- Key Laboratory of Biorheological Science and Technology, Ministry of Education, College of Bioengineering, Chongqing University, Chongqing, China
Introduction: The integrity of the erythrocyte membrane cytoskeletal network controls the morphology, specific surface area, material exchange, and state of erythrocytes in the blood circulation. The antioxidant properties of resveratrol have been reported, but studies on the effect of resveratrol on the hypoxia-induced mechanical properties of erythrocytes are rare.
Methods: In this study, the effects of different concentrations of resveratrol on the protection of red blood cell mor-phology and changes in intracellular redox levels were examined to select an appropriate concentration for further study. The Young’s modulus and surface roughness of the red blood cells and blood viscosity were measured via atomic force microsco-py and a blood rheometer, respectively. Flow cytometry, free hemoglobin levels, and membrane lipid peroxidation levels were used to characterize cell membrane damage in the presence and absence of resveratrol after hypoxia. The effects of oxida-tive stress on the erythrocyte membrane proteins band 3 and spectrin were further investigated by immunofluorescent label-ing and Western blotting.
Results and discussion: Resveratrol changed the surface roughness and Young’s modulus of the erythrocyte mem-brane, reduced the rate of eryptosis in erythrocytes after hypoxia, and stabilized the intracellular redox level. Further data showed that resveratrol protected the erythrocyte membrane proteins band 3 and spectrin. Moreover, resistance to band 3 pro-tein tyrosine phosphorylation and sulfhydryl oxidation can protect the stability of the erythrocyte membrane skeleton net-work, thereby protecting erythrocyte deformability under hypoxia. The results of the present study may provide new insights into the roles of resveratrol in the prevention of hypoxia and as an antioxidant.
1 Introduction
Mature red blood cells (erythrocytes) are deformable and transport oxygen and nutrients by forming in a single row through capillaries that are smaller than their diameter, which is essential for the normal physiological activities of mammals. Erythrocytes have no nucleus or inner membrane system, and the lipid bilayer of the red blood cell membrane, embedded proteins, transmembrane proteins, and membrane skeleton network located below the lipid bilayer play decisive roles in regulating the deformability, oxygen-carrying, and oxygen-releasing abilities of red blood cells (Mohandas and Gallagher, 2008; Himbert and Rheinstädter, 2022). The deformability of erythrocytes depends on both shear resistance and tensile resistance. Furthermore, their deformity is also related to the integrity and stability of the membrane skeleton network. The binding of membrane proteins to the cytoskeleton allows erythrocytes to maintain good membrane surface area and cell integrity (Diez-Silva et al., 2010; Rossi et al., 2019). Band 3 and spectrin are the main proteins in the erythrocyte membrane cytoskeletal network. Band 3 is composed of two domains: a, abbreviated as the “transmembrane domain,” is a binding site for the membrane skeleton, glycolytic enzymes, and deoxyhemoglobin, and b, cytosolic binding domains that primarily form erythrocyte anion-exchange channels and contribute to carbon dioxide transport. (phosphofructokinase, aldolase, and glyceraldehyde-3-phosphate dehydrogenase) (Lux, 2016; Pretini et al., 2019) ensures the average elasticity of erythrocyte membranes and the deformability of red blood cells. Previous studies have shown that abnormal proteins on the erythrocyte membrane can lead to the appearance of spherical cells, changes in cell osmotic pressure, and impairments in the ability of cells to perform normal circulation functions (Vercellati et al., 2022). Abnormal membrane proteins not only affect the physiological and biochemical properties of cells but also lead to blockage of blood circulation. Studies have shown that red blood cell deformability is associated with many clinical manifestations (Shin et al., 2007; Caimi et al., 2018; Jasenovec et al., 2019). Moreover, the deformability of erythrocytes has been increasingly studied for blood storage and transfusion in vitro (Koch et al., 2019; Yoshida et al., 2019; Barshtein et al., 2020; 2021; Karafin et al., 2023), in addition to focusing on blood type, transfusion volume and blood quality. Based on the good deformability of red blood cells, the detection methods mainly include AFM to detect cell elasticity and EI index (Huisjes et al., 2018; Carelli-Alinovi et al., 2019; Yang et al., 2019; Lopes et al., 2023; Spinelli et al., 2023).
This amazing deformability of red blood cells has undergone various tests, the most important of which is the attack of reactive oxygen. When too much oxidant is produced, or when the endogenous antioxidant defense is inefficient, this balance may be disturbed and cells are attacked (Remigante and Morabito, 2022). Oxidative stress promotes human erythrocyte senescence (Remigante et al., 2022) Reactive oxygen species have been reported to cause protein kinase C (PKC) activation(Shan et al., 2020), PKC regulates erythrocyte deformability by phosphorylating bands 4.1, 4.9, and adducin, changing the binding affinity of these proteins to other membrane skeleton proteins (Govekar and Zingde, 2001). And, when exposed to high altitude hypoxia, hemorrhagic shock, or other hypoxic injuries, the deformability of erythrocytes is impaired. Further, the process by which cells respond in vivo to the hypoxic environment through regulation is multidimensional and involves complex signal transduction processes (Taylor and Moncada, 2010); for example, erythrocyte transglutaminase 2 (eTG2) has been found to act as an erythrocyte protein stabilizer that regulates oxygen delivery to alleviate hypoxia (Xu et al., 2022). Furthermore, researchers have suggested that a hypoxic environment is beneficial for the storage of red blood cells (Rabcuka et al., 2022; Kriebardis, 2023). Although inflammatory signals in vivo have an adaptive mechanism in response to hypoxia, inflammation levels beyond those that can be self-regulated lead to an inflammatory response that produces many free radicals and reactive oxygen species (ROS) (Pham-Huy et al., 2008; Pandey and Rizvi, 2010a). Due to the lack of biosynthetic mechanisms, erythrocytes cannot respond to hypoxic injury through protein synthesis, and thus, erythrocyte damage will accumulate uncontrollably (Badior and Casey, 2021). In addition, it has been found that oxidative stress can drain from red blood cells and trigger the damage of adjacent cells and tissues, leading to vascular injury (Carelli-Alinovi et al., 2015). Clinically, the interaction between hypoxia and inflammation may lead to inflammatory-mediated metabolic and cardiovascular comorbidities (Maxwell et al., 1999; Walmsley et al., 2014). Many of the free radicals generated by hypoxia destroy the redox system in vivo, triggering membrane lipid peroxidation, and destroying the network structure of the erythrocyte membrane, which directly reduces the deformation ability of erythrocytes (Tasali and Ip, 2008; Quercioli et al., 2010; Cavailles et al., 2013; Walmsley and Whyte, 2014; Pham et al., 2021). Hypoxia-related damage to erythrocytes is irreversible, so identifying additional extracts or drugs to antagonize hypoxia is necessary and meaningful.
Resveratrol (3,5,4’-trihydroxy-trans-stilbene, Res) is a polyphenol stilbene present in red wine, grapes, berries, and peanuts and also a plant antitoxin produced by plants to resist external stimuli, which is highly desirable (Renaud and De Lorgeril, 1992; Weiskirchen and Weiskirchen, 2016; Gorabi et al., 2021). As a nonflavonoid bioactive polyphenolic compound, resveratrol has great potential in disease prevention and treatment. First, resveratrol is a powerful antioxidant, and it has been shown that resveratrol has better antioxidant effects in individuals that are young and middle aged (Galiniak et al., 2019; Malaguarnera, 2019; Santos et al., 2023). In addition, many studies have confirmed that resveratrol has anti-inflammatory, antiaging, and antitumor effects; protects blood vessel walls and the heart; and inhibits low-density lipoprotein oxidation (Saiko et al., 2008; Fei et al., 2018; Li et al., 2019a; 2019b; Huang et al., 2020; Chen and Musa, 2021). Resveratrol can even improve obesity and the condition of being overweight (Durazzo et al., 2019; Tabrizi et al., 2020). These effects of resveratrol are exerted in blood circulation and are related to its concentration in the blood. Resveratrol relies on circulation in the blood to reach its target site. In recent years, several studies have focused on the effects of resveratrol on red blood cells (Suwalsky et al., 2015; Gallardo et al., 2019; Bardyn et al., 2021; Meleleo, 2021), and these effects have been studied from different perspectives. We have focused mainly on the effects and potential underlying mechanisms of resveratrol on the mechanical properties and membrane proteins of circulating red blood cells. Salidroside (SAL), the main medicinal component of salidroside rosea, has long been used as a medicinal material and is a promising antioxidant preparation. If resveratrol has the same effects as salidroside, additional options for clinical treatment could be provided.
The aim of this study was to investigate the mechanism of action of resveratrol on the cytoskeleton network of erythrocytes damaged by hypoxia. Based on the findings of previous studies, further elucidating the potential protective effects of resveratrol against hypoxia-induced damage to erythrocytes is necessary.
2 Materials and methods
This study was conducted in accordance with the Declaration of Helsinki, and the protocol was approved by the Ethics Committee of Chongqing University Cancer Hospital. All adult volunteers provided informed consent for inclusion before they participated in the study (protocol code CZLS2023261-A).
2.1 Blood samples
Venous blood samples, taken from the forearm vein of healthy adult volunteers, were treated with the anticoagulant sodium citrate (15 IU/mL). The collected blood was centrifuged at 800 × g for 3 min to separate the plasma and red blood cells. Then, the red blood cells were washed with phosphate-buffered saline (PBS), the supernatant was completely removed with a pipette, and the pellet was centrifuged 3 times for 2 min each time. Finally, 0.45 L/L hematocrit was retained, to which citrate phosphate dextrose solution was added. This solution can provide nutrients to the red blood cells and maintain the cellular osmotic pressure (main ingredients: citric acid, sodium citrate, glucose, and phosphate). The resulting mixture was a suspension of red blood cells.
2.2 Resveratrol concentration selection
2.2.1 Red blood cell morphology
Suspension of red blood cells were divided into three groups for this part of the experiment: a control group (normoxic conditions, 37°C, 120 min), a hypoxic group {in which a red blood cell suspension was placed in an anoxic chamber [95% N2, 5% CO2 (Boyd et al., 1980; Dillon and Waldrop, 1992; Bruhn et al., 2018), 37°C, 120 min]}, and a different concentration treatment group. In the treatment group, the red blood cell suspension was mixed with resveratrol so that the final concentrations of resveratrol were 10 μM, 40 μM, 70 μM, 100 μM, and 150 μM. Then, the erythrocytes were incubated with the resveratrol at 37°C for 60 min in normoxic conditions, followed by hypoxia treatment for 120 min.
