- 1DSM Nutritional Products, Kaiseraugst, Switzerland
- 2Department of Poultry Science, University of Arkansas, Fayetteville, AR, United States
- 3DSM Nutritional Products, Jerusalem, OH, United States
The objective of the present study was to determine the effect of a novel (4th generation) phytase supplementation as well as its mode of action on growth, meat quality, and incidence of muscle myopathies. One-day old male broilers (n = 720) were weighed and randomly allocated to 30 floor pens (24 birds/pen) with 10 replicate pens per treatment. Three diets were fed from hatch to 56- days-old: a 3-phase corn-soy based diet as a positive control (PC); a negative control (NC) formulated to be isocaloric and isonitrogenous to the PC and with a reduction in Ca and available P, respectively; and the NC supplemented with 2,000 phytase units per kg of diet (NC + P). At the conclusion of the experiment, birds fed with NC + P diet were significantly heavier and had 2.1- and 4.2-points better feed conversion ratio (FCR) compared to birds offered NC and PC diets, respectively. Processing data showed that phytase supplementation increased live weight, hot carcass without giblets, wings, tender, and skin-on drum and thigh compared to both NC and PC diets. Macroscopic scoring showed that birds fed the NC + P diet had lower woody breast (WB) severity compared to those fed the PC and NC diets, however there was no effect on white striping (WS) incidence and meat quality parameters (pH, drip loss, meat color). To delineate its mode of action, iSTAT showed that blood glucose concentrations were significantly lower in birds fed NC + P diet compared to those offered PC and NC diets, suggesting a better glucose uptake. In support, molecular analyses demonstrated that the breast muscle expression (mRNA and protein) of glucose transporter 1 (GLUT1) and glucokinase (GK) was significantly upregulated in birds fed NC + P diet compared to those fed the NC and PC diets. The expression of mitochondrial ATP synthase F0 subunit 8 (MT-ATP8) was significantly upregulated in NC + P compared to other groups, indicating intracellular ATP abundance for anabolic pathways. This was confirmed by the reduced level of phosphorylated-AMP-activated protein kinase (AMPKα1/2) at Thr172 site, upregulation of glycogen synthase (GYS1) gene and activation of mechanistic target of rapamycin and ribosomal protein S6 kinase (mTOR-P70S6K) pathway. In conclusion, this is the first report showing that in-feed supplementation of the novel phytase improves growth performance and reduces WB severity in broilers potentially through enhancement of glucose uptake, glycolysis, and intracellular ATP production, which used for muscle glycogenesis and protein synthesis.
Introduction
The US broiler industry is the largest producer of meat-type chickens, producing approximately 9.17 billion chickens in 2022 with a production value of $50,445,885,000 (USDA, 2023). Although it supports the livelihoods and food security of billions of people, the US broiler industry is facing several challenges from a steep projected increase in global demand for high quality animal protein by 73% by 2050; and the need to adapt to environmental constraints driven by climate changes and already scarce natural resources (water, energy, land). Additional challenges facing broiler production in the next decades will include, but are not limited to, water shortage, disease challenges and zoonoses, environmental footprint, and pollution concerns, while maintaining affordable, healthy, adequate, nutritious, and wholesome protein supply to feed the future of an ever-increasing world-population.
Poultry meat is highly regarded worldwide as one of the most efficient food sources with high quality nutrients, relatively inexpensive, and without religious taboos (Cavani et al., 2009; Marangoni et al., 2015). However, the newly emerging breast muscle disorders, woody breast (WB) (Bilgili et al., 2014) and white stripping (WS) (Bauermeister et al., 2009), have had an increasingly negative impact on worldwide chicken meat production and quality (Mudalal et al., 2015; Trocino et al., 2015). White stripping is characterized by visible white striations parallel to breast muscle fibers (Kuttappan et al., 2013), however WB is distinguished by a hard consistency to raw breast filets (Sihvo et al., 2014). Although probable different etiologies, both myopathies appeared in varying degree and more often together on the same breast filet. The lesions associated with both myopathies appear to be aseptic, superficially located, and include muscle fiber fragmentation, hyalinization, and swelling with replacement by fibrous connective tissue, as well as an influx of macrophages and other immune and fat cells (Soglia et al., 2016; Baldi et al., 2018). This constitutes a major animal health, welfare, and economic concern that costs the industry several hundred million dollars a year due to on-farm culling and mortality, down-grading, and condemnation at processing, as well as rejection from human consumption, and for which there is no effective solution because of its unknown etiology (Che et al., 2022). There is, therefore, a critical need to define its causation and identify mechanism-based strategies to prevent it.
Several high throughput omics studies postulated the involvement of diverse dysregulated cellular processes, including hypoxia, oxidative stress, and altered glucose utilization, in the progression of these muscle myopathies. Using functional mechanistic studies, Dridi’s group has shown that hypoxia induces WB through the unfolded protein response, ER stress, and modulation of satellite cell fate (Emami et al., 2021; Greene et al., 2023). Supplementation of high dose of phytase reduced the severity of WB via the improvement of local (muscle) and systemic oxygen homeostasis and modulation of muscle metabolome and fatty acid profile (Greene et al., 2019; Cauble et al., 2020; Greene et al., 2020). Furthermore, it has been reported that when fed at high dose, exogenous phytase induces a rapid and complete breakdown of diet-derived phytates, reduces the anti-nutritional effects, and thereby elicits a greater nutrient utilization, better growth (Shirley and Edwards, 2003; Rutherfurd et al., 2012), and free myo-inositol (Schmeisser et al., 2017; Gonzalez-Uarquin et al., 2020). Recently, a novel 4th generation phytase, HiPhorius™ (DSM Nutritional Products, Kaiseraugst, Switzerland), has been developed for optimal enzyme activity, thermo-resistance, stability to wide pH range in the gastrointestinal tract, and low phosphorus and greenhouse gas emissions (Imtiaz, 2022). It has been found to further improve broiler growth compared to classical phytase (Ronozyme HiPhos, DSM Nutritional products, Kaiseraugst, Switzerland) (Zhang et al., 2022). Yet, its mode of action is still not well defined. The aim of the present study was, therefore, to determine the effect and the mode of action of HiPhorius™ on growth performance, blood parameters, and muscle myopathy incidences in broiler chickens.
Materials and methods
Experimental design and diets
A total of 720 day-old straight run broiler chicks (Ross 708, Aviagen, Huntsville, AL) were obtained from a local commercial hatchery (Simmons Foods, Siloam Springs, AR) and transported to the University of Arkansas Broiler Research Farm. Upon arrival, chicks were randomly placed into 30 temperature-controlled pens at 24 birds per pen with a density of 0.096 m2/bird. Each pen was equipped with a hanging feeder, section of continuous nipple drinker line (5 nipples per pen), and built-up, top-dressed litter composed of clean pine shavings. All broilers were fed a 3-phase feeding program; d0-18 starter, d19-36 grower, and d37-56 finisher. Each pen was assigned to 1 of 3 isocaloric and isonitrogenous experimental treatments (10 pens/treatment), which consisted of a positive control with adequate nutrient supply (PC), negative control with a reduction of 0.08% Ca and 0.15% available P (NC), and a negative control supplemented with 2,000 phytase units/kg (FYT per kg of feed) of a novel fourth generation phytase [HiPhorius™, DSM Nutritional Products, Kaiseraugst, Switzerland, (NC + P)] (Table 1). Feed and water were provided ad libitum throughout the experiment. Initial temperatures were set at 32°C and gradually reduced to 20°C by d28 and remained there until the conclusion of the experiment. Lighting schedules were settled at 24L:0D for d0, 23L:1D from d1-6, and 18L:6D from d7-56. Light intensities were verified at bird level using a light meter (LT300, Extech Instruments, Waltham, MA). Birds were weighed and feed intake were measured by pen at d0, 18, 36, and 55 post hatch. Averaged body weight (BW), body weight gain (BWG), feed intake (FI), and mortality corrected feed conversion ratio (FCR) were subsequently calculated. These parameters and corresponding mortality were calculated for each phase and overall growth performance.
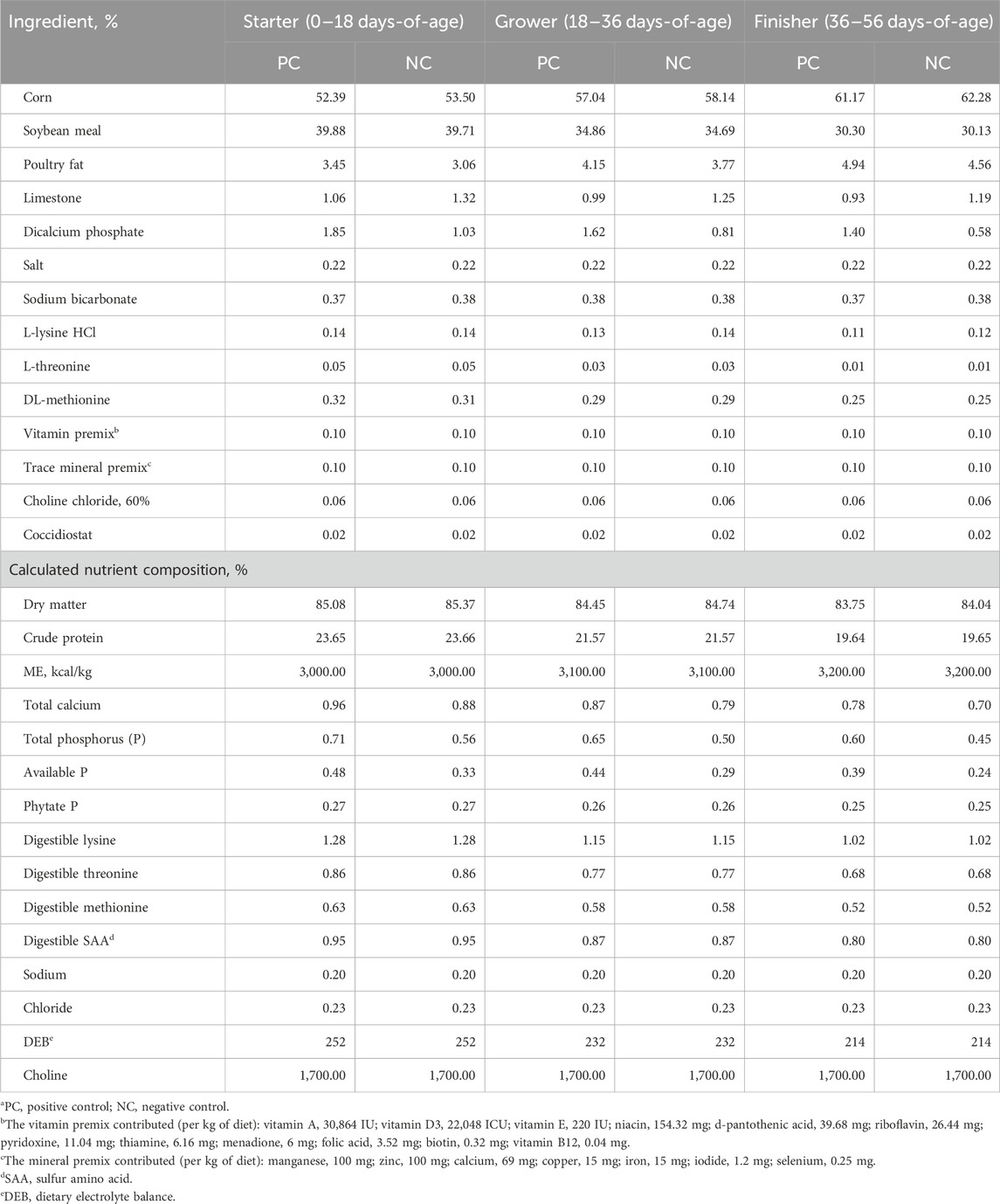
TABLE 1. Ingredients and nutrient composition of the experimental dietsa.
Blood parameters and tissue collection
On d55, 72 birds (8 pens/treatment; 3 birds/pen) were randomly selected, weighed, and sampled for venous blood analysis using an i-STAT Alinity system (SN:801128); software version JAMS 88.A.1/CLEW D44; Abaxis, Union City, CA, United States) with the i-STAT CG8+ cartridge test (ABBT-03P88-25) according to manufacturer’s recommendation. Heparinized whole blood (0.2 mL) was analyzed for ionized sodium (iNa), potassium (iK), chloride (iCl), calcium (iCa), total carbon dioxide (TCO2), glucose (Glu), bicarbonate (HCO−3), and pH. The i-STAT system has been validated in avian species (Martin et al., 2010; Schaal et al., 2016). Birds were euthanized by cervical dislocation immediately following blood draw and a cranial sample of left breast muscle (Pectoralis major) was removed, snap frozen in liquid nitrogen, and stored at −80°C until use.
