at: https://www.frontiersin.org/articles/10.3389/fphys.2024.1410660/full#supplementary-material
18S rRNA 18S ribosomal RNA; 5-hmC 5-hydroxymethylcitocine; 5-mC 5-methylcytocine; ACTB β actin; CAT catalase activity; DAC 5-Aza-2′-deoxycytidine; DMAP1 DNA Methyltransferase 1 Associated Protein 1; DNMT DNA Methyltransferase; EF1A elongation factor 1 α; GAPDH glyceraldehyde-3-phosphate dehydrogenase; GEN genistein; GH growth hormone; GHR GH receptor; GHREs growth hormone response elements; IGFBPs insulin-like growth factor binding proteins; IGF-I insulin-like growth factor I; LDH lactate dehydrogenase; PCNA proliferating cell nuclear antigen; PMS post-mitochondrial supernatant; RPL27A ribosomal protein L27; RPS18 ribosomal protein S18; SAM S-adenosylmethionine; TET tet methylcytosine dioxygenase.
Adam A. C., Saito T., Espe M., Whatmore P., Fernandes J. M. O., Vikeså V., et al. (2022). Metabolic and molecular signatures of improved growth in Atlantic salmon (Salmo salar) fed surplus levels of methionine, folic acid, vitamin B6 and B12 throughout smoltification. Br. J. Nutr. 127 (9), 1289–1302. doi:10.1017/S0007114521002336
Amri A., Kessabi K., Bouraoui Z., Sakli S., Gharred T., Guerbej H., et al. (2020). Effect of melatonin and folic acid supplementation on the growth performance, antioxidant status, and liver histology of the farmed gilthead sea bream (Sparus aurata L.) under standard rearing conditions. Fish. Physiol. Biochem. 46, 2265–2280. doi:10.1007/s10695-020-00879-5
Andersen C. L., Ledet-Jensen J., Ørntoft T. (2004). Normalization of real-time quantitative reverse transcription-PCR data: a model-based variance estimation approach to identify genes suited for normalization, applied to bladder and colon cancer data sets. Cancer Res. 64, 5245–5250. doi:10.1158/0008-5472.CAN-04-0496
Antequera F., Boyes J., Bird A. (1990). High levels of de novo methylation and altered chromatin structure at CpG islands in cell lines. Cell 62, 503–514. doi:10.1016/0092-8674(90)90015-7
Astola A., Calduch-Giner J. A., Ortiz M., Pérez-Sánchez J., Valdivia M. M. (2004). Genomic structure and functional analysis of promoter region of somatolactin gene of sea bream (Sparus aurata). Mar. Biotechnol. (NY). 6 (5), 411–418. doi:10.1007/s10126-004-3210-2
Beitel S. C., Doering J. A., Eisner B. K., Hecker M. (2015). Comparison of the sensitivity of four native Canadian fish species to 17-α ethinylestradiol, using an in vitro liver explant assay. Environ. Sci. Pollut. Res. Int. 22 (24), 20186–20197. doi:10.1007/s11356-015-5101-7
Bertucci J. I., Blanco A. M., Sundarrajan L., Rajeswari J. J., Velasco C., Unniappan S. (2019). Nutrient regulation of endocrine factors influencing feeding and growth in fish. Front. Endocrinol. 10, 83. doi:10.3389/fendo.2019.00083
Boughanem H., Yubero-Serrano E. M., López-Miranda J., Tinahones F. J., Macias-Gonzalez M. (2021). Potential role of insulin growth-factor-binding protein 2 as therapeutic target for obesity-related insulin resistance. Int. J. Mol. Sci. 22 (3), 1133. doi:10.3390/ijms22031133
Cánepa M., Pozzi A., Astola A., Maggese M. C., Vissio P. (2008). Effect of salmon melanin-concentrating hormone and mammalian gonadotrophin-releasing hormone on somatolactin release in pituitary culture of Cichlasoma dimerus. Cell Tissue Res. 333 (1), 49–59. doi:10.1007/s00441-008-0622-8
Cederbaum A. I. (2010). Hepatoprotective effects of S-adenosyl-L-methionine against alcohol- and cytochrome P450 2E1-induced liver injury. World J. Gastroenterol. 21 (11), 1366–1376. doi:10.3748/wjg.v16.i11.1366
Clairborne A. (1985). “Catalase activity,” in Handbook methods for oxygen radical research. Editor R. A. Greenwald (Boca Raton, FL, USA: CRC Press), 283–284.