Afterward, a 1 mL sample was taken from each group for centrifugation at 800 × g for 3 min, followed by washing with PBS (800 × g) 3 times for 2 min each time. Then, after the cells were diluted in PBS to a concentration of 104 cells/mL, 10 μL was placed on a slide and imaged using an Olympus IX71 microscope equipped with a 63/1.25 oil immersion objective and a CCD camera (Olympus, Tokyo, Japan).
Images of 50 erythrocytes from each experimental group were visually inspected under an electron microscope (4-5 photographs) to determine the proportions of discocytes and echinocytes.
2.2.2 Redox levels
To further evaluate the concentration of resveratrol that can protect red blood cells, we performed assays to determine the level of oxidoreductase and extent of membrane lipid peroxidation in red blood cells from each group using kits. The activity of catalase (CAT) was determined by measuring the absorbance at 405 nm by the ammonium molybdate method (Góth, 1991), activity of CAT was determined as follows: CAT (U/mL)=((ODcontrol–ODtreatment) *271*60)/0.1, 271 is constant, 60 is reaction time (s), and 0.1 is sample volume.
Superoxide dismutase (SOD) activity was determined by measuring the absorbance at 450 nm by the 2-(4-iodophenyl)-3-(4-nitrophenyl)-5-(2,4-disulfonic acid phenyl)-2H-tetrazolium salt, disodium salt (WST-1) method. Lipid peroxidation of the erythrocyte membrane was evaluated by measuring the content of malondialdehyde (MDA), the product of lipid peroxidation, via the thiobarbituric acid (TBA) method (Liu et al., 2022). TBA reactive material levels were estimated by measuring the absorbance at 532 nm. Activity of MDA was determined as follows: MDA (nmol/mL) = (ODtreatment-ODcontrol)/(ODstandard-ODblank)*10 nmol/mL/0.15 mL, 10 nmol/mL is concentration of standard, 0.15 mL is sample volume.
The methemoglobin (MetHb) content was used to assess the degree of cellular oxidation. Two samples from each experimental group. One sample was added to the hemoglobin test solution for measurement of the absorbance at 540 nm, and the other sample was added to the methemoglobin test solution to measure the absorbance at 630 nm and 602 nm. Activity of MetHb was determined as follows: MetHb =[(OD630 nm-0.14*OD602 nm)/OD602 nm*1.67] * OD540 nm*367.7, where 0.14,1.67 and 367.7 are constants determined by the kit company.
The calculations for all assays were performed according to manufacturer’s instructions of the kits provided by the Nanjing Jiancheng Institute of Biological Engineering. (http:Njjcbio.com/product.asp).
2.3 Effect of resveratrol on the rheological properties of erythrocytes
In a subsequent experiment, we established six experimental groups: the healthy red blood cells group (control), the hypoxia group (hypoxia), the healthy red blood cells add resveratrol group (C-Res), the healthy red blood cells add salidroside group (C-SAL), the hypoxic red blood cells add resveratrol group (H-Res), and the hypoxic red blood cells add salidroside group (H-SAL), with the salidroside group serving as the active control and the hypoxia group serving as the negative control. The working concentration of resveratrol and salidroside was 70 μM (purchased from Beijing Solarbio Science & Technology Co., Ltd.).
Blood is a non-Newtonian fluid, and the viscosity of blood at high shear rates is related to erythrocyte deformability, whereas at low shear rates, blood viscosity is related to erythrocyte aggregation (Shiga et al., 1990; Beris et al., 2021). Isolated plasma was added to washed red blood cells from each experimental group to simulate whole blood for whole blood viscosity testing (0.45 L/L hematocrit). The viscosity of each sample suspension was measured by an automatic hemorheology rapid detector (FASCO-5010DX, Chongqing Weiduo Technology Co., Ltd., China) to determine the deformability and aggregation of red blood cells. The hemorheometer was heated to and maintained at 37°C, after which the channel was cleaned. Samples from each group were then placed into tubes for whole blood viscosity determination. At the end of the test, the channel was cleaned again.
2.4 Influence on erythrocyte deformability
Atomic force microscopy (AFM; BRUKER, Dimension ICON010803, United States) was used to measure the Young’s modulus and surface roughness of the cells. Pretreated erythrocytes were resuspended in PBS and added to polylysine-treated glass slides. Peak force quantitative nanomechanical mapping (PFQNM) mode was selected for AFM calibration. Measurements were started after a single red blood cell was observed under the microscope using a soft-cone silicon nitrided cantilever with an elastic constant of 0.92 N/m (F), a probe angle of 18°, and a probe radius of 23.1 nm. In PFQNM mode, three kinds of images can be obtained: a high sensor, peak force error and DMT modulus. The Young’s modulus of the cells was determined in the DMT Modulus channel using Nanoscale analysis software. In addition, the 5 μm × 5 μm region of the cell was selected in the DMT Modulus channel to determine the surface roughness.
For each experiment, 30 cells from each group were randomly examined under a microscope.
AFM was performed based on the Sneddon model (Sneddon, 1965) formula:
where E is the elastic or Young’s modulus, v is the Poisson ratio (assumed to be 0.5), α is the opening angle and δ is the indentation depth. Here, F = 0.92 N/m.
The surface roughness was calculated with the following formula (https://www.bruker.com/):
where Rq is the mean square root roughness, N is the number of sampling points, and Z is the height of the ith sampling point.
2.5 Detection of erythrocyte membrane damage—FHb, MetHb, and MDA
For the detection of free hemoglobin (FHb) in the supernatants from different treatment groups, the absorbance at 510 nm was measured after different color development reactions. First, the relationship between the optical density and the concentration of the standard substance was determined. Then, 0.15 mL of supernatant was collected from each group, and 2.5 mL of chromogenic agent was added. In the control group, 0.15 mL of double distilled water and 2.5 mL of chromogenic agent were added. Each mixture was heated in a water bath at 37°C for 20 min, after which the optical density was measured at a wavelength of 510 nm. The free hemoglobin concentration was calculated according to the standard curve. Then FHb concentration was experimentally calculated according to: FHb (mg/L) = 126.03*(ODtreatment-ODblank) + 3.5041, 126.03, and 3.5041 were based on a standard curve.
The method for detecting MetHb and MDA were consistent with that described in Section 2.2.2.
All the parameters and calculation methods used were measured using kits provided by Nanjing Jiancheng Bioengineering Institute (Njjcbio.com/product.asp) according to the manufacturer’s instructions.
2.6 Eryptosis detection by flow cytometry
Differently treated erythrocytes were centrifuged at 800 × g for 5 min, after which the supernatant was discarded and the cells were collected and resuspended in PBS. Then, 1–5 × 105 cells were taken and centrifuged again at 800 × g for 5 min, and the supernatant was discarded. Cell survival was detected using an Annexin V-FITC apoptosis detection kit (China, http://www.solar.bio.com). Briefly, 500 μL of binding solution was added to the cellular precipitate and mixed gently. Then, 5 μL of Annexin V-FITC was added, and the mixture was incubated for 10 min at 25°C in the dark. The CytoFLEX assay was performed immediately (Beckman Coulter, United States).
2.7 Determination of erythrocyte membrane sulfhydryl and glutathione levels
Both the reduced glutathione (GSH) molecules in the erythrocytes and the sulfhydryl groups in the erythrocyte membrane proteins can react with 5,5'-dithiol-di-nitrobenzoic acid (DTNB), and the resulting compounds have a maximum absorption peak at 412 nm. The contents of GSH and sulfhydryl groups in membrane proteins can be measured via visible spectrophotometry (Aksenov and Markesbery, 2001).
2.8 Immunofluorescence and image analyses
Cells were fixed by mixing 4% paraformaldehyde and 0.01% glutaraldehyde at a 1:1 ratio and then washed with PBS at 1200 rpm 3 times for 2 min each time. Then, 0.01% Triton X-100 was used to permeabilize the red blood cell membranes, after which the cells were washed with PBS at 1200 rpm 3 times for 2 min each time. Bovine serum albumin (BSA) was used to block the other sites on the membrane so that subsequent antibodies could bind specifically. After the corresponding antibody was selected, the cells were incubated with mouse monoclonal spectrin antibody and rabbit monoclonal band 3 antibody (Abcam, UK, No. ab2808; Abcam, UK, No. ab108414) diluted 1:200 in 5% BSA for 2 h at room temperature. The cells were washed with PBS three times (two minutes each time). Then, the cells were incubated with secondary antibodies (Alexa Fluor 488 goat anti-mouse, No. A0428 and Alexa Fluor 488 goat anti-rabbit, No. A0423; Beyotime, China), diluted with 5% BSA at a 1:400 dilution, and washed three times with PBS. Finally, the cells were imaged to observe fluorescence using an Olympus IX71 microscope equipped with a 63/1.25 oil immersion objective and a CCD camera (Olympus, Tokyo, Japan).
2.9 Western blot analysis
Rinsed erythrocytes were placed in a cold lysis buffer mixture containing 1 mM MgCl2, 1 mM ethylenediaminetetraacetic acid sodium, 10 mM Tris-HCl, and 0.1 mM phenylmethylsulfonyl fluoride (pH 8.0) at 4°C for hemolysis. Erythrocyte membrane proteins were collected after treatment with lysis buffer and centrifugation at 12,000 rpm for 10 min, and protein concentrations were quantified by the Bradford method. The proteins were solubilized in SDS‒PAGE loading buffer with or without dithiothreitol (DTT) at a volume ratio of 4:1, and each mixture was incubated at 100°C for 10 min.