Processing
All birds underwent a feed withdrawal period of 10 h prior being transported to the University of Arkansas Pilot Processing Plant on d56. Five hundred and forty birds (18 birds/pen) were weighed to obtain live dock weights before being placed on shackles, electrically stunned (11 V, 11 mA for 11 s), exsanguinated, soft scalded (55°C for 2 min), de-feathered (Foodcraft Model 3; Baker international, MI, United States), and mechanically eviscerated. With-out giblets (WOG) and abdominal fat weights were then recorded, before being chilled for 3 h at 4°C. Chilled with-out giblets (CWOG) weights were recorded, before being deboned to acquire breast (Pectoralis major), tender (Pectoralis minor), wing, and leg quarter weights.
Woody breast (WB) and white striping (WS) palpation and scoring
On d28 and 49, WB occurrence was estimated via live-bird palpation as previously described (Greene et al., 2019). After slaughter process at d56, breast filets were macroscopically scored and classified to WB and WS categories (Supplementary Figure S1). For WB and as described previously, a normal category (NORM) has a degree 0 with flexible breast throughout, moderate (MOD) has a degree 0.5–1.5 with mild hardening in the caudal area, and severe (SEV) has a degree with severe hardening and hemorrhagic lesions in the caudal region (Greene et al., 2019). For WS, a three-category scoring scheme, primarily accounting for the thickness and density of striations, has been used: normal (NORM) category is characterized by absence of white lines, moderate (MOD) with small (∼<1 mm), and severe (SEV) which is marked by large white striation (>1–2 mm) (Supplementary Figure S1) (Vignale et al., 2017).
Meat quality
Breast fillets were collected after processing, placed on trays, and covered with plastic overlay before being stored at 4°C for 24 h for drip loss, pH, and colorimetric analysis. Drip loss (%) was calculated as the difference between hot debone breast weights and chilled breast weights and is expressed as a percentage of WOG. A Minolta colorimeter (CR-400; Konica Minolta Sensing Inc., Sakai Osaka, Japan; size 102(W) X 217 (H) X 63 (D) mm) using illuminant D65 and a 2-degree observer was used to determine the L* (lightness), a* (redness), and b* (yellowness) values from three readings on the ventral side of the left breast fillet. The same breast fillet was used to measure the average pH, from 3 readings, with a temperature-compensating pH meter (Testo 205; Testo Inc., West Chester, PA).
RNA extraction, reverse transcription, and quantitative real-time PCR
Total RNAs were extracted from chicken left-breast muscle using Trizol reagent (Life Technologies, Carlsbad, CA) according to the manufacturer’s recommendations. After DNAse treatment and purification, the concentrations of total RNAs were measured for each breast muscle sample by Take 3 Micro-Volume Plate using Synergy HT multi-mode micro plate reader (BioTek, Winooski, VT), and RNA integrity and quality were assessed by both OD260/OD280 nm absorption ratio (>1.8) and by using 1% agarose gel electrophoresis. Reverse transcription and qPCR were performed as previously described (Dridi et al., 2012; Greene et al., 2023). In brief, RNA (1 µg) was reverse transcribed using qScript cDNA Synthesis Supermix (Quanta Biosciences, Gaithersburg, MD) in a 20 µL total reaction under the following conditions (42°C for 30 min followed by 85°C for 5 min). cDNAs were amplified by qPCR (Applied Biosystems 7500 Real Time System) with Power-Up Sybr green master mix (Life Technologies, Carlsbad, CA), 5 µL of 10X diluted cDNA, and 0.5 µM of each forward and reverse specific primers as previously described (Dridi et al., 2012). Oligonucleotide primers specific for chicken mechanistic target of rapamycin (mTOR), ribosomal protein S6 kinase A1 (RPS6K), adenosine monophosphate-activated protein kinases (AMPKα1, AMPKα2, AMPKβ1, AMPKβ2, AMPKγ1, AMPKγ2, AMPKγ3), ribosomal RNA 18S and beta-actin have been previously reported (Nguyen et al., 2015; Vignale et al., 2015; Blankenship et al., 2016). Oligonucleotide primers specific for chicken glucose transporters (GLUT1, GLUT3, GLUT6, GLUT8, GLUT10, and GLUT12), glucokinase (GK), hexokinases (HK1, HK2, and HK3), glycogen synthases (GYS1 and GYS2), glycogen branching enzyme (GBE1), glycogen debranching enzyme (AGL), mitochondrial ATP synthase F0 subunit 8 (MT-ATP8), glycogen synthase kinase 3 beta (GSK-3β), eukaryotic translation initiation factor 2 alpha kinase 4 (EIF2AK4 or GCN2), eukaryotic translation initiation factor 2 subunit alpha (EiF2α), and eukaryotic translation initiation factor 4E binding protein 1 (EIF4EBP1) are summarized in Table 2. Relative expression of the target genes was determined using the 2−ΔΔCT method (Schmittgen and Livak, 2008), with normalization to ribosomal 18S expression. PC broilers were used as a calibrator.
Western blot analysis
The immunoblot analyses were performed as previously described (Dridi et al., 2012; Emami et al., 2021; Greene et al., 2023). In brief, muscle tissues were homogenized in lysis buffer using bullet blender storm (NextAdvance, Averill Park, NY). Following concentration determination by a Bradford assay kit (Bio-Rad, Hercules, CA) using a Synergy HT multimode microplate reader (Biotek Agilent, Winooski, VT), total proteins (70–100 µg) were run in 4%–12% gradient Bis-Tris gels (Life Technologies, Carlsbad, CA) and then transferred to polyvinylidene difluoride (PVDF) membranes. After transfer, PVDF membranes were blocked using a Tris-buffered saline (TBS) with 5% nonfat milk and Tween 20 at room temperature for 1 h. The membranes were washed with TBS and Tween 20 and then incubated with primary antibodies at a dilution of either 1:500 or 1:1000 overnight at 4°C. Primary antibodies used were rabbit anti-glucose transporter 1 (GLUT1 #A6982, ABClonal, Woburn, MA), rabbit anti-GLUT12 (#LS-C110860, LSBio, Lynwood, WA), rabbit anti-glucokinase (GK, #A15059, ABClonal, Woburn, MA), rabbit anti-hexokinase 1(HK1, #A1054, ABClonal, Woburn, MA), rabbit anti-HK2 (#A20829, ABClonal, Woburn, MA), rabbit anti-phospho-AMP-activated protein kinase (AMPKα1/α2)Thr172 (#2531, Cell Signaling, Technology, Danvers, MA), rabbit anti-AMPKα1/α2 (#2603, Cell Signaling, Technology, Danvers, MA), rabbit anti-phospho-mechanistic target of rapamycin (mTOR)Ser2448 (#2971, Cell Signaling, Technology, Danvers, MA), rabbit anti-mTOR (#2972, Cell Signaling, Technology, Danvers, MA), goat anti-phospho-P70S6 kinase (P70S6K)Thr389 (#SC-11759, Santa Cruz Biotechnology, Dallas, TX), rabbit anti-P70S6K (#SC-230, Santa Cruz Biotechnology, Dallas, TX), mouse anti-phospho-glycogen synthase kinase 3 beta (GSK-3β)Ser9 (#SC-373800, Santa Cruz Biotechnology, Dallas, TX), and rabbit anti-Glyceraldehyde 3-phosphate dehydrogenase (GAPDH, #NB300-327, Novus Biologicals, Centennial, CO) as a housekeeping protein. Pre-stained molecular weight marker (Precision Plus Protein Dual Color) was used as a standard (BioRad, Hercules, CA) and as indicator for transfer efficiency. The secondary anti-mouse (#SC-358914, Santa Cruz Biotechnology, Dallas, TX)-, anti-goat (#SC-2354, Santa Cruz Biotechnology, Dallas, TX)-, and anti-rabbit (#7074S, Cell Signaling, Technology, Danvers, MA)-IgG-HRP-linked antibodies were used at 1:5,000 dilution for 1 h at room temperature. The signal was visualized by enhanced chemiluminescence (ECL plus) (GE Healthcare Bio-Sciences, Buckinghamshire, United Kingdom) and captured by FluorChem M MultiFluor System (Proteinsimple, Santa Clara, CA). Image Acquisition and Analysis were performed by AlphaView software (Version 3.4.0, 1993–2011, Proteinsimple, Santa Clara, CA).
Statistical analyses
Data were analyzed by One-way ANOVA and Tukey’s HSD multiple comparison test using the Mixed Model platform of JMP Pro v. 17.0 (SAS Institute, Cary, NC, United States) for the growth, blood parameters, and meat quality and processing data, and Graph Pad Prism version 9.00 for Windows (Graph Pad Software, La Jolla California, United States) for muscle gene and protein expression profiles. For the growth data, pen served as the experimental unit and pen averages were calculated and analyzed for each quality characteristic. For the gene and protein expression, however, the bird was used as the experimental unit. For the muscle myopathy incidence, bird was the experimental unit and score was considered an ordinal variable. The model included diet. When diet was significant, score means between diets were separated using Pearson Chi-square. Statistical significance was set at p ≤ 0.05.
Results
Effect of phytase supplementation on growth performance
Phytase recoveries in the NC + P diet were 1,829; 1,595; and 1,771 FYT/kg in the starter, grower, and finisher phases, respectively. Broiler performance was approximately 83% of Ross 708 breed guidelines (Aviagen, Huntsville, AL) at d55. The overall mortality for the entire period (0–55 days) was high at 17.9% ± 7.5% and not influenced by treatment (p = 0.9798); the majority occurred from d18-55. This elevated mortality was a result of intense heat waves that happed on day 31 and 43 of the experiment (data not shown).
At the conclusion of the experiment (d55), birds fed with NC + P diet were significantly heavier and gained more (∼112 g/bird, p < 0.05) than the birds offered the PC and NCdiets (Table 3). There was no difference in feed intake between all studied groups, which resulted in 2.1- and 4.2-points better FCR for the birds fed NC + P diet compared to those fed NC and PC diets, respectively (Table 3). When categorized by rearing phase, birds fed NC + P diet had better FCR than their counterparts fed NC and PC diets at all periods (Tables 3), but the difference was statistically discernible only at starter period (Table 3). Birds fed the NC diet ate less feed (p < 0.05), gained less, and were significantly lighter compared to those offered the PC and NC + P diets at the starter phase, then they compensated and catch-up similar growth as the birds fed the PC diet during the grower and finisher periods (Tables 3).