Cleveland B. M., Manor M. L. (2015). Effects of phytoestrogens on growth-related and lipogenic genes in rainbow trout (Oncorhynchus mykiss). Comp. Biochem. Physiol. C Toxicol. Pharmacol. 170, 28–37. doi:10.1016/j.cbpc.2015.02.001
Colwell M., Wanner N. M., Drown C., Drown M., Dolinoy D. C., Faulk C. (2021). Paradoxical whole genome DNA methylation dynamics of 5’aza-deoxycytidine in chronic low-dose exposure in mice. Epigenetics 16 (2), 209–227. doi:10.1080/15592294.2020.1790951
Cong W., Han W., Liu J., Zhao R., Wu L. (2023). Embryonic thermal manipulation leads growth inhibition and reduced hepatic insulin-like growth factor1 expression due to promoter DNA hypermethylation in broilers. Poult. Sci. 102 (4), 102562. doi:10.1016/j.psj.2023.102562
Cox M. C., Mendes R., Silva F., Mendes T. F., Zelaya-Lazo A., Halwachs K., et al. (2021). Application of LDH assay for therapeutic efficacy evaluation of ex vivo tumor models. Sci. Rep. 11, 18571. doi:10.1038/s41598-021-97894-0
Dewyse L., Reynaert H., van Grunsven L. A. (2021). Best practices and progress in precision-cut liver slice cultures. Int. J. Mol. Sci. 22, 7137. doi:10.3390/ijms22137137
Diaz M., Garde E., Lopez-Bermejo A., de Zegher F., Ibañez L. (2020). Differential DNA methylation profile in infants born small-for-gestational-age: association with markers of adiposity and insulin resistance from birth to age 24 months. BMJ Open Diabetes Res. 8, e001402. doi:10.1136/bmjdrc-2020-001402
Doering J. A., Beitel S. C., Eisner B. K., Heide T., Hollert H., Giesy J. P., et al. (2015). Identification and response to metals of metallothionein in two ancient fishes: white sturgeon (Acipenser transmontanus) and lake sturgeon (Acipenser fulvescens). Comp. Biochem. Physiol. C Toxicol. Pharmacol. 171, 41–48. doi:10.1016/j.cbpc.2015.03.002
Eisner B. K., Doering J. A., Beitel S. C., Wiseman S., Raine J. C., Hecker M. (2016). Cross-species comparison of relative potencies and relative sensitivities of fishes to dibenzo-p-dioxins, dibenzofurans, and polychlorinated biphenyls in vitro. Environ. Toxicol. Chem. 35 (1), 173–181. doi:10.1002/etc.3173
Fu Q., McKnight R. A., Callaway C. W., Yu X., Lane R. H., Majnik A. V. (2015). Intrauterine growth restriction disrupts developmental epigenetics around distal growth hormone response elements on the rat hepatic IGF-1 gene. FASEB J. 29 (4), 1176–1184. doi:10.1096/fj.14-258442
Fuentes J., Brinca L., Guerreiro P. M., Power D. M. (2010). PRL and GH synthesis and release from the sea bream (Sparus auratus L.) pituitary gland in vitro in response to osmotic challenge. Gen. Comp. Endocrinol. 168 (1), 95–102. doi:10.1016/j.ygcen.2010.04.005
Garcia de la Serrana D., Macqueen D. J. (2018). Insulin-like growth factor-binding proteins of teleost fishes. Front. Endocrinol. 9, 80. doi:10.3389/fendo.2018.00080
Gavery M. R., Roberts S. B. (2017). Epigenetic considerations in aquaculture. PeerJ 5, e4147. doi:10.7717/peerj.4147
Gerecke C., Schumacher F., Edlich A., Wetzel A., Yealland G., Neubert L. K., et al. (2018). Vitamin C promotes decitabine or azacytidine induced DNA hydroxymethylation and subsequent reactivation of the epigenetically silenced tumour suppressor CDKN1A in colon cancer cells. Oncotarget 9 (67), 32822–32840. doi:10.18632/oncotarget.25999