Clear bands were obtained by electrophoresis on a preconfigured polyacrylamide separation gel and a concentrated gel. The proteins were subsequently transferred from the gel to a polyvinylidene fluoride membrane and immunostained with primary antibodies (mouse monoclonal to spectrin, No. S3396, Sigma, Germany, diluted 1:500; rabbit monoclonal to band 3, No. ab108414, Abcam, UK, diluted 1:5000; rabbit monoclonal to β-actin, No. 380624, ZENBIO, China, diluted 1:5000; and rabbit monoclonal to band 3 phospho Y359 and phospho Y21, Abcam, UK, diluted 1:20,000 and 1:10,000. No. ab77236 and No. ab125070). The sections were incubated with anti-mouse (No. 701051) or anti-rabbit (No. 511203) secondary antibodies (ZENBIO, China) diluted 1:10,000, and the proteins were detected via chemiluminescence (Thermo Scientific, United States). Quantification of the immunocomplexes was conducted by lengthwise scanning densitometry using a gel analyzer image processing program (Azure Biosystem, United States).
2.10 Statistical analysis
The experimental results are expressed as the mean ± standard deviation. One-way ANOVA was used to compare more than three groups.
Origin 9.0 software was used for statistical analysis. Quantity One was used for gray analysis. FlowJo was used for cell eryptosis analysis.
3 Results
3.1 Selection of resveratrol concentration—erythrocyte morphology
When a red blood cell suspension was placed in a hypoxic environment, echinocytes (spiculated cells) appeared. To determine an appropriate treatment concentration of resveratrol, normal red blood cells were incubated with different concentrations of resveratrol (Figure 1A), and then, some of the cells were subjected to hypoxic conditions (Figure 1B) and observed under a light microscope to determine the resveratrol concentration that had a protective effect on red blood cell morphology.
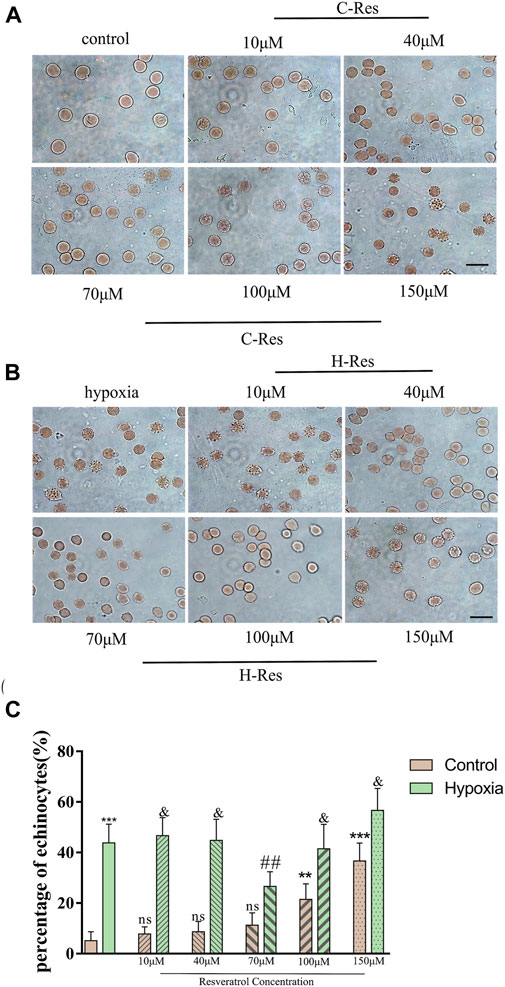
Figure 1. (A), Changes of erythrocyte morphology in different concentrations of resveratrol. (B), Morphological changes of erythrocytes after different concentrations of resveratrol were incubated and treated with hypoxia. Scale bar = 10 μm. (C), Percentage of echinocytes. n = 3, compared with the control group, **p < 0.01, ***p < 0.001, ns means not significant; compared with hypoxia group ##p < 0.01, & means not significant.
As shown in Figure 1C, when the resveratrol concentration was increased to 100 μM, the number of echinocytes increased to more than 20%, and at 150 μM resveratrol, 40% were echinocytes. Compared with the normal group, high concentrations of resveratrol (100 μM and 150 μM) were not conducive to protecting cell morphology (p < 0.01, p < 0.001). Compared with the hypoxia group, 70 μM resveratrol protected cell morphology (p < 0.01). Moreover, there were no significant differences between the low (10 μM and 40 μM) and high (100 μM and 150 μM) resveratrol concentration groups and the hypoxia group.
3.2 Selection of resveratrol concentration—effect on redox levels in red blood cells
To determine the optimal working concentration of resveratrol, the levels of CAT, SOD, MDA, and MetHb in normal and hypoxic erythrocytes incubated with resveratrol were measured.
Compared with the control group, the levels of CAT (Figure 2A) and SOD (Figure 2B) were significantly lower after hypoxia treatment (p < 0.001; p < 0.01), but the MDA (Figure 2C) and MetHb (Figure 2D) levels were higher (p < 0.01; p < 0.001). After incubating normal erythrocytes with different concentrations of resveratrol, the CAT content gradually decreased with increasing concentration (100 μM and 150 μM) and was significantly different from that in the control group (p < 0.05); however, the SOD content did not change significantly with increasing resveratrol concentration. With respect to the MDA content, when the concentration of resveratrol was increased to 150 μM, the MDA content increased and was significantly different from that in the control group (p < 0.05). When the concentration of resveratrol was 100 μM or 150 μM, the MetHb content was significantly higher than that in the control group (p < 0.05; p < 0.01).
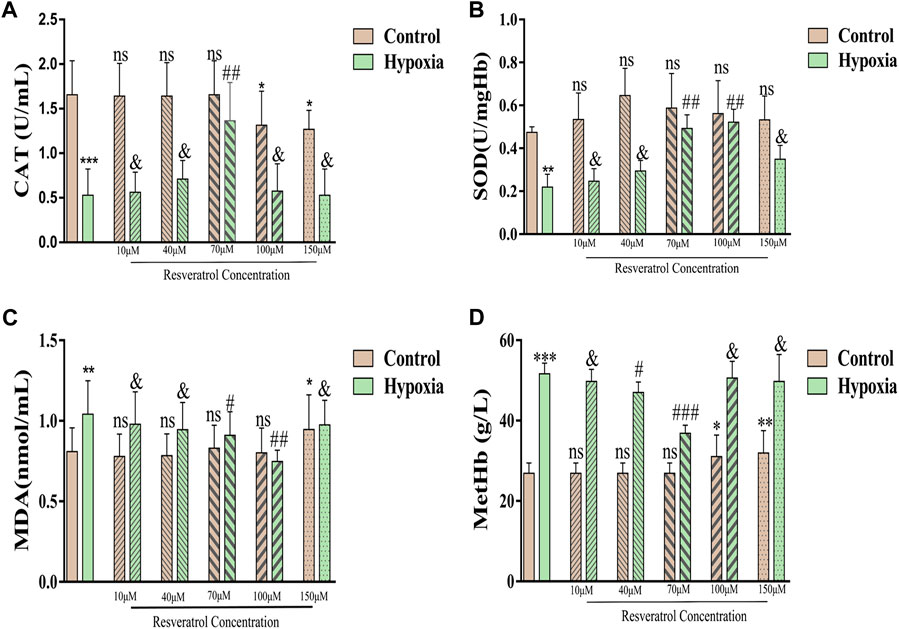
Figure 2. (A), Changes of CAT contents after incubation with different concentrations of resveratrol. Among them, the Y-axis in panel A represents CAT activity, defined as the amount of 1umol hydrogen peroxide catabolized per milliliter of serum per second as a unit of activity; (B), Changes of SOD content, the Y-axis is the SOD activity, which is the corresponding enzyme amount when the SOD inhibition rate reaches 50%; (C), Changes in MDA content; (D), Changes in MetHb content. Values are expressed as the mean ± SD. Compared with control group, **p < 0.01, ***p < 0.001, ns means not significant; Compared with hypoxia group, ##p < 0.01, & means not significant. n = 3.
After hypoxia treatment after incubation with 70 µM resveratrol, the levels of CAT and MetHb were significantly different from those in the hypoxia group (p < 0.01; p < 0.001). The levels of SOD and MDA after treatment with 70 μM and 100 μM resveratrol were significantly different from those in the hypoxia group (p < 0.01; p < 0.01; p < 0.05; p < 0.01).
Considering the changes in red blood cell morphology and CAT, SOD, MDA, and MetHb levels, we selected 70 μM resveratrol as the optimal concentration for use subsequent experiments.
3.3 Effect of resveratrol on erythrocyte aggregation and deformation
Whole-blood viscosity can reflect the aggregation and deformability of red blood cells. According to the results in Figure 3A, we found that the simulated whole-blood viscosity of the hypoxia group was significantly greater than that of the normal group under both high (p < 0.05), medium (p < 0.001), and low shear rate (p < 0.05) conditions. When resveratrol and salidroside were added, the whole-blood viscosity did not significantly change compared with that of the control group, and resveratrol achieved the same effect as salidroside.
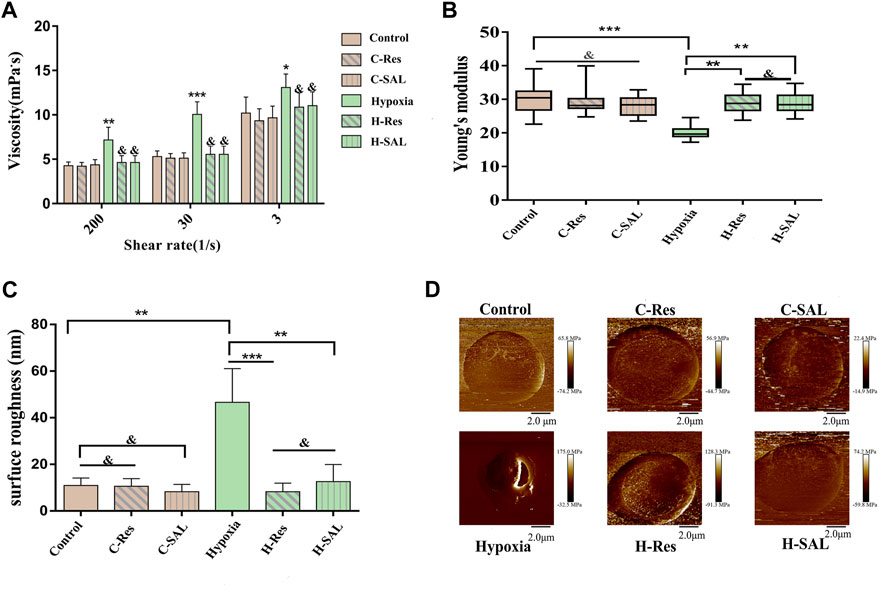
Figure 3. (A), The viscosity of erythrocyte suspension was measured at different shear forces to indirectly characterize the aggregation and deformability of erythrocyte. Compared with control group, *p < 0.05, **p < 0.01, and ***p < 0.001. Compared with the hypoxia group, & means there is no significant difference. (B), Changes in the Young’s modulus and (C) surface roughness of erythrocyte reflect the deformability of cells. surface roughness was measured over the 5 μm × 5 μm area. **p < 0.01, ***p < 0.001 and and means no significant difference. n = 3. (D), DMT modulus, representative images of individual cells in each group were selected.