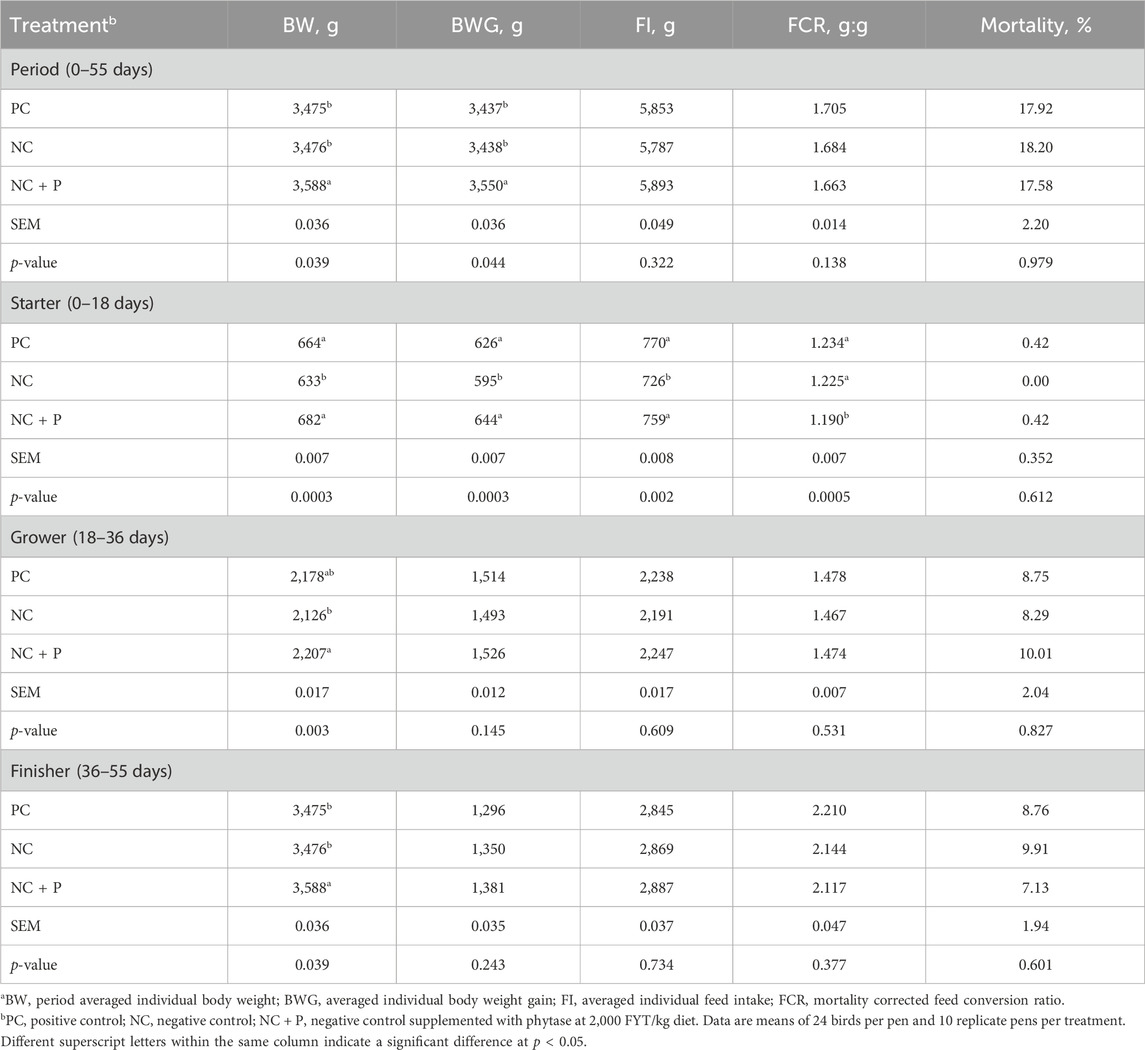
TABLE 3. Growth performances during the entire experiment and by rearing phasea.
Effect of phytase supplementation on carcass parameters and meat quality
As depicted in Table 4, phytase supplementation significantly increased live weight at processing, wings, and skin-on drum and thigh (LQ) compared to birds fed both NC and PC diets. Chickens fed NC + P diet exhibited a significantly higher tender weight compared to those offered the PC diet (Table 4). Phytase supplementation tended to increase WOG and CWOG weights compared to PC and NC diets (p = 0.05, Table 4). Although it was not statistically significant, birds fed NC + P diet exhibited higher breast weight (17 and 13 g) compared to those fed the PC and NC diets (Table 4). There was no significant difference in abdominal fat content between the studied groups (Table 4).
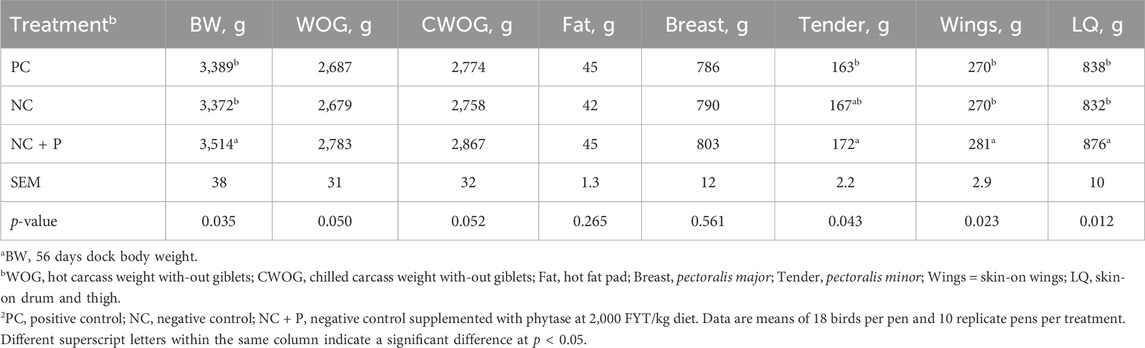
TABLE 4. Processing weights of 56 days-old Ross 708 broilersa.
There was no effect of treatment on the incidence of WS scores and incidence (Table 5). The combination of live-bird palpation and macroscopic scoring showed that WB incidence increased with age in all studied groups, but the amplitude was significantly lower in birds fed the NC + P diet particularly at d49 and d56 (Figure 1) compared to the other groups. Table 6 showed that phytase sypplementation reduced the WB severity (SEV, p = 0.0531) compared to PC and NC diets. Breast meat quality parameters (pH, drip loss, lightness, redness, and yellowness) were not influenced by the dietary treatments (Table 7).
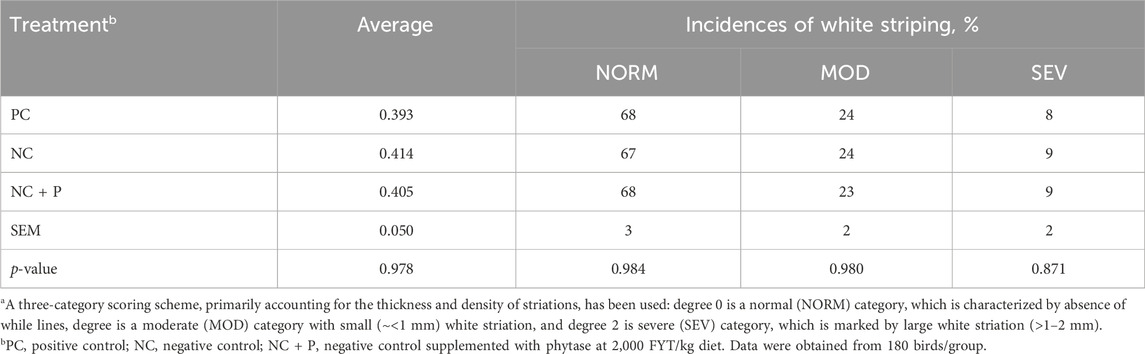
TABLE 5. White stripping incidence in 56 days-old Ross 708 broilersa.
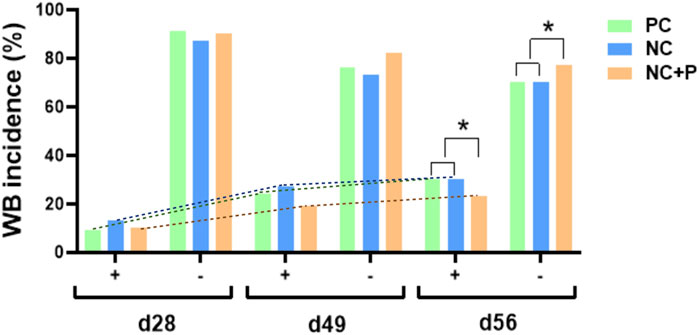
FIGURE 1. Effect of phytase supplementation on WB incidence in broilers at different ages. WB occurrence and incidence were assessed by live-bird palpation at the age of 28 and 49 days, and macroscopically scored at the processing (56 days). Data are presented as WB presence (+) or absence (−). WB incidence increased with age in all studied groups, but the amplitude was significantly lower in the NC + P birds particularly at d49 and d56. NC, negative control; NC + P, negative control supplemented with 2,000 phytase units/kg (FYT per kg of feed); PC, positive control.
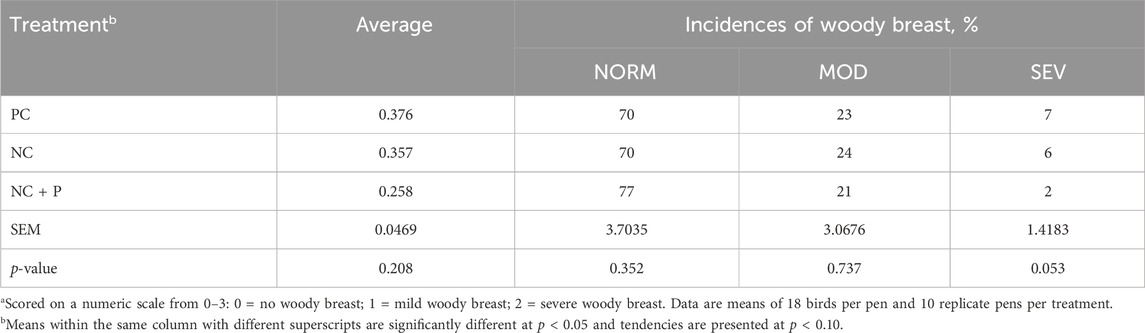
TABLE 6. Woody breast incidence in 56 days-old Ross 708 broilersa.
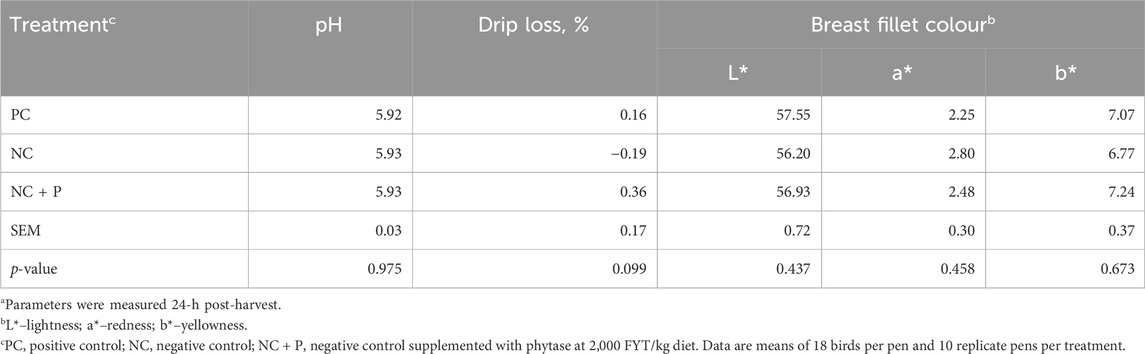
TABLE 7. Breast meat quality of 56 days-old Ross 708 broilersa.
Effect of phytase supplementation on blood parameters
There was no effect of dietary treatment on the concentration of Na, Ca, total CO2, or bicarbonate in the whole blood. However, birds fed the NC + P diet had a greater concentration of K (p = 0.0083) in the blood compared with birds fed the NC, and intermediate in birds fed the PC. Blood glucose (p = 0.037) and pH (p = 0.018) were lower in birds fed the NC + P diet compared with birds fed the PC and NC diets, respectively (Table 8).
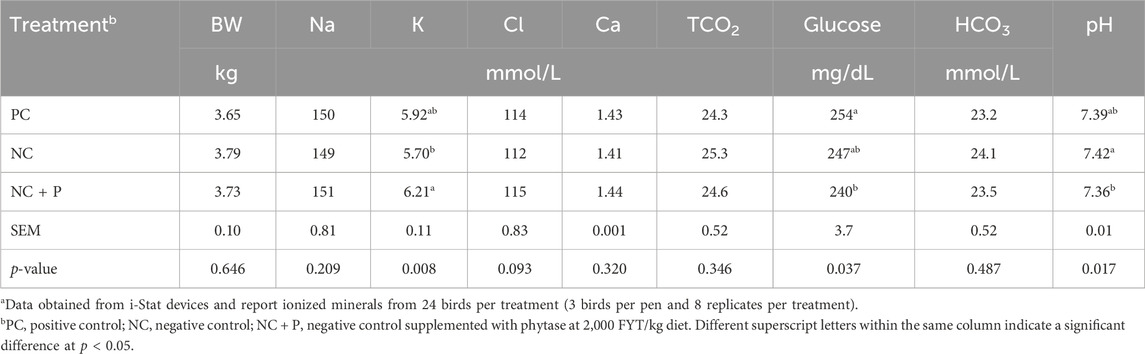
TABLE 8. Blood parameters of 56-old Ross 708 broilersa.
Effect of phytase supplementation on muscle gene and protein expression profile
As shown in Figure 2A, the tested GLUT (GLUT1, 3, 6, 8, 10, and 12) genes are all expressed in chicken breast muscle. The expression of GLUT1 and GLUT6 was significantly upregulated in phytase-supplemented (NC + P) birds compared to NC and PC groups (Figures 2B, C). Compared to PC birds, GLUT3 mRNA levels were significantly higher in NC, and GLUT10 mRNA abundances were higher in NC + P (Figures 2D, E). mRNA abundances of GLUT8 and GLUT12 were significantly increased in NC compared to PC and NC + P birds (Figures 2F, G). Immunoblot analyses showed that GLUT12 and GLUT1 protein levels were significantly induced in NC + P compared to the other groups (Figures 2H, I).