Glencross B., Fracalossi D. M., Hua K., Izquierdo M., Mai K., Øverland M., et al. (2023). Harvesting the benefits of nutritional research to address global challenges in the 21st century. J. World. Aquac. Soc. 54 (2), 343–363. doi:10.1111/jwas.12948
Helms L. M., Grau E. G., Shimoda S. K., Nishioka R. S., Bern H. A. (1987). Studies on the regulation of growth hormone release from the proximal pars distalis of male tilapia, Oreochromis mossambicus, in vitro. Gen. Comp. Endocrinol. 65 (1), 48–55. doi:10.1016/0016-6480(87)90221-8
Huang Y., Wen H., Zhang M., Hu N., Si Y., Li S., et al. (2018). The DNA methylation status of MyoD and IGF-I genes are correlated with muscle growth during different developmental stages of Japanese flounder (Paralichthys olivaceus). Comp. Biochem. Physiol. B Biochem. Mol. Biol. 219-220, 33–43. doi:10.1016/j.cbpb.2018.02.005
Janssen K., Saatkamp H., Komen H. (2018). Cost-benefit analysis of aquaculture breeding programs. Genet. Sel. Evol. 50, 2. doi:10.1186/s12711-018-0372-3
Kadayifci F. Z., Zheng S., Pan Y. X. (2018). Molecular mechanisms underlying the link between diet and DNA methylation. Int. J. Mol. Sci. 19 (12), 4055. doi:10.3390/ijms19124055
Kammel A., Saussenthaler S., Jähnert M., Jonas W., Stirm L., Hoeflich A., et al. (2016). Early hypermethylation of hepatic Igfbp2 results in its reduced expression preceding fatty liver in mice. Hum. Mol. Genet. 25, 2588–2599. doi:10.1093/hmg/ddw121
Kharbanda K. K., Rogers D. D., Mailliard M. E., Siford G. L., Barak A. J., Beckenhauer H. C., et al. (2005). A comparison of the effects of betaine and S-adenosylmethionine on ethanol-induced changes in methionine metabolism and steatosis in rat hepatocytes. J. Nutr. 135 (3), 519–524. doi:10.1093/jn/135.3.519
Kocalar K., Canli E. G., Canli M. (2023). Responses of oxidative stress biomarkers of freshwater fish (Oreochromis niloticus) exposed to Cr6+, Hg2+, Ni2+ and Zn2+ in differing calcium levels. Comp. Biochem. Physiol. C Toxicol. Pharmacol. 267, 109577. doi:10.1016/j.cbpc.2023.109577
Konstantinidis I., Anastasiadi D., Sætrom P., Nedoluzhko A. V., Mjelle R., Podgorniak T., et al. (2021). Epigenetic mapping of the somatotropic axis in Nile tilapia reveals differential DNA hydroxymethylation marks associated with growth. Genomics 113 (5), 2953–2964. doi:10.1016/j.ygeno.2021.06.037
LeClair E. E. (2021). The last half century of fish explant and organ culture. Zebrafish 18 (1), 1–19. doi:10.1089/zeb.2020.1935
Li S., He F., Wen H., Li J., Si Y., Liu M., et al. (2017). Low salinity affects cellularity, DNA methylation, and mRNA expression of igf1 in the liver of half smooth tongue sole (Cynoglossus semilaevis). Fish. Physiol. Biochem. 43, 1587–1602. doi:10.1007/s10695-017-0395-7
Livak K. J., Schmittgen T. D. (2001). Analysis of relative gene expression data using real-time quantitative PCR and the 2(-Delta Delta C(T)) Method. Methods 25, 402–408. doi:10.1006/meth.2001.1262
Luna L. G. (1968). Manual of histologic staining methods of the armed forces Institute of pathology. 3rd Edition. New York: McGraw-Hill.