To investigate the cellular mechanical properties of hypoxic erythrocytes after resveratrol treatment, 30 single cells from each sample were selected for AFM measurements, which were repeated three times. The whole experiment consisted of six independent replicate tests, and the resulting data collation is shown in Figure 3B. Neither the presence of resveratrol nor the presence of salidroside affected the mechanical properties of red blood cells. After hypoxia treatment, the Young’s modulus of the red blood cells decreased significantly (p < 0.001). However, after resveratrol or salidroside were added, the Young’s modulus increased (p < 0.01), and there was no obvious difference between them. The surface of the normal red blood cells was smooth. After hypoxia treatment, the surface roughness of the red blood cells increased, and after resveratrol and salidroside treatment, the surfaces of the red blood cells were smoother than those of the hypoxia group, as shown in Figure 3C.
Figure 3D presents the DMT modulus diagram, which shows representative images of single cells selected from each group.
3.4 Protection of the erythrocyte membrane by resveratrol after hypoxia
The levels of FHb, MetHb, and MDA were measured to investigate the effect of resveratrol on hypoxic injury in erythrocytes. As shown in Figure 4, the contents of FHb (Figure 4A), MetHb (Figure 4B) and MDA (Figure 4C) did not change significantly in control red blood cells after the addition of resveratrol or salidroside. After hypoxia, the FHb, MetHb and MDA levels increased (p < 0.01; p < 0.001; p < 0.001). In the presence of resveratrol and salidroside, the FHb, MetHb, and MDA contents were lower than those in hypoxia group.
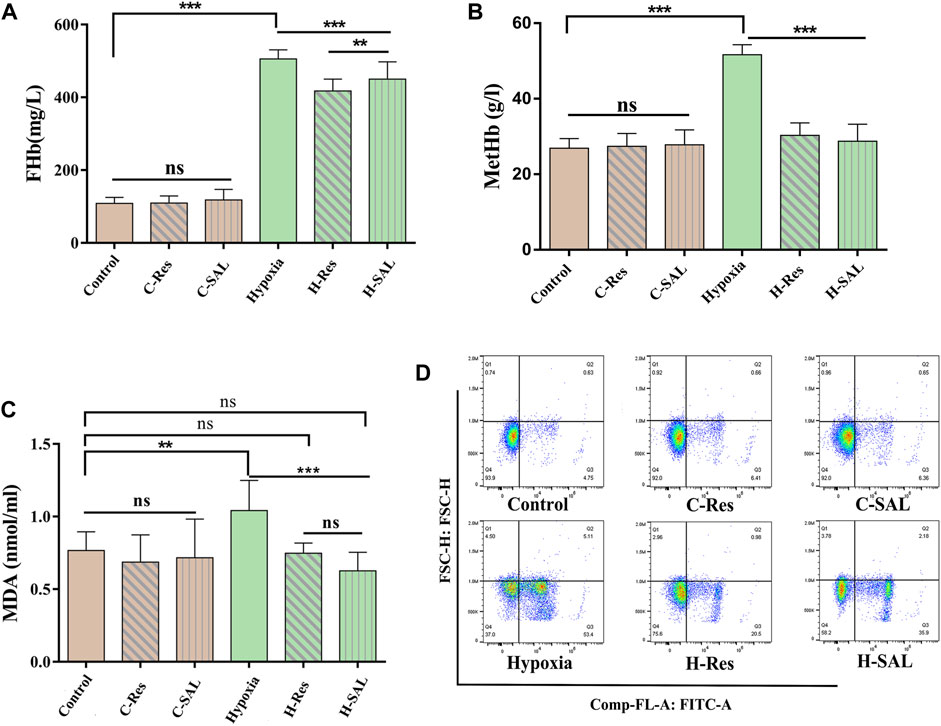
Figure 4. Erythrocyte membrane damage was measured by (A,B) free hemoglobin and methemoglobin content in erythrocyte suspensions, and (C) membrane lipid peroxidation levels. (D) Effect of resveratrol on eryptosis induced by hypoxic stimulation, n = 3. Values are expressed as the mean ± SD. **p < 0.01, ***p < 0.001 and ns means no significant difference.
Flow cytometry was used to analyze whether resveratrol could protect red blood cells from eryptosis. The results (Figure 4D) show that when normal red blood cells were incubated with resveratrol and salidroside, there was no significant change in the number of eryptotic cells. After hypoxia, the percentage of cells binding Annexin V increased from 6.12% to 63.01%. However, under hypoxic conditions, resveratrol and salidroside significantly reduced the percentage of cells binding Annexin V from 63.01% to 24.44% and 41.86%, respectively.
3.5 Effect of erythrocyte membrane proteins
Spectrin is the main skeletal component of the erythrocyte membrane that plays an important role in maintaining erythrocyte deformability. Immunofluorescence localization analysis (Figure 5A) showed that resveratrol and salidroside had no effect on the expression of the protein spectrin, although hypoxia decreased the average fluorescence intensity of the spectrin protein (Figure 5B; p < 0.01). After incubation with resveratrol, the fluorescence intensity of the cells was significantly greater than that in the hypoxia group (p < 0.05).
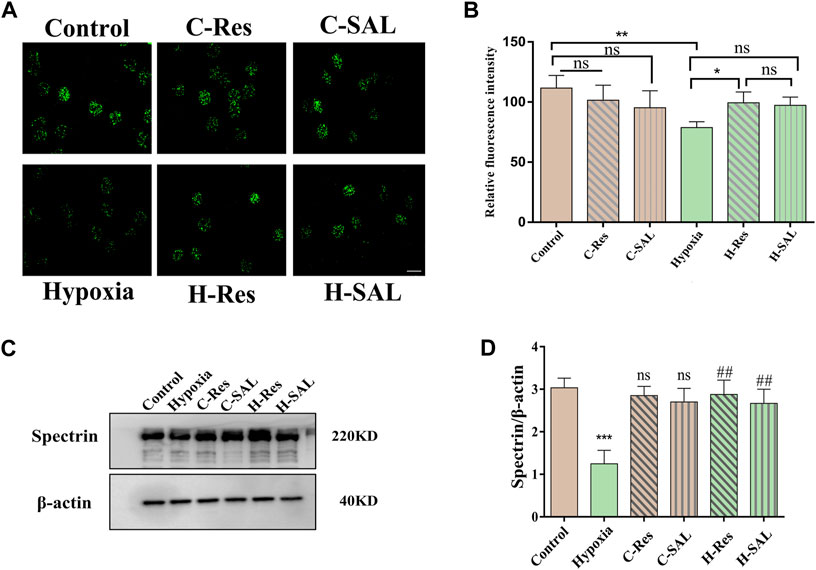
Figure 5. (A) Immunofluorescence staining of spectrin protein in different groups was performed and (B) the fluorescence intensity was analyzed, scale bar = 10 μm. (C) Western blotting was used to analyze the effect of resveratrol on spectrin protein after hypoxic stress in the absence of DTT. (D) Spectrin western blot density was quantified, and β-actin was used as an internal reference. n = 3. Compared with the control group, ***p < 0.001, ns means no significant difference. Compared with the hypoxia group, ##p < 0.01.
Western blotting confirmed the effects of hypoxia and resveratrol supplementation on the spectrin protein level (Figure 5C). Hypoxia led to a decrease in the spectrin protein content (Figure 5D; p < 0.001), but after the addition of resveratrol and salidroside to the hypoxia treatment group, the protein content of spectrin was significantly greater than that in the hypoxia group (Figure 5D; p < 0.01; p < 0.01), indicating that resveratrol could resist the damage to spectrin caused by hypoxic conditions.
3.6 Effect of resveratrol on erythrocyte membrane -SH levels
Compared with those in the control group, the intracellular GSH and membrane-SH contents in the hypoxia group were significantly lower (Figure 6A, p < 0.01; Figure 6B, p < 0.001), but the GSH and -SH contents were significantly greater in the presence of resveratrol (p < 0.01; p < 0.01) and salidroside (p < 0.01; p < 0.01). After hypoxia treatment, there was no significant difference in the GSH or -SH content between the groups incubated with resveratrol and salidroside.