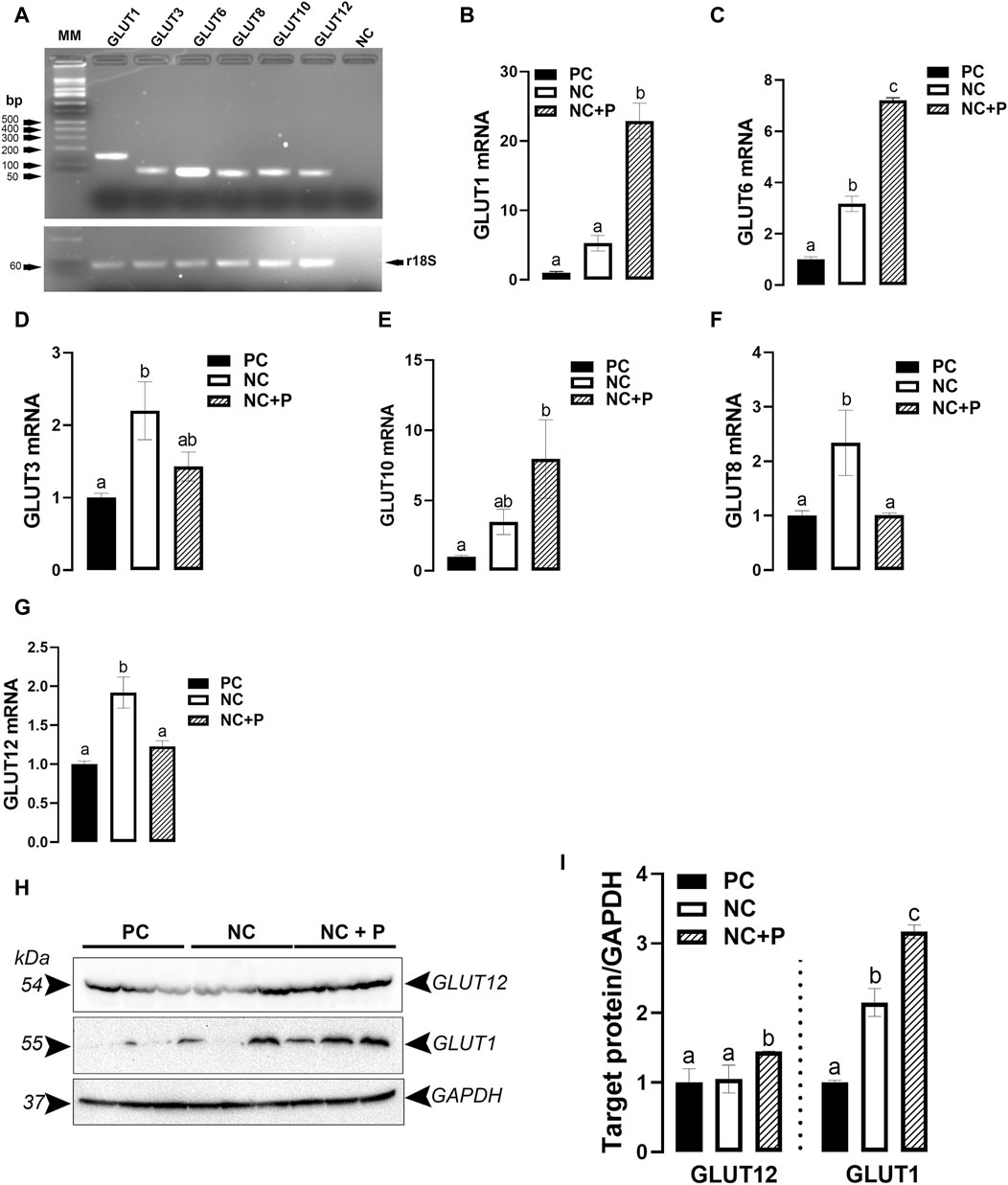
FIGURE 2. Effect of phytase supplementation on the expression profile of breast muscle glucose transporters (GLUTs). Several GLUT genes are expressed in the breast muscle (A). mRNA abundances of GLUT1 (B), GLUT6 (C), GLUT3 (D), GLUT10 (E), GLUT8 (F), GLUT12 (G) were measured by real-time quantitative PCR and analyzed by 2−ΔΔCt method (Schmittgen and Livak, 2008). Protein levels of GLUT1 and GLUT12 were determined by immunoblot (H,I). Data are presented as mean ± SEM (n = 6 birds/group). Different letters indicate significant difference at p < 0.05. GLUT, glucose transporter; NC, negative control; NC + P, negative control supplemented with 2,000 phytase units/kg (FYT per kg of feed); PC, positive control.
As depicted in Figure 3A, GK (also known as HK4) and HK (1, 2, and 3) genes are all expressed in chicken breast muscle. Phytase supplementation significantly upregulated the expression of GK and HK2 genes, but it downregulated that of HK1 compared to the other groups (Figures 3B–D). The expression of HK3 remained unchanged between all studied groups (Figure 3E). Western blot analyses showed that GK protein levels were significantly increased in NC + P birds compared to the other groups, however HK1 and HK2 remained unchanged between all tested groups (Figures 3F, G).
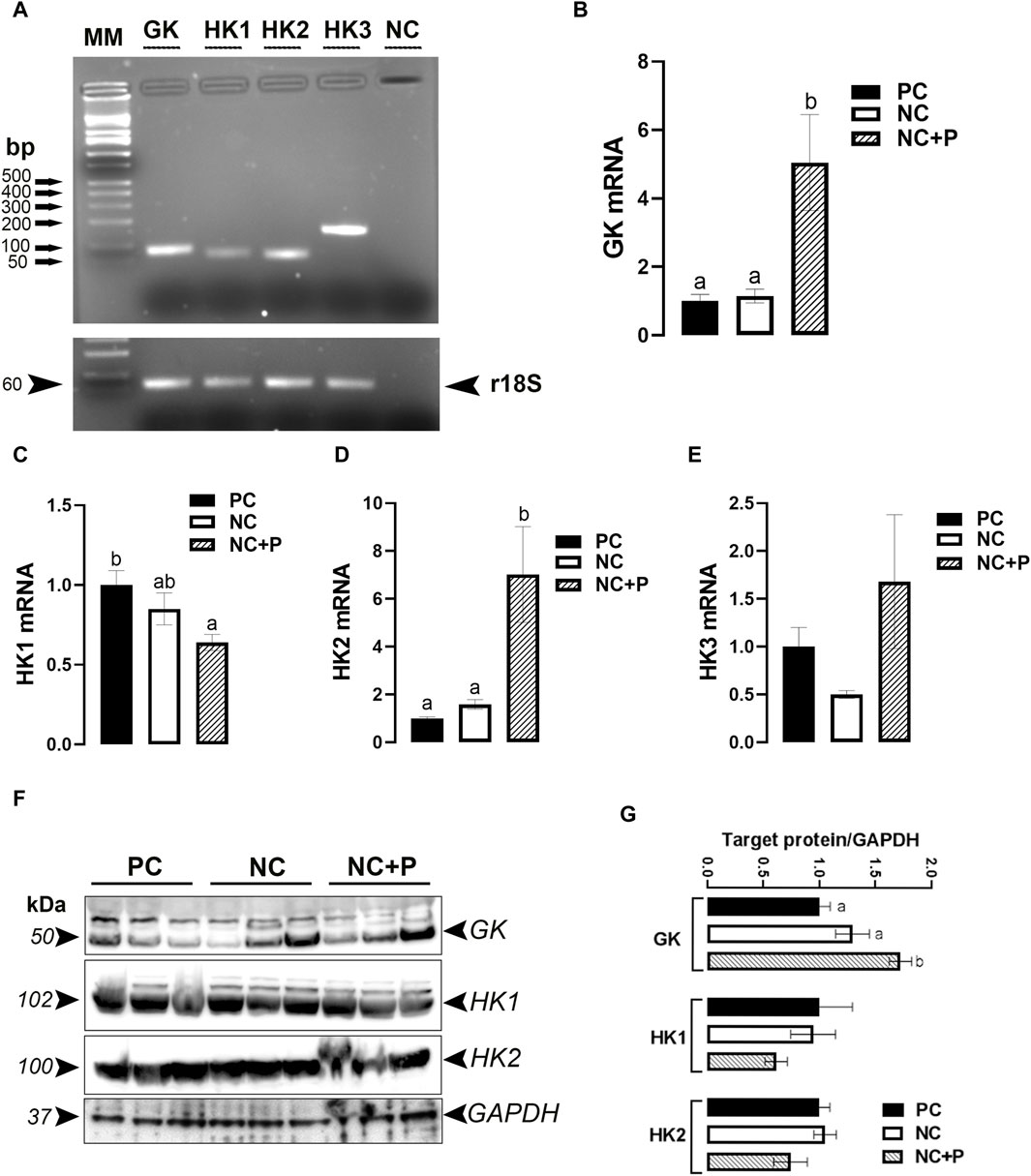
FIGURE 3. Effect of phytase supplementation on the expression profile of breast muscle hexokinases. Hexokinase-coding genes are expressed in the breast muscle (A). mRNA abundances of GK (B), HK1 (C), HK2 (D), and HK3 (E) were measured by real-time qPCR and analyzed by 2−ΔΔCt method (Schmittgen and Livak, 2008). Protein levels of GK, HK1, and HK2 were determined by immunoblot (F,G). Data are presented as mean ± SEM (n = 6 birds/group). Different letters indicate significant difference at p < 0.05. GK, glucokinase (or HK4), HK, hexokinase; NC, negative control; NC + P, negative control supplemented with 2,000 phytase units/kg (FYT per kg of feed); PC, positive control.
The expression of breast muscle GYS1 was significantly upregulated in NC + P birds compared to the other groups, however GYS2 mRNA levels were significantly higher in both NC and NC + P compared to PC group (Figures 4A, B). Phytase supplementation significantly downregulated the expression of both AGL and GBE1 expression, however NC diet significantly upregulated the expression of the abovementioned genes (Figures 4C, D).
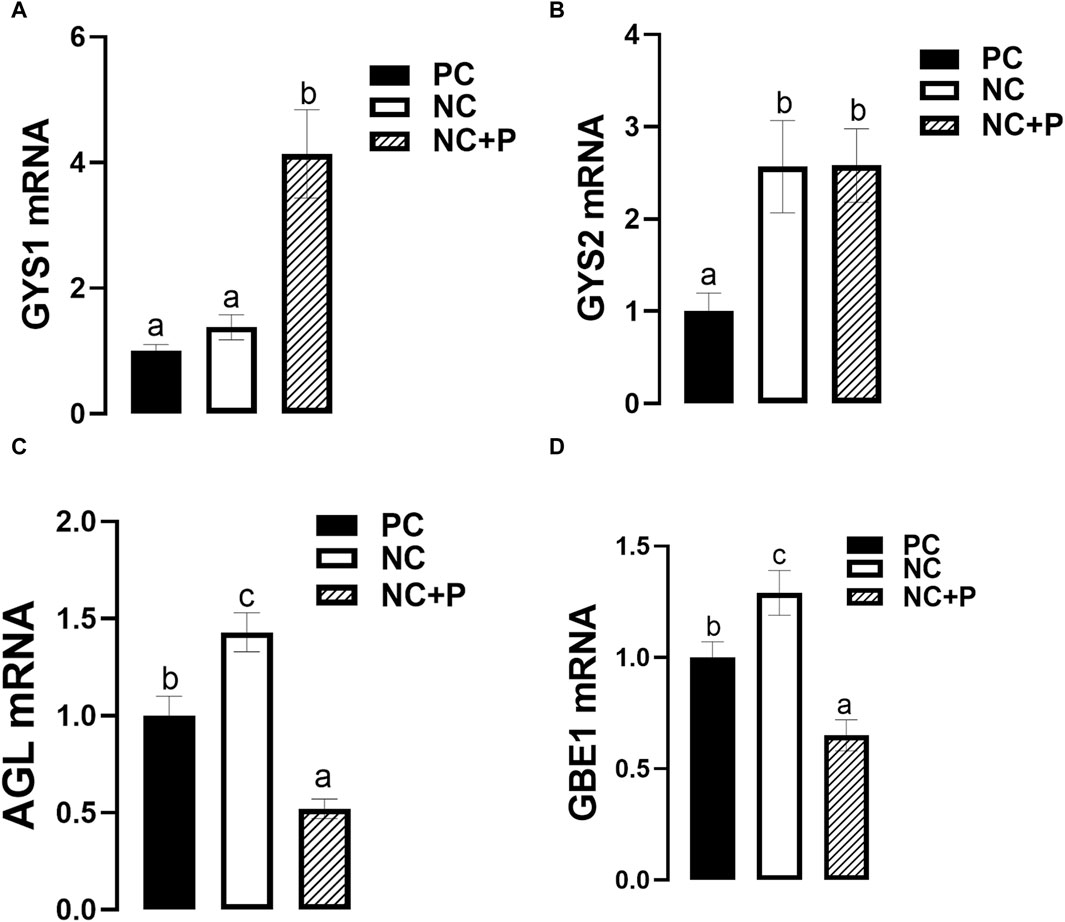
FIGURE 4. Effect of phytase supplementation on the expression of glycogen metabolism-associated genes in broiler breast muscle. Gene expression of Gys1 (A), Gys2 (B), AGL (C), and GBE1 (D) were measured by real-time qPCR and analyzed by 2−ΔΔCt method (Schmittgen and Livak, 2008). Data are presented as mean ± SEM (n = 6 birds/group). Different letters indicate significant difference at p < 0.05. AGL, glycogen debranching enzyme; GBE1, glycogen branching enzyme; Gys, glycogen synthase; NC, negative control; NC + P, negative control supplemented with 2,000 phytase units/kg (FYT per kg of feed); PC, positive control.