Ndandala C. B., Dai M., Mustapha U. F., Li X., Liu J., Huang H., et al. (2022). Current research and future perspectives of GH and IGFs family genes in somatic growth and reproduction of teleost fish. Aquac. Rep. 26, 101289. doi:10.1016/j.aqrep.2022.101289
Nestor C. E., Ottaviano R., Reinhardt D., Cruickshanks H. A., Mjoseng H. K., McPherson R. C., et al. (2015). Rapid reprogramming of epigenetic and transcriptional profiles in mammalian culture systems. Genome Biol. 16, 11. doi:10.1186/s13059-014-0576-y
Ortiz-Delgado J. B., Porcelloni S., Fossi C., Sarasquete C. (2008). Caracterización histoquímica de ovocitos del pez espada, <i>Xiphias gladius</i>. Sci. Mar. 72 (3), 549–564. doi:10.3989/scimar.2008.72n3549
Ouni M., Belot M. P., Castell A. L., Fradin D., Bougneres P. (2016). The P2 promoter of the IGF1 gene is a major epigenetic locus for GH responsiveness. Pharm. J. 16, 102–106. doi:10.1038/tpj.2015.26
Pampaloni F., Reynaud E., Stelzer E. (2007). The third dimension bridges the gap between cell culture and live tissue. Nat. Rev. Mol. Cell Biol. 8, 839–845. doi:10.1038/nrm2236
Pérez-Sánchez J., Simó-Mirabet P., Naya-Català F., Martos-Sitcha J. A., Perera E., Bermejo-Nogales A., et al. (2018). Somatotropic Axis regulation unravels the differential effects of nutritional and environmental factors in growth performance of marine farmed fishes. Front. Endocrinol. 9, 687. doi:10.3389/fendo.2018.00687
Piñuela C., Rendón C., González de Canales M. L., Sarasquete C. (2004). Development of the Senegal sole, Solea senegalensis forebrain. Eur. J. Histochem. 48 (4), 377–384. doi:10.4081/911
Pleyer L., Greil R. (2015). Digging deep into "dirty" drugs - modulation of the methylation machinery. Drug Metab. Rev. 47 (2), 252–279. doi:10.3109/03602532.2014.995379
Podgorniak T., Brockmann S., Konstantinidis I., Fernandes J. M. O. (2019). Differences in the fast muscle methylome provide insight into sex-specific epigenetic regulation of growth in Nile tilapia during early stages of domestication. Epigenetics 14, 818–836. doi:10.1080/15592294.2019.1618164
Ransy C., Vaz C., Lombès A., Bouillaud F. (2020). Use of H2O2 to cause oxidative stress, the catalase issue. Int. J. Mol. Sci. 21 (23), 9149. doi:10.3390/ijms21239149
Rodríguez-Viera L., Martí I., Martínez R., Perera E., Estrada M. P., Mancera J. M., et al. (2022). Feed supplementation with the GHRP-6 peptide, a ghrelin analog, improves feed intake, growth performance and aerobic metabolism in the gilthead sea bream Sparus aurata. Fishes 7 (1), 31. doi:10.3390/fishes7010031
Saera-Vila A., Calduch-Giner J. A., Pérez-Sánchez J. (2007). Co-expression of IGFs and GH receptors (GHRs) in gilthead sea bream (Sparus aurata L.): sequence analysis of the GHR-flanking region. J. Endocrinol. 194 (2), 361–372. doi:10.1677/JOE-06-0229
Saera-Vila A., Calduch-Giner J. A., Prunet P., Pérez-Sánchez J. (2009). Dynamics of liver GH/IGF axis and selected stress markers in juvenile gilthead sea bream (Sparus aurata) exposed to acute confinement: differential stress response of growth hormone receptors. Comp. Biochem. Physiol. A Mol. Integr. Physiol. 154 (2), 197–203. doi:10.1016/j.cbpa.2009.06.004
Saito T., Whatmore P., Taylor J. F., Fernandes J. M. O., Adam A. C., Tocher D. R., et al. (2021). Micronutrient supplementation affects transcriptional and epigenetic regulation of lipid metabolism in a dose-dependent manner. Epigenetics 16 (11), 1217–1234. doi:10.1080/15592294.2020.1859867
Skjærven K. H., Adam A. C., Takaya S., Waagbø R., Espe M. 2022 Nutritional epigenetics (chapter 5) 161- 192 pp. In: Cellular and molecular approaches in fish biology. United States Academic Press. doi:10.1016/B978-0-12-822273-7.00006-9
Slack A., Cervoni N., Pinard M., Szyf M. (1999). Feedback regulation of DNA methyltransferase gene expression by methylation. Eur. J. Biochem. 264 (1), 191–199. doi:10.1046/j.1432-1327.1999.00603.x
Sundaram M. K., Unni S., Somvanshi P., Bhardwaj T., Mandal R. K., Hussain A., et al. (2019). Genistein modulates signaling pathways and targets several epigenetic markers in HeLa cells. Genes 10, 955. doi:10.3390/genes10120955