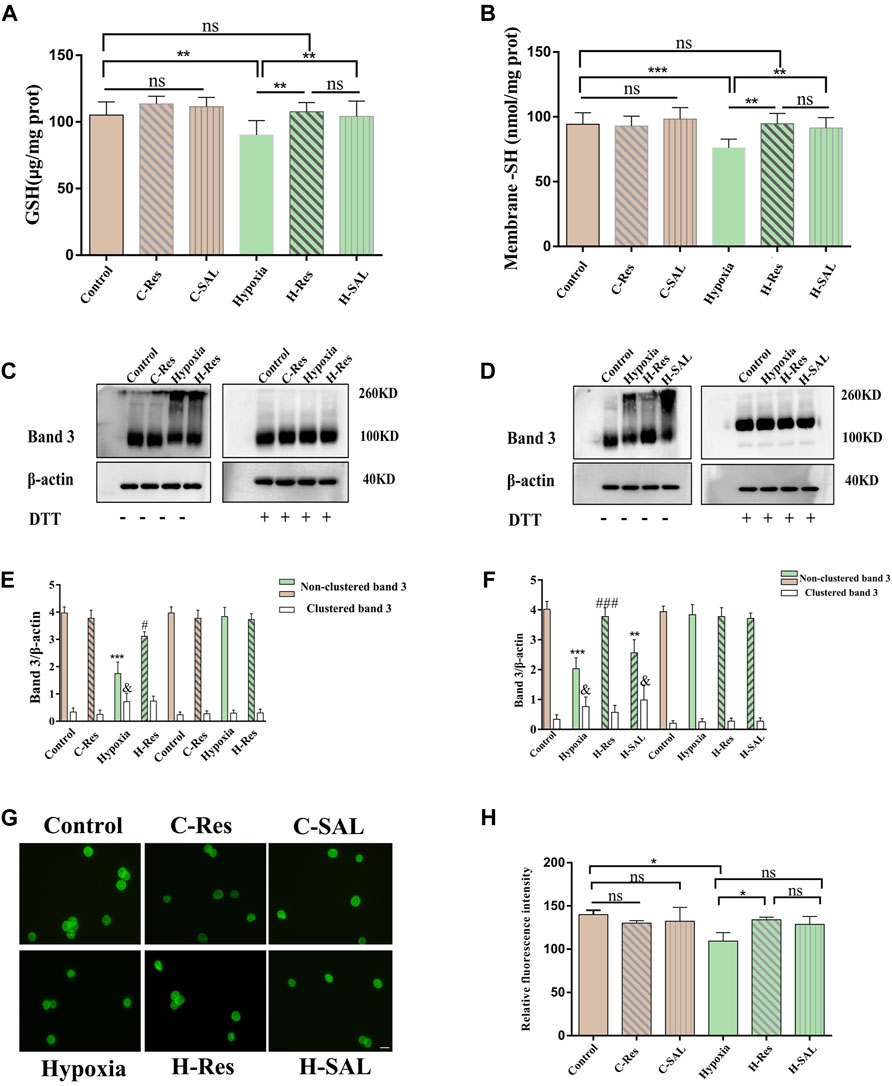
Figure 6. Protection of thiol groups by resveratrol (A) erythrocyte glutathione and (B) changes in protein thiol content on membranes. Values are expressed as the mean ± SD. **p < 0.01, ***p < 0.001 and ns means no significant difference. (C,D) The effect of resveratrol on the crosslinking sum of hypoxia stress-related band 3 was analyzed by western blotting in the presence or absence of DTT, (E,F) The band 3 cluster immunoblot density was quantified, and β-actin was used as an internal reference. Values are expressed as the mean ± SD. Compared with the unclustered control group, ***p < 0.001; Compared with the unclustered hypoxia group, ###p < 0.001; Compared with the clustered control group, & p < 0.05. (G) Cellular immunofluorescence staining for band 3 protein. Scale bar = 10 μm. (H) The average fluorescence intensity of immunofluorescence staining of band 3 protein in different groups was analyzed, *p < 0.05, and ns means no significant difference. n = 3.
Resveratrol may regulate the deformability of hypoxic erythrocytes under hypoxic conditions by regulating the cross linking of band 3. To explore this possibility, we examined the status of band 3 after incubation under hypoxic conditions or with resveratrol in the presence or absence of DTT. Figure 6C–F shows that the band 3 protein content in the hypoxia group decreased significantly in the absence of DTT (p < 0.05, p < 0.001). Additionally, this protein was partially oxidized to a high polymer (p < 0.05, p < 0.05) but reduced back to a band between 90 and 100 kDa in erythrocytes after incubation with DTT. After incubation with resveratrol in the absence of DTT, the band 3 protein content was significantly greater than that in the hypoxia group (p < 0.05; p < 0.001) the polymers were reduced or absent with no significant difference compared with the crosslinked band 3 in the control group (Figures 6C–F). Hypoxia-associated oxidative stress leads to damage caused by the free sulfhydryl groups in membrane proteins, thereby inducing the oxidation of band 3 proteins through the formation of reducible intermolecular and/or intramolecular disulfide bonds.
Immunofluorescence localization analysis of the membrane proteins was also performed (Figures 6G, H). Compared with those in the control group, the relative fluorescence intensity of the band 3 protein in normal erythrocytes incubated with resveratrol and salidroside did not significantly change. After hypoxia, the relative fluorescence intensity of the band 3 protein on the erythrocyte membrane was significantly lower than that in the control group (p < 0.05; p < 0.01). After the addition of resveratrol, the relative fluorescence intensity of the band 3 protein was significantly greater than that in the hypoxia group (p < 0.05; p < 0.05). After the addition of salidroside, the relative fluorescence intensities of band 3 and the contractile protein were not significantly different from those in the hypoxia group. There was no significant difference between the resveratrol group and the salidroside group after hypoxia treatment.
3.7 Analysis of band 3 protein tyrosine phosphorylation
Western blot analysis of tyrosine phosphorylation at positions 359 and 21 in the band 3 protein (Figure 7A) showed that band 3 phosphorylation was induced after erythrocytes were subjected to hypoxia (Figures 7B, C; p < 0.001; p < 0.001). After resveratrol or salidroside were added, compared with that in the hypoxia group, the phosphorylation of band 3 at position 21 was decreased (Figure 7B; p < 0.001, p < 0.001). However, after the addition of resveratrol or salidroside, there was no significant difference in phosphorylation at position 359 of the band 3 protein compared with that in the hypoxia group (Figure 7C).
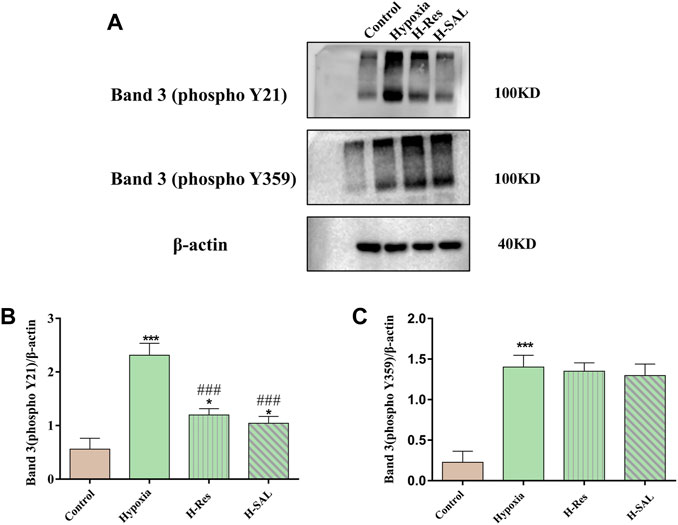
Figure 7. (A) Western blot was used to detect the phosphorylation of tyrosine 21 and 359 in band 3, (B,C), and the western blot band was quantified using actin as an internal reference. Compared with the control group, *p < 0.05, ***p < 0.001. Compared with the hypoxia group, ###p < 0.001. n = 3.
4 Discussion
Resveratrol protect the morphological integrity of red blood cells and prevent damage to the red blood cell membrane, the oxidation of membrane sulfhydryl groups, damage to the protein spectrin, and the phosphorylation of the protein band 3 under hypoxia. All of these are important events involved in the stabilization of the mechanical properties of the membrane.
The first step of this study was to test different concentrations of resveratrol (from 10 μM to 150 μM) to rule out the possible effect of high dose of resveratrol on red blood cells. In this experiment, the optimal working concentration of resveratrol was evaluated from four aspects: red blood cell morphology, red blood cell REDOX level (CAT and SOD), red blood cell membrane lipid peroxidation level (MDA) and MetHb. As shown in Figure 1A, increased concentrations of resveratrol above 100 μM resulted in the appearance of acanthocytes; Conversely, it was shown in 1b that resveratrol at 40–70 μM had the best protective effect on red blood cell morphology caused by hypoxia. Similarly, by examining the response of REDOX level in normal erythrocytes to different concentrations of resveratrol in Figure 2, we found that the content of CAT decreased at 100 μM, while the content of CAT in hypoxic erythrocytes showed a trend of first increasing and then decreasing, with the highest content of CAT at 70 μM. In addition, there was no significant change in SOD content in normal erythrocytes with the increase of resveratrol concentration, but the SOD content in hypoxic erythrocytes increased with the increase of resveratrol concentration, and the SOD content was the highest at 70 μM and 100 μM. In the experiments with MDA and MetHb, the same pattern emerged. This suggests that excessive antioxidants may exhibit pro-oxidative (Giordano et al., 2020) and pro-membrane lipid peroxidation effects. Based on these findings, we chose 70 μM resveratrol to investigate the possible mechanism in the hypoxia model, which is also close to the concentration selected by other investigators in different disease models (Horn et al., 2007; Saiko et al., 2008; Komorowska et al., 2023; Ei et al., 2024; Fu et al., 2024).
The results of atomic force microscopy showed that resveratrol had a statistically significant effect on the increase of Young’s modulus of red blood cells induced by hypoxia in Figure 3. In addition, the blood viscosity increased at a high shear rate after hypoxia as determined by hemorheology, which clinically indicates that the deformability of red blood cells was poor. Moreover, viscosity also increased at a low shear rate, suggesting that the red blood cells were aggregated. These results all suggest that resveratrol is involved in hypoxia-induced changes in erythrocyte deformability. We further found that resveratrol could decrease the expression of free hemoglobin and methemoglobin in the cytoplasm of hypoxic erythrocytes, protect the integrity of erythrocyte membrane, and reduce the expression of MDA in hypoxic erythrocytes. This also proves that resveratrol protects erythrocyte membrane integrity and inhibits membrane lipid peroxidation. The structure, composition, and fluidity of the erythrocyte membrane affect erythrocyte deformability. Erythrocytes are among the most vulnerable cells to oxidative stress. Unsaturated fatty acids on the cell membrane undergo oxidative attacks, leading to membrane lipid peroxidation and decreased membrane fluidity (Mohandas and Chasis, 1993; Ranjekar et al., 2003). Human erythrocytes are rich in sulfhydryl groups, and the importance of the -SH groups on the erythrocyte membrane in the overall cellular redox balance has been emphasized. Hypoxia results in increased sulfhydryl oxidation, which is inhibited by resveratrol, indicating that resveratrol exerts a very rapid protective effect on the oxidation of membrane sulfhydryl groups (Pandey and Rizvi, 2010b). In previous studies, resveratrol was shown to stimulate the production of heme oxygenase-1 (HO-1), whose degradation promotes the oxidation of oxidized heme to produce bilirubin, thereby eliminating oxygen free radicals (Doré, 2002; Zhuang et al., 2003; Juan et al., 2005) and protecting the body from the adverse effects of various environmental toxins (Latruffe et al., 2015; Vestergaard and Ingmer, 2019). Resveratrol can effectively reduce the expression of inflammatory factors such as interleukin-6 (IL-6), which are activated by hypoxia, and activate the production of the deacetylase Sirt1 (Gomes et al., 2018).