Phytase supplementation significantly reduced the phosphorylated levels of the catalytic subunit AMPKα1/α2 at Thr172 site, indicating a decreased activity of AMPK in NC + P birds compared to the other groups (Figures 5A, B). Real-time quantitative PCR showed that phytase supplementation significantly downregulated the expression of AMPKα2 and upregulated that of AMPKγ3 and MT-ATP8 compared to PC and NC groups (Figures 5D, I, J). The expression of AMPKβ1was significantly higher in both NC and NC + P compared to PC birds (Figure 5E), however the mRNA abundances of AMPKγ1 was significantly induced only in NC chickens compared to PC group (Figure 5G). The expression of AMPKα1, AMPKβ2, and AMPKγ2 remained unchanged between all studied groups (Figures 5C, F, H).
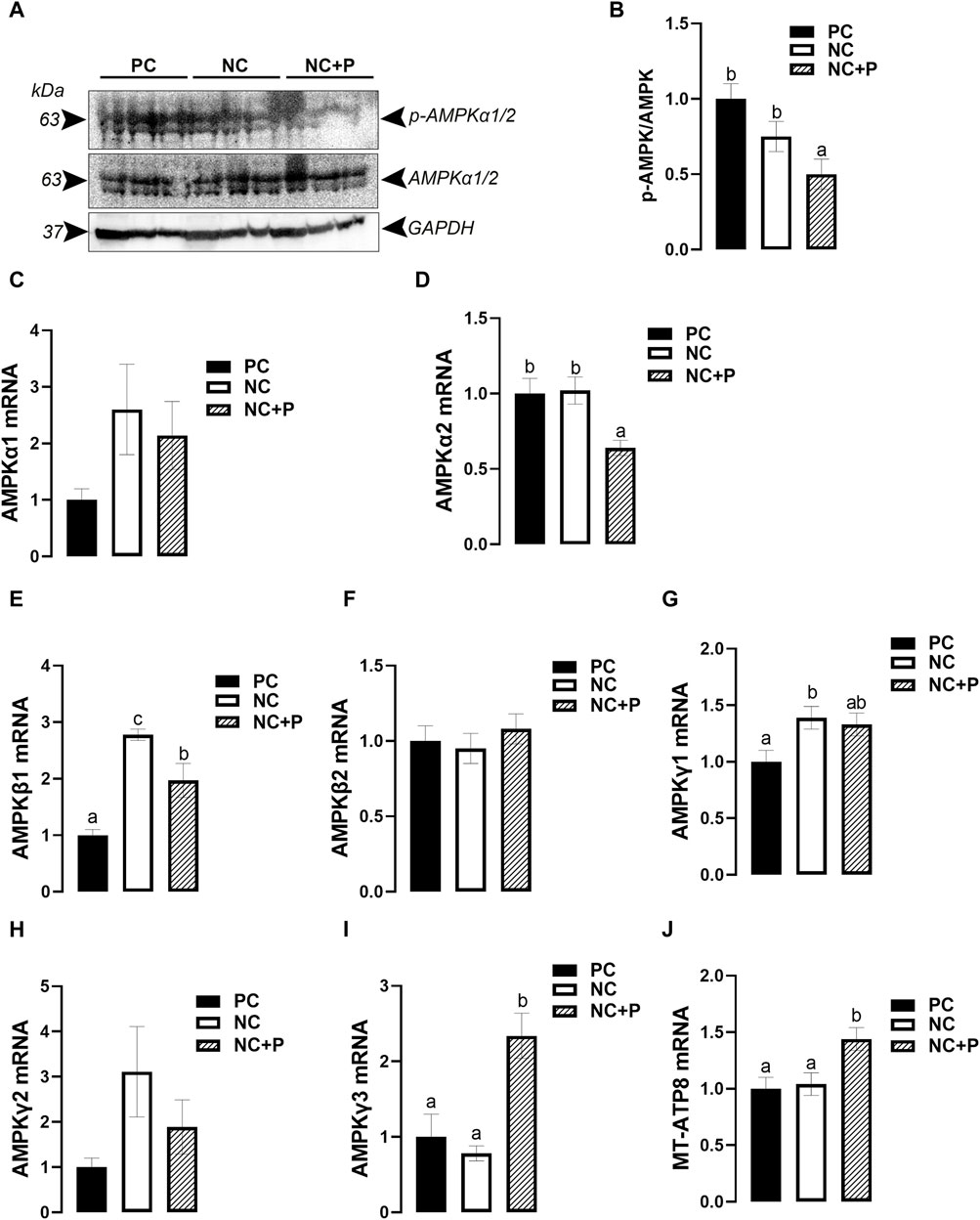
FIGURE 5. Effect of phytase supplementation on the expression of AMPK subunits in broiler breast muscle. The levels of pan and phosphorylated AMPKα1/2 at Thr172 site were determined by Western blot analysis (A,B). mRNA abundances of AMPKα1 I, AMPKα2 (D), AMPKI (E), AMPKβ2 (F), AMPKγ1 (G), AMPKγ2 (H), AMPKγ3 (I), and MT-ATP8 (J) were measured by real-time quantitative PCR and analyzed by 2−ΔΔCt method (Schmittgen and Livak, 2008). Data are presented as mean ± SEM (n = 6 birds/group). Different letters indicate significant difference at p < 0.05. AMPK, adenosine monophosphate (AMP)-activated protein kinase; MT-ATP8, mitochondrial ATP synthase F0 subunit 8; NC, negative control; NC + P, negative control supplemented with 2,000 phytase units/kg (FYT per kg of feed); PC, positive control.
Phytase supplementation significantly increased the levels of phosphorylated mTOR and P70S6K at Ser2448 and Thr389 sites, respectively, compared to the other groups (Figures 6A, B). However, the activity of GSK-3β remained unchanged between all tested groups (Figures 6A, B). At mRNA levels, phytase supplementation significantly induced the expression of mTOR and RPS6K, but it reduced that of EiF2α compared to PC and NC diets (Figures 6C–E). The expression of GSK3β and EiF4BP1 was significantly upregulated in NC compared to PC and NC + P birds (Figures 6F, G). GCN2 mRNA levels did not change in all groups (Figure 6H).
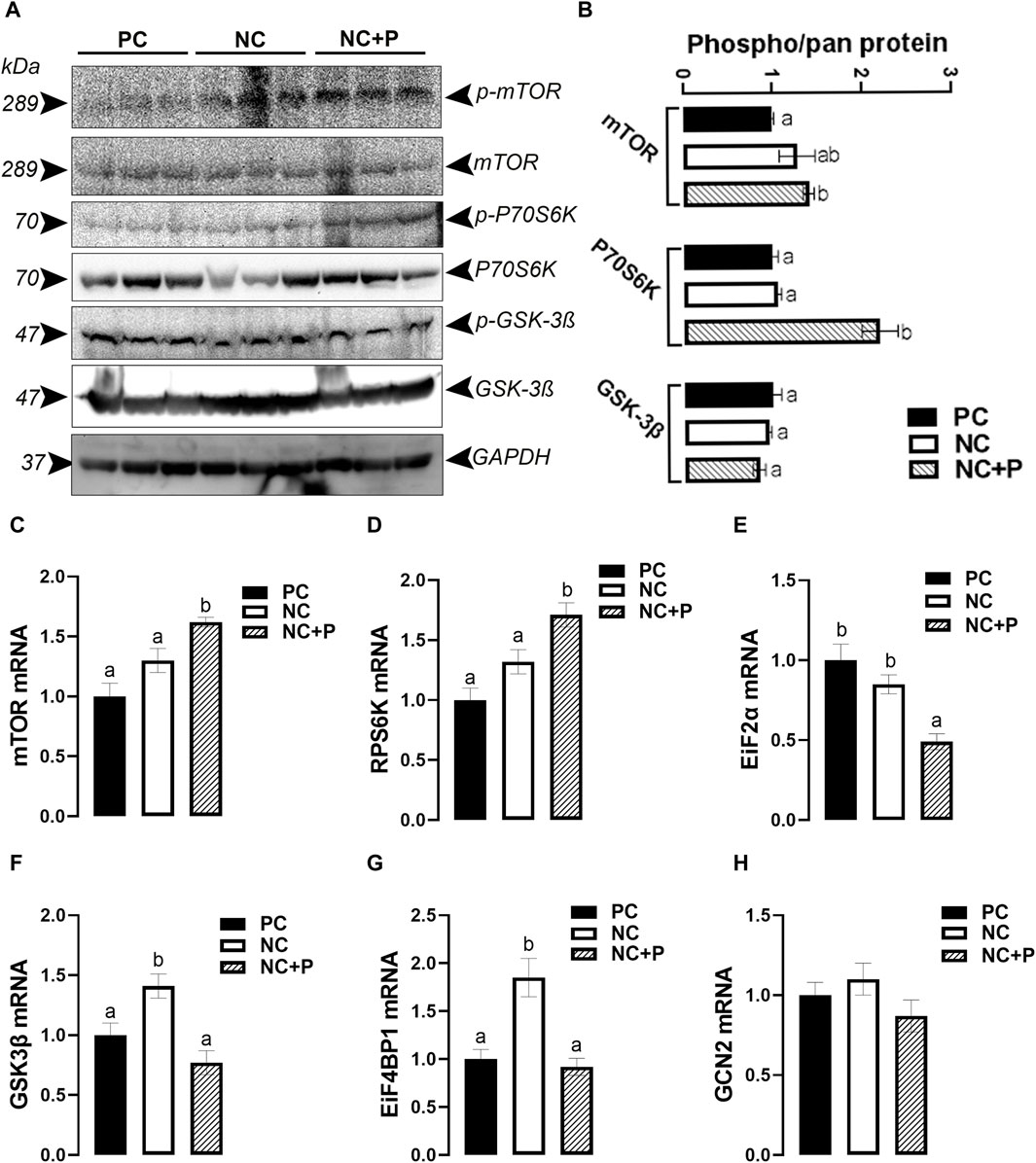
FIGURE 6. Effect of phytase supplementation on the expression of key genes and proteins involved in breast muscle protein synthesis. The levels of pan and phosphorylated mTORSer2448, P70S6KThr389, and GSK-3βSer9 were determined by Western blot analysis (A,B). mRNA abundances of mTOR (C), RPS6K (D), EiF2α (E), GSK-3β (F), EiF4BP1 (G), and GCN2 (H) were measured by real-time quantitative PCR and analyzed by 2−ΔΔCt method (Schmittgen and Livak, 2008). Data are presented as mean ± SEM (n = 6 birds/group). Different letters indicate significant difference at p < 0.05. GCN2, general control nonderepressible 2 or eukaryotic translation initiation factor 2 alpha kinase 4; EiF2α, eukaryotic translation initiation factor 2 subunit alpha; EiF4BP1, eukaryotic translation initiation factor 4E binding protein 1; GSK-3β, glycogen synthase kinase 3 beta; mTOR, mechanistic target of rapamycin; P70S6K, ribosomal protein S6 kinase.