Tiago D. M., Laizé V., Cancela M. L. (2008). Alternatively spliced transcripts of Sparus aurata insulin-like growth factor 1 are differentially expressed in adult tissues and during early development. Gen. Comp. Endocrinol. 157, 107–115. doi:10.1016/j.ygcen.2008.04.006
Tian Y., Du J., Yang X., Zeng W., He J., Zhao B., et al. (2022). Sheep IGFBP2 and IGFBP4 promoter methylation regulates gene expression and hair follicle development. Electron. J. Biotechnol. 59, 46–54. doi:10.1016/j.ejbt.2022.07.001
Triantaphyllopoulos K. A., Cartas D., Miliou H. (2020). Factors influencing GH and IGF-I gene expression on growth in teleost fish: how can aquaculture industry benefit? Rev. Aquacult. 12, 1637–1662. doi:10.1111/raq.12402
Vélez E. J., Unniappan S. A. (2021). A comparative update on the neuroendocrine regulation of growth hormone in vertebrates. Front. Endocrinol. 11, 1174. doi:10.3389/fendo.2020.614981
Wang Y., Sun Z., Szyf M. (2017). S-adenosyl-methionine (SAM) alters the transcriptome and methylome and specifically blocks growth and invasiveness of liver cancer cells. Oncotarget 8 (67), 111866–111881. doi:10.18632/oncotarget.22942
Xie Q., Bai Q., Zou L. Y., Zhang Q. Y., Zhou Y., Chang H., et al. (2014). Genistein inhibits DNA methylation and increases expression of tumor suppressor genes in human breast cancer cells. Genes Chromosom. Cancer 53 (5), 422–431. doi:10.1002/gcc.22154
Yu R. M., Ng P. K., Tan T., Chu D. L., Wu R. S., Kong R. Y. (2008). Enhancement of hypoxia-induced gene expression in fish liver by the aryl hydrocarbon receptor (AhR) ligand, benzo[a]pyrene (BaP). Aquat. Toxicol. 90 (3), 235–242. doi:10.1016/j.aquatox.2008.09.004
Zhang X., Zhang Y., Wang C., Wang X. (2023). TET (Ten-eleven translocation) family proteins: structure, biological functions and applications. Sig. Transduct. Target. Ther. 8, 297. doi:10.1038/s41392-023-01537-x
Zhang Y., Shen W., Cao M., Li J., Zheng B., Lou Z., et al. (2019). Dynamic alterations in methylation of global DNA and growth-related genes in large yellow croaker (Larimichthys crocea) in response to starvation stress. Comp. Biochem. Physiol. B Biochem. Mol. Biol. 227, 98–105. doi:10.1016/j.cbpb.2018.09.006
Zhao J. L., Si Y. F., He F., Wen H. S., Li J. F., Ren Y. Y., et al. (2015). Polymorphisms and DNA methylation level in the CpG site of the GHR1 gene associated with mRNA expression, growth traits and hormone level of half-smooth tongue sole (Cynoglossus semilaevis). Fish. Physiol. Biochem. 41 (4), 853–865. doi:10.1007/s10695-015-0052-y
Keywords: DNA methylation, epigenetics, fish, GH-IGF axis, in vitro culture, tissue explant
Citation: Perera E, Román-Padilla J, Hidalgo-Pérez JA, Huesa-Cerdán R, Yúfera M, Mancera JM, Martos-Sitcha JA, Martínez-Rodríguez G, Ortiz-Delgado JB, Navarro-Guillén C and Rodriguez-Casariego JA (2024) Tissue explants as tools for studying the epigenetic modulation of the GH-IGF-I axis in farmed fish. Front. Physiol. 15:1410660. doi: 10.3389/fphys.2024.1410660
Received: 01 April 2024; Accepted: 03 June 2024;
Published: 20 June 2024.
Edited by:
Jose Fernando Lopez-Olmeda, University of Murcia, SpainReviewed by:
Ashis Saha, Central Institute of Freshwater Aquaculture (ICAR), IndiaCopyright © 2024 Perera, Román-Padilla, Hidalgo-Pérez, Huesa-Cerdán, Yúfera, Mancera, Martos-Sitcha, Martínez-Rodríguez, Ortiz-Delgado, Navarro-Guillén and Rodriguez-Casariego. This is an open-access article distributed under the terms of the Creative Commons Attribution License (CC BY). The use, distribution or reproduction in other forums is permitted, provided the original author(s) and the copyright owner(s) are credited and that the original publication in this journal is cited, in accordance with accepted academic practice. No use, distribution or reproduction is permitted which does not comply with these terms.
*Correspondence: Erick Perera, ZXJpY2sucGVyZXJhQGNzaWMuZXM=
Disclaimer: All claims expressed in this article are solely those of the authors and do not necessarily represent those of their affiliated organizations, or those of the publisher, the editors and the reviewers. Any product that may be evaluated in this article or claim that may be made by its manufacturer is not guaranteed or endorsed by the publisher.
Research integrity at Frontiers
Learn more about the work of our research integrity team to safeguard the quality of each article we publish.