Changes in erythrocyte membrane function during hypoxia are associated with changes in membrane biophysical properties (George et al., 2010; 2013). Spectrin is a major component of the cytoskeleton, and the spectrin-based membrane skeleton essentially maintains the integrity and mechanical properties of the cell membrane and the cytoskeletal network that supports cell shape. In our experiments, hypoxia-induced spectrin degradation led to destabilization of the membrane skeleton network, altered discocyte morphology, and decreased cell deformability. In addition, our study showed that crosslinked band 3 forms oligomer protein complexes under hypoxic conditions. This high-molecular-weight protein is involved in hypoxia-associated oxidative stress, leading to damage by free sulfhydryl groups in membrane proteins during hypoxia, thereby inducing the oxidation of the band 3 protein through the formation of reversible intermolecular and/or intramolecular disulfide bonds. No high-molecular-weight oligomeric proteins were found after resveratrol treatment, suggesting that resveratrol inhibits the oxidation of free sulfhydryl groups. The band 3 protein cluster was shown to be a recognition site generated during erythrocyte decay (Arese et al., 2005), which induces recognition and clearance from the circulation by macrophages. Sulfhydryl groups on the erythrocyte membrane are closely related to erythrocyte viscoelasticity and osmotic fragility (Pandey and Rizvi, 2010b). Combined with the deformability analysis, the protection of hypoxia-induced protein aggregation by resveratrol may be one of the mechanisms by which resveratrol improves red blood cell deformability.
The function of the band 3 protein on the erythrocyte membrane is regulated by protein tyrosine phosphatases (PTP), spleen tyrosine kinase (syk) and Src family tyrosine kinase (Lyn), Y-phosphorylation/dephosphorylation is likely involved in the regulation of several erythrocyte functions (Minetti et al., 1998, p. 3; Brunati et al., 2000) Tyrosine phosphorylation of band 3 is induced by many stimuli, such as aging, malaria, and oxidative stress (Ferru et al., 2011, p. 3; Spinelli et al., 2023). In the present study, hypoxia induced the formation of disulfide bonds from the free sulfhydryl groups on the erythrocyte membrane. The formation of disulfide bonds attenuated the dephosphorylation of PTP, and in this way, a series of acidic amino acids in syk and lyn surrounded the band 3 protein and removed most of it from the membrane skeleton. Previous studies have shown that phosphorylation of band 3 on the erythrocyte membrane reduces binding to anchor proteins (Pantaleo et al., 2009; Ferru et al., 2011, p. 3), increasing the mobility of band 3 and its phosphorylation and promoting the dissociation of band 3 from anchor proteins and its aggregation, which seriously damages the stability of the skeleton network and reduces the deformability of erythrocytes. Thus, band 3 tyrosine phosphorylation promotes band 3 crosslinking, leading to reduced erythrocyte aggregation and deformability. Due to the dissociation of ankyrin from band 3 by the peroxidation of membrane lipids and tyrosine phosphorylation of band 3, the immobile band 3 becomes mobile and is prone to cluster formation. Resveratrol may act as a regulatory fragment to alter the affinity between the anchoring protein and band 3 by changing the phosphorylation state of band 3 in a hypoxic environment.
In vitro and in vivo phosphorylation of band 3 in human erythrocytes is catalysed by syk and Src-associated tyrosine kinases, lyn acts on tyrosines located in the thiol (SH)-binding domain of the band 3 protein, and tyr21 and tyr359 are the main binding sites for syk and lyn, respectively (Yannoukakos et al., 1991; Brunati et al., 2000). Bands were analyzed by immunoblotting, under hypoxic conditions, the sulfhydryl group of the band 3 protein is oxidized, leading to phosphorylation of tyr21 and tyr359. Phosphorylation of tyr21 causes the band 3 protein to undergo a change in conformation, which further promotes tyr359 phosphorylation. Resveratrol attenuates the degree of phosphorylation of the band 3 protein by protecting its molecular structural stability and membrane sulfhydryl groups from oxidative attack, especially by protecting tyr21. Reduced phosphorylation associated with syk and lyn by resveratrol is consistent with previous findings (Kotha et al., 2006; Chung et al., 2019; Lewis et al., 2024).
Data availability statement
The original contributions presented in the study are included in the article/Supplementary Material, further inquiries can be directed to the corresponding author.
Ethics statement
The studies involving humans were approved by the Ethics Committee of Chongqing University Cancer Hospital. The studies were conducted in accordance with the local legislation and institutional requirements. The participants provided their written informed consent to participate in this study.
Author contributions
QY: Conceptualization, Methodology, Resources, Supervision, Validation, Visualization, Writing–original draft, Writing–review and editing. DC: Formal Analysis, Software, Writing–original draft. CL: Investigation, Resources, Software, Validation, Writing–original draft. RL: Formal Analysis, Investigation, Resources, Software, Writing–original draft. XW: Funding acquisition, Project administration, Supervision, Writing–original draft, Writing–review and editing.
Funding
The author(s) declare that financial support was received for the research, authorship, and/or publication of this article. This research was funded by grants from the National Natural Science Foundation of China (grant numbers 12172071 and 11572065). The APC was funded by 12172071.
Conflict of interest
The authors declare that the research was conducted in the absence of any commercial or financial relationships that could be construed as a potential conflict of interest.
Publisher’s note
All claims expressed in this article are solely those of the authors and do not necessarily represent those of their affiliated organizations, or those of the publisher, the editors and the reviewers. Any product that may be evaluated in this article, or claim that may be made by its manufacturer, is not guaranteed or endorsed by the publisher.
References
Aksenov M. Y., Markesbery W. R. (2001). Changes in thiol content and expression of glutathione redox system genes in the hippocampus and cerebellum in Alzheimer’s disease. Neurosci. Lett. 302, 141–145. doi:10.1016/S0304-3940(01)01636-6
Arese P., Turrini F., Schwarzer E. (2005). Band 3/Complement-mediated recognition and removal of normally senescent and pathological human erythrocytes. Cell Physiol. Biochem. 16, 133–146. doi:10.1159/000089839
Badior K. E., Casey J. R. (2021). Large conformational dynamics in Band 3 protein: significance for erythrocyte senescence signalling. Biochimica Biophysica Acta (BBA) - Biomembr. 1863, 183678. doi:10.1016/j.bbamem.2021.183678
Bardyn M., Allard J., Crettaz D., Rappaz B., Turcatti G., Tissot J.-D., et al. (2021). Image- and fluorescence-based test shows oxidant-dependent damages in red blood cells and enables screening of potential protective molecules. IJMS 22, 4293. doi:10.3390/ijms22084293
Barshtein G., Gural A., Zelig O., Arbell D., Yedgar S. (2020). Unit-to-unit variability in the deformability of red blood cells. Transfus. Apher. Sci. 59, 102876. doi:10.1016/j.transci.2020.102876
Barshtein G., Pajic-Lijakovic I., Gural A. (2021). Deformability of stored red blood cells. Front. Physiol. 12, 722896. doi:10.3389/fphys.2021.722896
Beris A. N., Horner J. S., Jariwala S., Armstrong M. J., Wagner N. J. (2021). Recent advances in blood rheology: a review. Soft Matter 17, 10591–10613. doi:10.1039/D1SM01212F
Boyd R. L., Clark C. H., Mcdaniel G. R. (1980). Chicken oxygen dissociation curve by dynamic tonometry. Poult. Sci. 59, 2782–2786. doi:10.3382/ps.0592782
Bruhn P. J., Nikolian V. C., Halaweish I., Chang Z., Sillesen M., Liu B., et al. (2018). Tubastatin A prevents hemorrhage-induced endothelial barrier dysfunction. J. Trauma Acute Care Surg. 84, 386–392. doi:10.1097/TA.0000000000001753
Brunati A. M., Bordin L., Clari G., James P., Quadroni M., Baritono E., et al. (2000). Sequential phosphorylation of protein band 3 by Syk and Lyn tyrosine kinases in intact human erythrocytes: identification of primary and secondary phosphorylation sites. Blood 96, 1550–1557. doi:10.1182/blood.V96.4.1550
Caimi G., Carlisi M., Montana M., Gallà E., Lo Presti R., Hopps E., et al. (2018). Erythrocyte deformability and hemorheological profile in multiple myeloma. Chem. 68, 25–34. doi:10.3233/CH-170267
Carelli-Alinovi C., Dinarelli S., Sampaolese B., Misiti F., Girasole M. (2019). Morphological changes induced in erythrocyte by amyloid beta peptide and glucose depletion: a combined atomic force microscopy and biochemical study. Biochimica Biophysica Acta (BBA) - Biomembr. 1861, 236–244. doi:10.1016/j.bbamem.2018.07.009
Carelli-Alinovi C., Giardina B., Misiti F. (2015). Amyloid beta peptide (1–42)-mediated antioxidant imbalance is associated with activation of protein kinase C in red blood cells. Cell Biochem. Funct. 33, 196–201. doi:10.1002/cbf.3103
Cavailles A., Brinchault-Rabin G., Dixmier A., Goupil F., Gut-Gobert C., Marchand-Adam S., et al. (2013). Comorbidities of COPD. Eur. Respir. Rev. 22, 454–475. doi:10.1183/09059180.00008612