Discussion
The global poultry sector soared from 9 to 133 million tonnes of meat and mounted from 15 to 93 million tonnes of eggs between 1961 and 2020 (Nations and Rome, 2023) and continues to support the livelihoods of billions of people worldwide. However, muscle myopathies, including WB and WS incidence, are emerging on a global scale and imposing heavy welfare and food security burdens on the poultry industry worldwide (Mudalal et al., 2015; Trocino et al., 2015), accounting for more than $1 billion annual economic loss in North America alone (Barbut, 2020). Although their etiologies are still not well defined, breast fillets affected by these myopathies share super-imposed and overlapping histological hallmarks, which are typically characterized by myo-degeneration and necrosis, infiltration of inflammatory cells in the endomysium, and accumulation of connective tissues and fat (Sihvo et al., 2014; Baldi et al., 2018).
Despite their rising incidence and associated complications, mechanistic understanding of these myopathies remains limited. In that regard, several omics studies (Mutryn et al., 2015; Abasht et al., 2016; Kuttappan et al., 2017) and recent functional evidence suggested that hypoxia is a key causative factor for WB (Emami et al., 2021) and that in-feed supplementation of phytase improved growth performance and reduced WB severity (Greene et al., 2019). Building on these previous studies and in a continuum of the same research hot spot, we report here that addition of a new fourth generation phytase improves growth performances (BW, weight corrected FCR, and carcass cut up) and reduces the severity of WB without affecting WS incidence and meat quality attributes (pH and colors) in Ross 708 broilers. This novel phytase was encoded by a 6-phytase gene from Citrobacter braakii (ATCC51113), expressed in Aspergillus oryzae, and characterized by an optimal enzyme activity and intrinsic temperature- and pH-stability (Imtiaz, 2022; Zhang et al., 2022). The improvement of growth performance observed here is not surprising and it is in agreement with previous investigations (Zhang et al., 2022). However, it is worth noting that compared to the previous study where the effect of dietary phytase on growth was reported to be minimal in older birds (d28-36), here the present study showed that the effect was significant in all rearing phases (ages). Although further studies are needed, the abovementioned differential age-dependent effects were probably associated with the experimental duration (36 days vs. 55 days), conditions and location (China or France vs. United States and cage vs. floor pens), diet composition and nutrient specifications, and/or broiler strains (Cobb 500 and Ross 308 vs. Ross 708) in the previous (Zhang et al., 2022) and in the present experiment, respectively.
In attempt to delineate the mode of action of the novel phytase, and as WB myopathy has been predicted to be associated with carbohydrate dysmetabolism, we next measured blood glucose concentrations using iSTAT Alinity. Interestingly, phytase supplementation decreased circulating glucose levels compared to both NC and PC diets, which suggests an increased glucose uptake by the breast muscle and probably by other glucose-absorbing tissues. The upregulation of GLUT (GLUT1, 6, and 10) gene expression and GLUT1-and GLUT12-protein levels in our experimental conditions supports the abovementioned hypothesis that the novel phytase enhances glucose uptake by the breast muscle (Cowieson et al., 2013; Lu et al., 2019). This has been supported by enriched GLUT1 and GLUT12 proteins in membrane fractions (data not shown). However, it is intriguing and still unknown how and why the other GLUTs (GLUT3, GLUT8, and GLUT12) were induced in the breast muscle of NC birds. As they belong to different classes (I to III) (Olson and Pessin, 1996; Joost and Thorens, 2001), it is possible that these GLUTs have different and/or additional functions and affinities. For instance, GLUT3 has been reported to transport maltose, xylose, dehydroascorbic acid, mannose, and galactose in mammals (Colville et al., 1993). GLUT12, on the other hand, has been shown to be involved in urate transport in rodents (Toyoda et al., 2020). As the glycogen debranching enzyme (AGL) expression was upregulated by the NC diet, it is probable that the activated glycogenolysis pathway might directly or indirectly regulate these GLUTs (Fidler et al., 2017). Furthermore, as we used the whole muscle tissue, it is plausible that the expression of these GLUTs is cell-, compartment-, and/or fiber-specific, and therefore they respond differently to stimuli [for review see (Pyla et al., 2013)].
After transport and uptake, the cellular fate of glucose begins with phosphorylation and then undergoes subsequent utilization pathways, including glycolysis, glycogen formation, or conversion to other intermediates in the hexose phosphate or hexosamine biosynthesis pathways, depending on the expression of isozymic forms of HKs (Bouche et al., 2004). Here, real-time qPCR analyses showed that both GK (also known as HK4) and HK1-3 are expressed in the muscle, corroborating a previous study in chickens (Roy et al., 2013). In human, however, GK expression has been shown to be restricted to the pancreas and the liver (Matschinsky, 1990). Selective expression/regulation of HK isozymic forms, differing in catalytic and regulatory properties as well as subcellular localization, is likely to be an important factor in determining the pattern of glucose metabolism. In fact, despite their overall structural similarity, HK1 (type I) serves for catabolic function for energy production, however HK2 (Type II) and probably HK3 (Type III) isozymes are suggested to serve primarily anabolic functions (Wilson, 2003). The upregulation of GK at both mRNA and protein levels suggests that phytase supplementation induces muscle glycolysis and ATP synthesis, which is supported by the upregulation of MT-ATP8 gene expression and the downregulation of the catalytic AMPKα1/2 subunit gene and its phosphorylated levels at Thr172 site. MT-ATP8 encodes for the mitochondrial ATP synthase (complex V), which is an important enzyme that provides energy to be used by the cell through the ATP synthesis (Jonckheere et al., 2012). AMPK is a conserved master cellular “fuel gauge” and metabolic sensor that is activated by falling energy status, but downregulated when the cellular ATP/AMP ratio is elevated (Hardie et al., 2012; Gowans and Hardie, 2014; Garcia and Shaw, 2017). In most species, AMPK exists as an obligate heterotrimer, containing catalytic subunits (α1 and α2), and two regulatory β (β1 and β2) and γ (γ1, γ2, and γ3) subunits, which are encoded by different genes (Hardie, 2007). The upregulation of both AMPKβ1 and AMPKγ3 subunits by phytase suggests that the downregulation of the catalytic AMPKα1/2 was probably mediated by ATP-binding to these regulatory subunits (Scott et al., 2004). However, it is still unclear why and how the NC diet induces muscle AMPKβ1 and AMPKγ1 expression in our experimental conditions, and the mechanism likely involves calcium flux that remains a critical underexplored area for future investigations (Dunn and Munger, 2020).
The increased level of cellular energy (ATP), described above, can be used for anabolic pathways. As there was no difference in fat content in our experimental conditions, we limited our analyses to glycogen- and protein-biosynthesis. The increased abundance of glycogen synthases (GYS1 and GYS2) mRNA indicates that the supplementation of phytase enhances muscle glycogen synthesis. However, the downregulation of both glycogen branching and debranching enzyme expression suggests that both glycogenolysis and glycogenesis are concomitant, a phenomenon known as glycogen cycling (Landau, 2001). Importantly, it has been shown that AMPKγ3 mutation increased glycogen content and decreased triglyceride in human skeletal muscle (Costford et al., 2007), which suggests that phytase supplementation might regulate breast muscle glycogen and energy homeostasis via AMPKγ3-AMPKα1/2 interaction.
The enhanced BW along with increased part (breast, wing, tender) weights suggest that phytase supplementation enhances global and local (breast muscle) protein synthesis, which is supported by the upregulation of mTOR-RPS6K and the downregulation of EIF-4BP1 and EIF2α expression (Schmeisser et al., 2017). mTOR is a conserved serine/threonine kinase and nutrient sensor (Kim et al., 2002), that belongs to the PI3K-related protein kinase (PIKK) family (Richardson et al., 2004) and plays a key role in ribosomal biogenesis and protein synthesis (Zhang et al., 2014). The well-studied and best-characterized mTOR-downstream cascade is the translation regulator 4E-BP1-S6K1, both of which contain TOR signaling (TOS) motifs (Nojima et al., 2003; Schalm et al., 2003). 4E-BP1 is a translational repressor that inhibits translation initiation by interfering with the interaction between the cap-binding protein eIF4E and eIF4G1 (Hara et al., 1997; Zhang et al., 2010; Thoreen et al., 2012). Activation of EIF2α attenuates global protein synthesis, except the translation of select mRNA such as the activating transcription factor 4 (ATF4) (Harding et al., 2003; Nobukuni et al., 2005). Unlike its inhibitory effect on 4E-BP1 and EIF2α, mTOR activates P70S6K, which in turn, regulates ribosome function, mRNA processing, cap-dependent translation initiation and elongation, and then promotes several cellular processes, including protein production and cell size/growth (Price et al., 1992; Isotani et al., 1999; Magnuson et al., 2012). Although the exact mechanism by which phytase supplementation activates mTOR is not known at this time point, it is possible that the effect is mediated through high amino acid digestibility, absorption, and utilization (Nobukuni et al., 2005; Zoncu et al., 2011; Manifava et al., 2016). Indeed, exogenous phytase fed at high dose has been reported to induce a rapid and complete breakdown of phytate in diets, reducing the anti-nutritional effects, and thereby eliciting a greater amino acid utilization (Amerah et al., 2014; Sommerfeld et al., 2018; Siegert et al., 2021). Moreover, it is plausible that phytase activates mTOR via available phosphorus-stimulating AKT pathway (Simons et al., 1990; Jin et al., 2009; Liu et al., 2017). Although it was not functionally demonstrated here, it is very likely that phytase activates mTOR via glucose-AMPK pathway (Leprivier and Rotblat, 2020; Rumala et al., 2020; Caligaris et al., 2023), which merits future in-depth investigation. It is logical also to hypothesize that phytase supplementation might activate mTOR pathway via increased inositol (Kim et al., 2011; Badodi et al., 2021) and/or improvement of oxygen homeostasis (Miloslavski et al., 2014; Greene et al., 2019; Zhu et al., 2020), that warrants further consideration.
In summary, this is the first report, to the best of our knowledge, showing potential new mechanisms evolving glucose metabolism and AMPK-mTOR pathway, by which the novel phytase improves growth and reduces WB myopathy. This opens a new vista and provides a framework for future in-depth mechanistic research using different chicken strains.
Data availability statement
The original contributions presented in the study are included in the article/Supplementary Material, further inquiries can be directed to the corresponding author.
Ethics statement
Animal experiments were performed in accordance with the Animal Research: Reporting of In Vivo Experiments (ARRIVE, https://www.nc3rs.org.uk/arrive-guidelines). The present study was conducted in accordance with the recommendations in the guide for the care and use of laboratory animals of the National Institutes of Health and the protocols were approved by the University of Arkansas Animal Care and Use Committee under protocol # 21050.
Author contributions
CW: Funding acquisition, Writing–review and editing, Project administration. GM: Writing–review and editing, Investigation. CrM: Investigation, Writing–review and editing, Methodology. EG: Writing–review and editing. ClM: Writing–review and editing, Investigation, Methodology. NW: Investigation, Writing–review and editing, Funding acquisition. SD: Funding acquisition, Writing–review and editing, Conceptualization, Supervision, Visualization, Writing–original draft.
Funding
The author(s) declare financial support was received for the research, authorship, and/or publication of this article. This study was supported by a grant from DSM Animal Nutrition, Kaiseraugst, Switzerland (FY2022-2023 to SD). DSM Animal Nutrition had no role in conducting the research, generating the data, interpreting the results, or writing the manuscript.
Acknowledgments
The authors would like to thank Sara Orlowski, Travis Tabler, Alison Ramser, Maricela Maqueda, Kirsten Shafer, Blake Nelson, Savannah Crafton, Clay Maynard, the University of Arkansas Poultry Research farm, the University of Arkansas feed mill, and the University of Arkansas pilot processing plant staff. In addition, thanks to Montana Oubre, Brooke Jasek, and Thomas Frost from DSM Nutritional Products, United States.
Conflict of interest
Authors Carrie L. Walk and Nelson Ward were employed by DSM Nutritional Products.
The remaining authors declare that the research was conducted in the absence of any commercial or financial relationships that could be construed as a potential conflict of interest.
The author(s) declared that they were an editorial board member of Frontiers, at the time of submission. This had no impact on the peer review process and the final decision.
Publisher’s note
All claims expressed in this article are solely those of the authors and do not necessarily represent those of their affiliated organizations, or those of the publisher, the editors and the reviewers. Any product that may be evaluated in this article, or claim that may be made by its manufacturer, is not guaranteed or endorsed by the publisher.