Chen L., Musa A. E. (2021). Boosting immune system against cancer by resveratrol. Phytotherapy Res. 35, 5514–5526. doi:10.1002/ptr.7189
Chung Y.-H., Kim H. Y., Yoon B. R., Kang Y. J., Lee W.-W. (2019). Suppression of Syk activation by resveratrol inhibits MSU crystal-induced inflammation in human monocytes. J. Mol. Med. 97, 369–383. doi:10.1007/s00109-018-01736-y
Diez-Silva M., Dao M., Han J., Lim C.-T., Suresh S. (2010). Shape and biomechanical characteristics of human red blood cells in health and disease. MRS Bull. 35, 382–388. doi:10.1557/mrs2010.571
Dillon G. H., Waldrop T. G. (1992). In vitro responses of caudal hypothalamic neurons to hypoxia and hypercapnia. Neuroscience 51, 941–950. doi:10.1016/0306-4522(92)90531-6
Doré S. (2002). Decreased activity of the antioxidant heme oxygenase enzyme: implications in ischemia and in Alzheimer’s disease. Free Radic. Biol. Med. 32, 1276–1282. doi:10.1016/s0891-5849(02)00805-5
Durazzo A., Lucarini M., Souto E. B., Cicala C., Caiazzo E., Izzo A. A., et al. (2019). Polyphenols: a concise overview on the chemistry, occurrence, and human health. Phytotherapy Res. 33, 2221–2243. doi:10.1002/ptr.6419
Ei Z. Z., Srithawirat T., Chunhacha P., Chaotham C., Arunmanee W., Phookphan P., et al. (2024). Resveratrol shows potent senescence reversal in experimental cellular models of particular matter 2.5-induced cellular senescence in human dermal papilla cells. Vivo 38, 665–673. doi:10.21873/invivo.13487
Fei Q., Kent D., Botello-Smith W. M., Nur F., Nur S., Alsamarah A., et al. (2018). Molecular mechanism of resveratrol’s lipid membrane protection. Sci. Rep. 8, 1587. doi:10.1038/s41598-017-18943-1
Ferru E., Giger K., Pantaleo A., Campanella E., Grey J., Ritchie K., et al. (2011). Regulation of membrane-cytoskeletal interactions by tyrosine phosphorylation of erythrocyte band 3. Blood 117, 5998–6006. doi:10.1182/blood-2010-11-317024
Fu Y., Zhang L., Lin Y., Zhao X., Chen H., Zhong Y., et al. (2024). Unveiling the antibacterial mechanism of resveratrol against Aeromonas hydrophila through proteomics analysis. Front. Cell Infect. Microbiol. 14, 1378094. doi:10.3389/fcimb.2024.1378094
Galiniak S., Aebisher D., Bartusik-Aebisher D. (2019). Health benefits of resveratrol administration. Acta Biochim. Pol. 66, 13–21. doi:10.18388/abp.2018_2749
Gallardo M. J., Suwalsky M., Ramírez D., Tapia J., Sepulveda B. (2019). Antioxidant effect of resveratrol in single red blood cells measured by thermal fluctuation spectroscopy. Archives Biochem. Biophysics 665, 30–35. doi:10.1016/j.abb.2019.02.011
George A., Pushkaran S., Konstantinidis D. G., Koochaki S., Malik P., Mohandas N., et al. (2013). Erythrocyte NADPH oxidase activity modulated by Rac GTPases, PKC, and plasma cytokines contributes to oxidative stress in sickle cell disease. Blood 121, 2099–2107. doi:10.1182/blood-2012-07-441188
George A., Pushkaran S., Li L., An X., Zheng Y., Mohandas N., et al. (2010). Altered phosphorylation of cytoskeleton proteins in sickle red blood cells: the role of protein kinase C, Rac GTPases, and reactive oxygen species. Blood Cells, Mol. Dis. 45, 41–45. doi:10.1016/j.bcmd.2010.02.006
Giordano M. E., Caricato R., Lionetto M. G. (2020). Concentration dependence of the antioxidant and prooxidant activity of trolox in HeLa cells: involvement in the induction of apoptotic volume decrease. Antioxidants 9, 1058. doi:10.3390/antiox9111058
Gomes B. A. Q., Silva J. P. B., Romeiro C. F. R., Dos Santos S. M., Rodrigues C. A., Gonçalves P. R., et al. (2018). Neuroprotective mechanisms of resveratrol in alzheimer’s disease: role of SIRT1. Oxidative Med. Cell. Longev. 2018, 8152373–8152415. doi:10.1155/2018/8152373
Gorabi A. M., Aslani S., Imani D., Razi B., Sathyapalan T., Sahebkar A. (2021). Effect of resveratrol on C-reactive protein: an updated meta-analysis of randomized controlled trials. Phytotherapy Res. 35, 6754–6767. doi:10.1002/ptr.7262
Góth L. (1991). A simple method for determination of serum catalase activity and revision of reference range. Clin. Chim. Acta 196, 143–151. doi:10.1016/0009-8981(91)90067-M
Govekar R., Zingde S. (2001). Protein kinase C isoforms in human erythrocytes. Ann. Hematol. 80, 531–534. doi:10.1007/s002770100352
Himbert S., Rheinstädter M. C. (2022). Structural and mechanical properties of the red blood cell’s cytoplasmic membrane seen through the lens of biophysics. Front. Physiol. 13, 953257. doi:10.3389/fphys.2022.953257
Horn T. L., Cwik M. J., Morrissey R. L., Kapetanovic I., Crowell J. A., Booth T. D., et al. (2007). Oncogenicity evaluation of resveratrol in p53(+/-) (p53 knockout) mice. Food Chem. Toxicol. 45, 55–63. doi:10.1016/j.fct.2006.07.015
Huang T.-Y., Yu C.-P., Hsieh Y.-W., Lin S.-P., Hou Y.-C. (2020). Resveratrol stereoselectively affected (±)warfarin pharmacokinetics and enhanced the anticoagulation effect. Sci. Rep. 10, 15910. doi:10.1038/s41598-020-72694-0
Huisjes R., Bogdanova A., Van Solinge W. W., Schiffelers R. M., Kaestner L., Van Wijk R. (2018). Squeezing for life – properties of red blood cell deformability. Front. Physiol. 9, 656. doi:10.3389/fphys.2018.00656
Jasenovec T., Radosinska D., Celusakova H., Filcikova D., Babinska K., Ostatnikova D., et al. (2019). Erythrocyte deformability in children with autism spectrum disorder: correlation with clinical features. Physiol. Res. 68, S307–S313. doi:10.33549/physiolres.934349
Juan S.-H., Cheng T.-H., Lin H.-C., Chu Y.-L., Lee W.-S. (2005). Mechanism of concentration-dependent induction of heme oxygenase-1 by resveratrol in human aortic smooth muscle cells. Biochem. Pharmacol. 69, 41–48. doi:10.1016/j.bcp.2004.09.015
Karafin M. S., Field J. J., Ilich A., Li L., Qaquish B. F., Shevkoplyas S. S., et al. (2023). Hypoxic storage of donor red cells preserves deformability after exposure to plasma from adults with sickle cell disease. Transfusion 63, 193–202. doi:10.1111/trf.17163
Koch C. G., Duncan A. I., Figueroa P., Dai L., Sessler D. I., Frank S. M., et al. (2019). Real age: red blood cell aging during storage. Ann. Thorac. Surg. 107, 973–980. doi:10.1016/j.athoracsur.2018.08.073
Komorowska J., Wątroba M., Bednarzak M., Grabowska A. D., Szukiewicz D. (2023). The role of glucose concentration and resveratrol in modulating neuroinflammatory cytokines: insights from an in vitro blood–brain barrier model. Med. Sci. Monit. 29, e941044. doi:10.12659/MSM.941044
Kotha A., Sekharam M., Cilenti L., Siddiquee K., Khaled A., Zervos A. S., et al. (2006). Resveratrol inhibits Src and Stat3 signaling and induces the apoptosis of malignant cells containing activated Stat3 protein. Mol. Cancer Ther. 5, 621–629. doi:10.1158/1535-7163.MCT-05-0268
Kriebardis A. G. (2023). Benefits of hypoxic storage of red blood cells. Blood Transfus. 21, 1–2. doi:10.2450/2023.0229-22
Latruffe N., Lançon A., Frazzi R., Aires V., Delmas D., Michaille J.-J., et al. (2015). Exploring new ways of regulation by resveratrol involving miRNAs, with emphasis on inflammation: MicroRNA-dependent anti-inflammatory effect of resveratrol. Ann. N.Y. Acad. Sci. 1348, 97–106. doi:10.1111/nyas.12819
Lewis A. J., Richards A. C., Mendez A. A., Dhakal B. K., Jones T. A., Sundsbak J. L., et al. (2024). Plant phenolics inhibit focal adhesion kinase and suppress host cell invasion by uropathogenic Escherichia coli. Infect. Immun., e0008024. doi:10.1128/iai.00080-24
Li H., Ou G., He Y., Ren L., Yang X., Zeng M. (2019a). Resveratrol attenuates the MSU crystal-induced inflammatory response through the inhibition of TAK1 activity. Int. Immunopharmacol. 67, 62–68. doi:10.1016/j.intimp.2018.12.004
Li H., Xia N., Hasselwander S., Daiber A. (2019b). Resveratrol and vascular function. IJMS 20, 2155. doi:10.3390/ijms20092155
Liu J., Liao H., Chen Y., Zhu H., Li X., Liu J., et al. (2022). Resveratrol inhibits oxidative stress and regulates M1/M2-type polarization of microglia via mediation of the nrf2/shh signaling cascade after OGD/R injury in vitro. J. Pers. Med. 12, 2087. doi:10.3390/jpm12122087
Lopes C. S., Pronto-Laborinho A. C., Conceição V. A., Freitas T., Matias G. L., Gromicho M., et al. (2023). Erythrocytes’ surface properties and stiffness predict survival and functional decline in ALS patients. BioFactors. biof.2030. doi:10.1002/biof.2030
Lux S. E. (2016). Anatomy of the red cell membrane skeleton: unanswered questions. Blood 127, 187–199. doi:10.1182/blood-2014-12-512772
Malaguarnera (2019). Influence of resveratrol on the immune response. Nutrients 11, 946. doi:10.3390/nu11050946
Maxwell P. H., Wiesener M. S., Chang G.-W., Clifford S. C., Vaux E. C., Cockman M. E., et al. (1999). The tumour suppressor protein VHL targets hypoxia-inducible factors for oxygen-dependent proteolysis. Nature 399, 271–275. doi:10.1038/20459
Meleleo D. (2021). Study of resveratrol’s interaction with planar lipid models: insights into its location in lipid bilayers. Membranes 11, 132. doi:10.3390/membranes11020132
Minetti G., Seppi C., Ciana A., Balduini C., Low P. S., Brovelli A. (1998). Characterization of the hypertonically induced tyrosine phosphorylation of erythrocyte band 3. Biochem. J. 335, 305–311. doi:10.1042/bj3350305
Mohandas N., Chasis J. A. (1993). Red blood cell deformability, membrane material properties and shape: regulation by transmembrane, skeletal and cytosolic proteins and lipids. Semin. Hematol. 30, 171–192.