Supplementary material
The Supplementary Material for this article can be found online at: https://www.frontiersin.org/articles/10.3389/fphys.2024.1376628/full#supplementary-material
References
Abasht B., Mutryn M. F., Michalek R. D., Lee W. R. (2016). Oxidative stress and metabolic perturbations in wooden breast disorder in chickens. PLoS One 11 (4), e0153750. doi:10.1371/journal.pone.0153750
Amerah A. M., Plumstead P. W., Barnard L. P., Kumar A. (2014). Effect of calcium level and phytase addition on ileal phytate degradation and amino acid digestibility of broilers fed corn-based diets. Poult. Sci. 93 (4), 906–915. doi:10.3382/ps.2013-03465
Badodi S., Pomella N., Zhang X., Rosser G., Whittingham J., Niklison-Chirou M. V., et al. (2021). Inositol treatment inhibits medulloblastoma through suppression of epigenetic-driven metabolic adaptation. Nat. Commun. 12 (1), 2148. doi:10.1038/s41467-021-22379-7
Baldi G., Soglia F., Mazzoni M., Sirri F., Canonico L., Babini E., et al. (2018). Implications of white striping and spaghetti meat abnormalities on meat quality and histological features in broilers. Animal 12 (1), 164–173. doi:10.1017/S1751731117001069
Barbut S. (2020). “Understanding the woody breast syndrome and other myopathies in modern broiler chickens,” in 1st annual Australian poultry science symposium (Sydney, Australia: The Poultry Research Foundation).
Bauermeister L. J., Morey A. U., Moran E. T., Singh M., Owens C. M., McKee S. R. (2009). Occurrence of WS in chicken breast fillets in relation to broiler size. Poult. Sci. 88, 104.
Bilgili S. F., Meloche K. J., Campasino A., Dozier W. A. (2014). He influence of carnitine and guanidinoacetic acid supplementation of low and high amino acid density diets on Pectoralis major myopathies in broiler chickens. Poult. Sci. 93, M56.
Blankenship K., Gilley A., Piekarski A., Orlowski S., Greene E., Bottje W., et al. (2016). Differential expression of feeding-related hypothalamic neuropeptides in the first generation of quails divergently selected for low or high feed efficiency. Neuropeptides 58, 31–40. doi:10.1016/j.npep.2015.12.007
Bouche C., Serdy S., Kahn C. R., Goldfine A. B. (2004). The cellular fate of glucose and its relevance in type 2 diabetes. Endocr. Rev. 25 (5), 807–830. doi:10.1210/er.2003-0026
Caligaris M., Nicastro R., Hu Z., Tripodi F., Hummel J. E., Pillet B., et al. (2023). Snf1/AMPK fine-tunes TORC1 signaling in response to glucose starvation. Elife 12, e84319. doi:10.7554/eLife.84319
Cauble R. N., Greene E. S., Orlowski S., Walk C., Bedford M., Apple J., et al. (2020). Research Note: dietary phytase reduces broiler woody breast severity via potential modulation of breast muscle fatty acid profiles. Poult. Sci. 99 (8), 4009–4015. doi:10.1016/j.psj.2020.05.005
Cavani C., Petracci M., Trocino A., Xiccato G. (2009). Advances in research on poultry and rabbit meat quality. Italian J. Animal Sci. 8, 741–750. doi:10.4081/ijas.2009.s2.741
Che S., Wang C., Varga C., Barbut S., Susta L. (2022). Prevalence of breast muscle myopathies (spaghetti meat, woody breast, white striping) and associated risk factors in broiler chickens from Ontario Canada. PLoS One 17 (4), e0267019. doi:10.1371/journal.pone.0267019
Colville C. A., Seatter M. J., Jess T. J., Gould G. W., Thomas H. M. (1993). Kinetic analysis of the liver-type (GLUT2) and brain-type (GLUT3) glucose transporters in Xenopus oocytes: substrate specificities and effects of transport inhibitors. Biochem. J. 290 (3), 701–706. doi:10.1042/bj2900701
Costford S. R., Kavaslar N., Ahituv N., Chaudhry S. N., Schackwitz W. S., Dent R., et al. (2007). Gain-of-function R225W mutation in human AMPKgamma(3) causing increased glycogen and decreased triglyceride in skeletal muscle. PLoS One 2 (9), e903. doi:10.1371/journal.pone.0000903
Cowieson A. J., Ptak A., Mackowiak P., Sassek M., Pruszynska-Oszmalek E., Zyla K., et al. (2013). The effect of microbial phytase and myo-inositol on performance and blood biochemistry of broiler chickens fed wheat/corn-based diets. Poult. Sci. 92 (8), 2124–2134. doi:10.3382/ps.2013-03140
Dridi S., Hirano Y., Tarallo V., Kim Y., Fowler B. J., Ambati B. K., et al. (2012). ERK1/2 activation is a therapeutic target in age-related macular degeneration. Proc. Natl. Acad. Sci. U. S. A. 109 (34), 13781–13786. doi:10.1073/pnas.1206494109
Dunn D. M., Munger J. (2020). Interplay between calcium and AMPK signaling in human cytomegalovirus infection. Front. Cell Infect. Microbiol. 10, 384. doi:10.3389/fcimb.2020.00384
Emami N. K., Cauble R. N., Dhamad A. E., Greene E. S., Coy C. S., Velleman S. G., et al. (2021). Hypoxia further exacerbates woody breast myopathy in broilers via alteration of satellite cell fate. Poult. Sci. 100 (7), 101167. doi:10.1016/j.psj.2021.101167
Fidler T. P., Middleton E. A., Rowley J. W., Boudreau L. H., Campbell R. A., Souvenir R., et al. (2017). Glucose transporter 3 potentiates degranulation and is required for platelet activation. Arterioscler. Thromb. Vasc. Biol. 37 (9), 1628–1639. doi:10.1161/ATVBAHA.117.309184
Garcia D., Shaw R. J. (2017). AMPK: mechanisms of cellular energy sensing and restoration of metabolic balance. Mol. Cell 66 (6), 789–800. doi:10.1016/j.molcel.2017.05.032
Gonzalez-Uarquin F., Kenez A., Rodehutscord M., Huber K. (2020). Dietary phytase and myo-inositol supplementation are associated with distinct plasma metabolome profile in broiler chickens. Animal 14 (3), 549–559. doi:10.1017/S1751731119002337
Gowans G. J., Hardie D. G. (2014). AMPK: a cellular energy sensor primarily regulated by AMP. Biochem. Soc. Trans. 42 (1), 71–75. doi:10.1042/BST20130244
Greene E., Cauble R., Dhamad A. E., Kidd M. T., Kong B., Howard S. M., et al. (2020). Muscle metabolome profiles in woody breast-(un)Affected broilers: effects of quantum blue phytase-enriched diet. Front. Vet. Sci. 7, 458. doi:10.3389/fvets.2020.00458
Greene E., Flees J., Dadgar S., Mallmann B., Orlowski S., Dhamad A., et al. (2019). Quantum blue reduces the severity of woody breast myopathy via modulation of oxygen homeostasis-related genes in broiler chickens. Front. Physiol. 10, 1251. doi:10.3389/fphys.2019.01251
Greene E. S., Maynard C., Mullenix G., Bedford M., Dridi S. (2023). Potential role of endoplasmic reticulum stress in broiler woody breast myopathy. Am. J. Physiol. Cell Physiol. 324 (3), C679–C693. doi:10.1152/ajpcell.00275.2022
Hara K., Yonezawa K., Kozlowski M. T., Sugimoto T., Andrabi K., Weng Q. P., et al. (1997). Regulation of eIF-4E BP1 phosphorylation by mTOR. J. Biol. Chem. 272 (42), 26457–26463. doi:10.1074/jbc.272.42.26457
Hardie D. G. (2007). AMP-activated/SNF1 protein kinases: conserved guardians of cellular energy. Nat. Rev. Mol. Cell Biol. 8 (10), 774–785. doi:10.1038/nrm2249
Hardie D. G., Ross F. A., Hawley S. A. (2012). AMPK: a nutrient and energy sensor that maintains energy homeostasis. Nat. Rev. Mol. Cell Biol. 13 (4), 251–262. doi:10.1038/nrm3311
Harding H. P., Zhang Y., Zeng H., Novoa I., Lu P. D., Calfon M., et al. (2003). An integrated stress response regulates amino acid metabolism and resistance to oxidative stress. Mol. Cell 11 (3), 619–633. doi:10.1016/s1097-2765(03)00105-9
Imtiaz Z. (2022). “DSM launches 4th generation phytase in Korea,” in AsianAgribiz. (Singapore: Asian Agribusiness Media PTE LTD).
Isotani S., Hara K., Tokunaga C., Inoue H., Avruch J., Yonezawa K. (1999). Immunopurified mammalian target of rapamycin phosphorylates and activates p70 S6 kinase alpha in vitro. J. Biol. Chem. 274 (48), 34493–34498. doi:10.1074/jbc.274.48.34493
Jin H., Xu C. X., Lim H. T., Park S. J., Shin J. Y., Chung Y. S., et al. (2009). High dietary inorganic phosphate increases lung tumorigenesis and alters Akt signaling. Am. J. Respir. Crit. Care Med. 179 (1), 59–68. doi:10.1164/rccm.200802-306OC
Jonckheere A. I., Smeitink J. A., Rodenburg R. J. (2012). Mitochondrial ATP synthase: architecture, function and pathology. J. Inherit. Metab. Dis. 35 (2), 211–225. doi:10.1007/s10545-011-9382-9
Joost H. G., Thorens B. (2001). The extended GLUT-family of sugar/polyol transport facilitators: nomenclature, sequence characteristics, and potential function of its novel members (review). Mol. Membr. Biol. 18 (4), 247–256. doi:10.1080/09687680110090456
Kim D. H., Sarbassov D. D., Ali S. M., King J. E., Latek R. R., Erdjument-Bromage H., et al. (2002). mTOR interacts with raptor to form a nutrient-sensitive complex that signals to the cell growth machinery. Cell 110 (2), 163–175. doi:10.1016/s0092-8674(02)00808-5
Kim S., Kim S. F., Maag D., Maxwell M. J., Resnick A. C., Juluri K. R., et al. (2011). Amino acid signaling to mTOR mediated by inositol polyphosphate multikinase. Cell Metab. 13 (2), 215–221. doi:10.1016/j.cmet.2011.01.007
Kuttappan V. A., Bottje W., Ramnathan R., Hartson S. D., Coon C. N., Kong B. W., et al. (2017). Proteomic analysis reveals changes in carbohydrate and protein metabolism associated with broiler breast myopathy. Poult. Sci. 96 (8), 2992–2999. doi:10.3382/ps/pex069
Kuttappan V. A., Shivaprasad H. L., Shaw D. P., Valentine B. A., Hargis B. M., Clark F. D., et al. (2013). Pathological changes associated with white striping in broiler breast muscles. Poult. Sci. 92 (2), 331–338. doi:10.3382/ps.2012-02646
Landau B. R. (2001). Methods for measuring glycogen cycling. Am. J. Physiol. Endocrinol. Metab. 281 (3), E413–E419. doi:10.1152/ajpendo.2001.281.3.E413
Leprivier G., Rotblat B. (2020). How does mTOR sense glucose starvation? AMPK is the usual suspect. Cell Death Discov. 6, 27. doi:10.1038/s41420-020-0260-9
Liu N. N., Flanagan P. R., Zeng J., Jani N. M., Cardenas M. E., Moran G. P., et al. (2017). Phosphate is the third nutrient monitored by TOR in Candida albicans and provides a target for fungal-specific indirect TOR inhibition. Proc. Natl. Acad. Sci. U. S. A. 114 (24), 6346–6351. doi:10.1073/pnas.1617799114
Lu H., Kuhn I., Bedford M. R., Whitfield H., Brearley C., Adeola O., et al. (2019). Effect of phytase on intestinal phytate breakdown, plasma inositol concentrations, and glucose transporter type 4 abundance in muscle membranes of weanling pigs1. J. Anim. Sci. 97 (9), 3907–3919. doi:10.1093/jas/skz234
Magnuson B., Ekim B., Fingar D. C. (2012). Regulation and function of ribosomal protein S6 kinase (S6K) within mTOR signalling networks. Biochem. J. 441 (1), 1–21. doi:10.1042/BJ20110892
Manifava M., Smith M., Rotondo S., Walker S., Niewczas I., Zoncu R., et al. (2016). Dynamics of mTORC1 activation in response to amino acids. Elife 5, e19960. doi:10.7554/eLife.19960