Mohandas N., Gallagher P. G. (2008). Red cell membrane: past, present, and future. Blood 112, 3939–3948. doi:10.1182/blood-2008-07-161166
Pandey K. B., Rizvi S. I. (2010a). Markers of oxidative stress in erythrocytes and plasma during aging in humans. Oxidative Med. Cell. Longev. 3, 2–12. doi:10.4161/oxim.3.1.10476
Pandey K. B., Rizvi S. I. (2010b). Protective effect of resveratrol on markers of oxidative stress in human erythrocytes subjected to in vitro oxidative insult. Phytotherapy Res. 24, S11–S14. doi:10.1002/ptr.2853
Pantaleo A., Ferru E., Giribaldi G., Mannu F., Carta F., Matte A., et al. (2009). Oxidized and poorly glycosylated band 3 is selectively phosphorylated by Syk kinase to form large membrane clusters in normal and G6PD-deficient red blood cells. Biochem. J. 418, 359–367. doi:10.1042/BJ20081557
Pham K., Parikh K., Heinrich E. C. (2021). Hypoxia and inflammation: insights from high-altitude Physiology. Front. Physiol. 12, 676782. doi:10.3389/fphys.2021.676782
Pham-Huy L. A., He H., Pham-Huy C. (2008). Free radicals, antioxidants in disease and health. Free Radicals Antioxidants 4, 89–96. doi:10.59566/ijbs.2008.4089
Pretini V., Koenen M. H., Kaestner L., Fens M. H. A. M., Schiffelers R. M., Bartels M., et al. (2019). Red blood cells: chasing interactions. Front. Physiol. 10, 945. doi:10.3389/fphys.2019.00945
Quercioli A., Mach F., Montecucco F. (2010). Inflammation accelerates atherosclerotic processes in obstructive sleep apnea syndrome (OSAS). Sleep. Breath. 14, 261–269. doi:10.1007/s11325-010-0338-3
Rabcuka J., Blonski S., Meli A., Sowemimo-Coker S., Zaremba D., Stephenson D., et al. (2022). Metabolic reprogramming under hypoxic storage preserves faster oxygen unloading from stored red blood cells. Blood Adv. 6, 5415–5428. doi:10.1182/bloodadvances.2022007774
Ranjekar P. K., Hinge A., Hegde M. V., Ghate M., Kale A., Sitasawad S., et al. (2003). Decreased antioxidant enzymes and membrane essential polyunsaturated fatty acids in schizophrenic and bipolar mood disorder patients. Psychiatry Res. 121, 109–122. doi:10.1016/S0165-1781(03)00220-8
Remigante A., Morabito R. (2022). Cellular and molecular mechanisms in oxidative stress-related diseases. IJMS 23, 8017. doi:10.3390/ijms23148017
Remigante A., Spinelli S., Basile N., Caruso D., Falliti G., Dossena S., et al. (2022). Oxidation stress as a mechanism of aging in human erythrocytes: protective effect of quercetin. IJMS 23, 7781. doi:10.3390/ijms23147781
Renaud S., De Lorgeril M. (1992). Wine, alcohol, platelets, and the French paradox for coronary heart disease. Lancet 339, 1523–1526. doi:10.1016/0140-6736(92)91277-F
Rossi L., Fraternale A., Bianchi M., Magnani M. (2019). Red blood cell membrane processing for biomedical applications. Front. Physiol. 10, 1070. doi:10.3389/fphys.2019.01070
Saiko P., Szakmary A., Jaeger W., Szekeres T. (2008). Resveratrol and its analogs: defense against cancer, coronary disease and neurodegenerative maladies or just a fad? Mutat. Research/Reviews Mutat. Res. 658, 68–94. doi:10.1016/j.mrrev.2007.08.004
Santos M. A., Franco F. N., Caldeira C. A., De Araújo G. R., Vieira A., Chaves M. M. (2023). Resveratrol has its antioxidant and anti-inflammatory protective mechanisms decreased in aging. Archives Gerontology Geriatrics 107, 104895. doi:10.1016/j.archger.2022.104895
Shan D., Guo S., Wu H.-K., Lv F., Jin L., Zhang M., et al. (2020). Cardiac ischemic preconditioning promotes MG53 secretion through H2O2-activated protein kinase C-δ signaling. Circulation 142, 1077–1091. doi:10.1161/CIRCULATIONAHA.119.044998
Shiga T., Maeda N., Kon K. (1990). Erythrocyte rheology. Crit. Rev. Oncol. Hematol. 10, 9–48. doi:10.1016/1040-8428(90)90020-s
Shin S., Ku Y., Babu N., Singh M. (2007). Erythrocyte deformability and its variation in diabetes mellitus. INDIAN J. Exp. Biol. 45, 121–128.
Sneddon I. N. (1965). The relation between load and penetration in the axisymmetric boussinesq problem for a punch of arbitrary profile. Int. J. Eng. Sci. 3, 47–57. doi:10.1016/0020-7225(65)90019-4
Spinelli S., Straface E., Gambardella L., Caruso D., Falliti G., Remigante A., et al. (2023). Aging injury impairs structural properties and cell signaling in human red blood cells; açaì berry is a keystone. Antioxidants (Basel) 12, 848. doi:10.3390/antiox12040848
Suwalsky M., Villena F., Gallardo M. J. (2015). In vitro protective effects of resveratrol against oxidative damage in human erythrocytes. Biochimica Biophysica Acta (BBA) - Biomembr. 1848, 76–82. doi:10.1016/j.bbamem.2014.09.009
Tabrizi R., Tamtaji O. R., Lankarani K. B., Akbari M., Dadgostar E., Dabbaghmanesh M. H., et al. (2020). The effects of resveratrol intake on weight loss: a systematic review and meta-analysis of randomized controlled trials. Crit. Rev. Food Sci. Nutr. 60, 375–390. doi:10.1080/10408398.2018.1529654
Tasali E., Ip M. S. M. (2008). Obstructive sleep apnea and metabolic syndrome: alterations in glucose metabolism and inflammation. Proc. Am. Thorac. Soc. 5, 207–217. doi:10.1513/pats.200708-139MG
Taylor C. T., Moncada S. (2010). Nitric oxide, cytochrome C oxidase, and the cellular response to hypoxia. ATVB 30, 643–647. doi:10.1161/ATVBAHA.108.181628
Vercellati C., Marcello A. P., Fattizzo B., Zaninoni A., Seresini A., Barcellini W., et al. (2022). Effect of primary lesions in cytoskeleton proteins on red cell membrane stability in patients with hereditary spherocytosis. Front. Physiol. 13, 949044. doi:10.3389/fphys.2022.949044
Vestergaard M., Ingmer H. (2019). Antibacterial and antifungal properties of resveratrol. Int. J. Antimicrob. Agents 53, 716–723. doi:10.1016/j.ijantimicag.2019.02.015
Walmsley S., Harris A., Thompson A. A. R., Whyte M. K. B. (2014). HIF-mediated innate immune responses: cell signaling and therapeutic implications. HP 47, 47–58. doi:10.2147/HP.S50269
Walmsley S. R., Whyte M. K. B. (2014). Neutrophil energetics and oxygen sensing. Blood 123, 2753–2754. doi:10.1182/blood-2014-03-560409
Weiskirchen S., Weiskirchen R. (2016). Resveratrol: how much wine do you have to drink to stay healthy? Adv. Nutr. 7, 706–718. doi:10.3945/an.115.011627
Xu P., Chen C., Zhang Y., Dzieciatkowska M., Brown B. C., Zhang W., et al. (2022). Erythrocyte transglutaminase-2 combats hypoxia and chronic kidney disease by promoting oxygen delivery and carnitine homeostasis. Cell Metab. 34, 299–316.e6. doi:10.1016/j.cmet.2021.12.019
Yang Q., Noviana M., Zhao Y., Chen D., Wang X. (2019). Effect of curcumin extract against oxidative stress on both structure and deformation capability of red blood cell. J. Biomechanics 95, 109301. doi:10.1016/j.jbiomech.2019.07.045
Yannoukakos D., Meyer H. E., Vasseur C., Driancourt C., Wajcman H., Bursaux E. (1991). Three regions of erythrocyte band 3 protein are phosphorylated on tyrosines: characterization of the phosphorylation sites by solid phase sequencing combined with capillary electrophoresis. Biochim. Biophys. Acta 1066, 70–76. doi:10.1016/0005-2736(91)90252-4
Yoshida T., Prudent M., D’Alessandro A. (2019). Red blood cell storage lesion: causes and potential clinical consequences. Blood Transfus. 17, 27–52. doi:10.2450/2019.0217-18
Keywords: resveratrol, band 3 crosslinking, hypoxia, phosphorylation, erythrocyte deformability
Citation: Yang Q, Chen D, Li C, Liu R and Wang X (2024) Mechanism of hypoxia-induced damage to the mechanical property in human erythrocytes—band 3 phosphorylation and sulfhydryl oxidation of membrane proteins. Front. Physiol. 15:1399154. doi: 10.3389/fphys.2024.1399154
Received: 11 March 2024; Accepted: 05 April 2024;
Published: 19 April 2024.
Edited by:
Angelo D’Alessandro, University of Colorado Denver, United StatesReviewed by:
Alessia Remigante, University of Messina, ItalyFrancesco Misiti, University of Cassino, Italy
Copyright © 2024 Yang, Chen, Li, Liu and Wang. This is an open-access article distributed under the terms of the Creative Commons Attribution License (CC BY). The use, distribution or reproduction in other forums is permitted, provided the original author(s) and the copyright owner(s) are credited and that the original publication in this journal is cited, in accordance with accepted academic practice. No use, distribution or reproduction is permitted which does not comply with these terms.
*Correspondence: Xiang Wang, eHdhbmdjaG5AY3F1LmVkdS5jbg==