Marangoni F., Corsello G., Cricelli C., Ferrara N., Ghiselli A., Lucchin L., et al. (2015). Role of poultry meat in a balanced diet aimed at maintaining health and wellbeing: an Italian consensus document. Food Nutr. Res. 59, 27606. doi:10.3402/fnr.v59.27606
Martin M. P., Wineland M., Barnes H. J. (2010). Selected blood chemistry and gas reference ranges for broiler breeders using the i-STAT handheld clinical analyzer. Avian Dis. 54 (3), 1016–1020. doi:10.1637/9223-122209-Reg.1
Matschinsky F. M. (1990). Glucokinase as glucose sensor and metabolic signal generator in pancreatic beta-cells and hepatocytes. Diabetes 39 (6), 647–652. doi:10.2337/diab.39.6.647
Miloslavski R., Cohen E., Avraham A., Iluz Y., Hayouka Z., Kasir J., et al. (2014). Oxygen sufficiency controls TOP mRNA translation via the TSC-Rheb-mTOR pathway in a 4E-BP-independent manner. J. Mol. Cell Biol. 6 (3), 255–266. doi:10.1093/jmcb/mju008
Mudalal S., Lorenzi M., Soglia F., Cavani C., Petracci M. (2015). Implications of white striping and wooden breast abnormalities on quality traits of raw and marinated chicken meat. Animal 9 (4), 728–734. doi:10.1017/S175173111400295X
Mutryn M. F., Brannick E. M., Fu W., Lee W. R., Abasht B. (2015). Characterization of a novel chicken muscle disorder through differential gene expression and pathway analysis using RNA-sequencing. BMC Genomics 16 (1), 399. doi:10.1186/s12864-015-1623-0
Nguyen P., Greene E., Ishola P., Huff G., Donoghue A., Bottje W., et al. (2015). Chronic mild cold conditioning modulates the expression of hypothalamic neuropeptide and intermediary metabolic-related genes and improves growth performances in young chicks. PLoS One 10 (11), e0142319. doi:10.1371/journal.pone.0142319
Nobukuni T., Joaquin M., Roccio M., Dann S. G., Kim S. Y., Gulati P., et al. (2005). Amino acids mediate mTOR/raptor signaling through activation of class 3 phosphatidylinositol 3OH-kinase. Proc. Natl. Acad. Sci. U. S. A. 102 (40), 14238–14243. doi:10.1073/pnas.0506925102
Nojima H., Tokunaga C., Eguchi S., Oshiro N., Hidayat S., Yoshino K., et al. (2003). The mammalian target of rapamycin (mTOR) partner, raptor, binds the mTOR substrates p70 S6 kinase and 4E-BP1 through their TOR signaling (TOS) motif. J. Biol. Chem. 278 (18), 15461–15464. doi:10.1074/jbc.C200665200
Olson A. L., Pessin J. E. (1996). Structure, function, and regulation of the mammalian facilitative glucose transporter gene family. Annu. Rev. Nutr. 16, 235–256. doi:10.1146/annurev.nu.16.070196.001315
Price D. J., Grove J. R., Calvo V., Avruch J., Bierer B. E. (1992). Rapamycin-induced inhibition of the 70-kilodalton S6 protein kinase. Science 257 (5072), 973–977. doi:10.1126/science.1380182
Pyla R., Poulose N., Jun J. Y., Segar L. (2013). Expression of conventional and novel glucose transporters, GLUT1, -9, -10, and -12, in vascular smooth muscle cells. Am. J. Physiol. Cell Physiol. 304 (6), C574–C589. doi:10.1152/ajpcell.00275.2012
Richardson C. J., Schalm S. S., Blenis J. (2004). PI3-kinase and TOR: PIKTORing cell growth. Semin. Cell Dev. Biol. 15 (2), 147–159. doi:10.1016/j.semcdb.2003.12.023
Roy T. K., Iwasawa A., Shimizu Y., Kageyama K., Yoshizaki N. (2013). Ontogenic profile of hexokinase and glucokinase mRNA expressions in embryonic chicken liver and muscle. J. Poult. Sci. 50, 270–274. doi:10.2141/jpsa.0120156
Rumala C. Z., Liu J., Locasale J. W., Corkey B. E., Deeney J. T., Rameh L. E. (2020). Exposure of pancreatic β-cells to excess glucose results in bimodal activation of mTORC1 and mTOR-dependent metabolic acceleration. iScience 23 (2), 100858. doi:10.1016/j.isci.2020.100858
Rutherfurd S. M., Chung T. K., Thomas D. V., Zou M. L., Moughan P. J. (2012). Effect of a novel phytase on growth performance, apparent metabolizable energy, and the availability of minerals and amino acids in a low-phosphorus corn-soybean meal diet for broilers. Poult. Sci. 91 (5), 1118–1127. doi:10.3382/ps.2011-01702
Schaal T. P., Arango J., Wolc A., Brady J. V., Fulton J. E., Rubinoff I., et al. (2016). Commercial Hy-Line W-36 pullet and laying hen venous blood gas and chemistry profiles utilizing the portable i-STAT®1 analyzer. Poult. Sci. 95 (2), 466–471. doi:10.3382/ps/pev350
Schalm S. S., Fingar D. C., Sabatini D. M., Blenis J. (2003). TOS motif-mediated raptor binding regulates 4E-BP1 multisite phosphorylation and function. Curr. Biol. 13 (10), 797–806. doi:10.1016/s0960-9822(03)00329-4
Schmeisser J., Seon A. A., Aureli R., Friedel A., Guggenbuhl P., Duval S., et al. (2017). Exploratory transcriptomic analysis in muscle tissue of broilers fed a phytase-supplemented diet. J. Anim. Physiol. Anim. Nutr. Berl. 101 (3), 563–575. doi:10.1111/jpn.12482
Schmittgen T. D., Livak K. J. (2008). Analyzing real-time PCR data by the comparative C(T) method. Nat. Protoc. 3 (6), 1101–1108. doi:10.1038/nprot.2008.73
Scott J. W., Hawley S. A., Green K. A., Anis M., Stewart G., Scullion G. A., et al. (2004). CBS domains form energy-sensing modules whose binding of adenosine ligands is disrupted by disease mutations. J. Clin. Invest. 113 (2), 274–284. doi:10.1172/JCI19874
Shirley R. B., Edwards H. M. (2003). Graded levels of phytase past industry standards improves broiler performance. Poult. Sci. 82 (4), 671–680. doi:10.1093/ps/82.4.671
Siegert W., Krieg J., Sommerfeld V., Borda-Molina D., Feuerstein D., Camarinha-Silva A., et al. (2021). Phytase supplementation effects on amino acid digestibility in broiler chickens are influenced by dietary calcium concentrations but not by acid-binding capacity. Curr. Dev. Nutr. 5 (8), nzab103. doi:10.1093/cdn/nzab103
Sihvo H. K., Immonen K., Puolanne E. (2014). Myodegeneration with fibrosis and regeneration in the pectoralis major muscle of broilers. Vet. Pathol. 51 (3), 619–623. doi:10.1177/0300985813497488
Simons P. C., Versteegh H. A., Jongbloed A. W., Kemme P. A., Slump P., Bos K. D., et al. (1990). Improvement of phosphorus availability by microbial phytase in broilers and pigs. Br. J. Nutr. 64 (2), 525–540. doi:10.1079/bjn19900052
Soglia F., Mudalal S., Babini E., Di Nunzio M., Mazzoni M., Sirri F., et al. (2016). Histology, composition, and quality traits of chicken Pectoralis major muscle affected by wooden breast abnormality. Poult. Sci. 95 (3), 651–659. doi:10.3382/ps/pev353
Sommerfeld V., Schollenberger M., Kuhn I., Rodehutscord M. (2018). Interactive effects of phosphorus, calcium, and phytase supplements on products of phytate degradation in the digestive tract of broiler chickens. Poult. Sci. 97 (4), 1177–1188. doi:10.3382/ps/pex404
Thoreen C. C., Chantranupong L., Keys H. R., Wang T., Gray N. S., Sabatini D. M. (2012). A unifying model for mTORC1-mediated regulation of mRNA translation. Nature 485 (7396), 109–113. doi:10.1038/nature11083
Toyoda Y., Takada T., Miyata H., Matsuo H., Kassai H., Nakao K., et al. (2020). Identification of GLUT12/SLC2A12 as a urate transporter that regulates the blood urate level in hyperuricemia model mice. Proc. Natl. Acad. Sci. U. S. A. 117 (31), 18175–18177. doi:10.1073/pnas.2006958117
Trocino A., Piccirillo A., Birolo M., Radaelli G., Bertotto D., Filiou E., et al. (2015). Effect of genotype, gender and feed restriction on growth, meat quality and the occurrence of white striping and wooden breast in broiler chickens. Poult. Sci. 94 (12), 2996–3004. doi:10.3382/ps/pev296
Vignale K., Caldas J. V., England J. A., Boonsinchai N., Magnuson A., Pollock E. D., et al. (2017). Effect of white striping myopathy on breast muscle (Pectoralis major) protein turnover and gene expression in broilers. Poult. Sci. 96 (4), 886–893. doi:10.3382/ps/pew315
Vignale K., Greene E. S., Caldas J. V., England J. A., Boonsinchai N., Sodsee P., et al. (2015). 25-Hydroxycholecalciferol enhances male broiler breast meat yield through the mTOR pathway. J. Nutr. 145 (5), 855–863. doi:10.3945/jn.114.207936
Wilson J. E. (2003). Isozymes of mammalian hexokinase: structure, subcellular localization and metabolic function. J. Exp. Biol. 206 (Pt 12), 2049–2057. doi:10.1242/jeb.00241
Zhang D., Contu R., Latronico M. V., Zhang J., Rizzi R., Catalucci D., et al. (2010). MTORC1 regulates cardiac function and myocyte survival through 4E-BP1 inhibition in mice. J. Clin. Invest. 120 (8), 2805–2816. doi:10.1172/JCI43008
Zhang Q., Walk C., Berti Sorbara J. O., Cowieson A. J., Stamatopoulos K. (2022). Comparative effects of two phytases on growth performance, bone mineralization, nutrient digestibility and phytate-P hydrolysis of broilers. J. Appl. Poult. Res. 31, 100247–100314. doi:10.1016/j.japr.2022.100247
Zhang Y., Nicholatos J., Dreier J. R., Ricoult S. J., Widenmaier S. B., Hotamisligil G. S., et al. (2014). Coordinated regulation of protein synthesis and degradation by mTORC1. Nature 513 (7518), 440–443. doi:10.1038/nature13492
Zhu C., Zhou Q., Luo C., Chen Y. (2020). Dexmedetomidine protects against oxygen-glucose deprivation-induced injury through inducing astrocytes autophagy via TSC2/mTOR pathway. Neuromolecular Med. 22 (2), 210–217. doi:10.1007/s12017-019-08576-0
Keywords: broilers, phytase, growth, glucose uptake, GLUTs, AMPK, mTOR
Citation: Walk CL, Mullenix GJ, Maynard CW, Greene ES, Maynard C, Ward N and Dridi S (2024) Novel 4th-generation phytase improves broiler growth performance and reduces woody breast severity through modulation of muscle glucose uptake and metabolism. Front. Physiol. 15:1376628. doi: 10.3389/fphys.2024.1376628
Received: 25 January 2024; Accepted: 29 February 2024;
Published: 15 March 2024.
Edited by:
Kent M. Reed, University of Minnesota Twin Cities, United StatesReviewed by:
Felix Kwame Amevor, Sichuan Agricultural University, ChinaElham Assadi Soumeh, The University of Queensland, Australia
Copyright © 2024 Walk, Mullenix, Maynard, Greene, Maynard, Ward and Dridi. This is an open-access article distributed under the terms of the Creative Commons Attribution License (CC BY). The use, distribution or reproduction in other forums is permitted, provided the original author(s) and the copyright owner(s) are credited and that the original publication in this journal is cited, in accordance with accepted academic practice. No use, distribution or reproduction is permitted which does not comply with these terms.
*Correspondence: Sami Dridi, ZHJpZGlAdWFyay5lZHU=
†Present addresses: Carrie L. Walk, AB Vista Woodstock Court, Marlborough, United Kingdom
Garrett J. Mullenix, Cargill Inc., Plymouth, MN, United States
Craig W. Maynard, Bell and Evans, Fredericksburg, PA, United States
Clay Maynard, Tyson Food, W. Don Tyson Parkway, Springdale, AR, United States