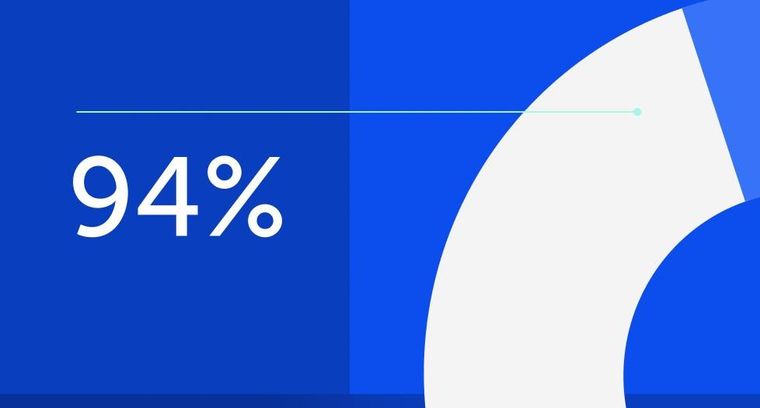
94% of researchers rate our articles as excellent or good
Learn more about the work of our research integrity team to safeguard the quality of each article we publish.
Find out more
MINI REVIEW article
Front. Physiol., 16 February 2024
Sec. Skin Physiology
Volume 15 - 2024 | https://doi.org/10.3389/fphys.2024.1367425
This article is part of the Research TopicTranslational Research in Skin RegenerationView all articles
Epithelial tissues, including the skin, are highly proliferative tissues with the capability to constant renewal and regeneration, a feature that is essential for survival as the skin forms a protective barrier against external insults and water loss. In adult mammalian skin, every injury will lead to a scar. The scar tissue that is produced to seal the wound efficiently is usually rigid and lacks elasticity and the skin’s original resilience to external impacts, but also secondary appendages such as hair follicles and sebaceous glands. While it was long thought that hair follicles develop solely during embryogenesis, it is becoming increasingly clear that hair follicles can also regenerate within a wound. The ability of the skin to induce hair neogenesis following injury however declines with age. As fetal and neonatal skin have the remarkable capacity to heal without scarring, the recapitulation of a neonatal state has been a primary target of recent regenerative research. In this review we highlight how modulating dermal signaling or the abundance of specific fibroblast subsets could be utilized to induce de novo hair follicles within the wound bed, and thus to shift wound repair with a scar to scarless regeneration.
Mammalian skin comprising the epidermis with its appendages - hair follicles (HFs), sebaceous and sweat glands - and the dermis, has evolved the remarkable ability to self-renew and repair itself upon injury (Fuchs, 2007; Watt and Fujiwara, 2011). However, every injury will lead to a scar. Amazingly, embryonic skin has the exceptional capability for scarless wound healing (Leavitt et al., 2016). Similarly, the formation of HFs from the developing epidermis, which depends on reciprocal signaling between cells of the epidermis and a condensate of mesenchymal cells of the underlying dermis which subsequently forms the dermal papilla of the HF, only occurs during embryonic or early postnatal development, but never in adult skin under homeostatic conditions (Millar, 2002; Sennett and Rendl, 2012). Only in rabbits and mice with large injuries HF neogenesis has been described in adult tissues (Breedis, 1954; Billingham and Russell, 1956; Ito et al., 2007).
Once the intact skin barrier is damaged, an interplay of complex cellular and molecular mechanisms is set in place to quickly and efficiently reinstate epidermal integrity in order to reestablish the skin’s impeccable and vital barrier function. To that end, a myriad of different cell types including thrombocytes, cells of the innate and adaptive immunity, epidermal stem cells, keratinocytes, endothelial cells, neurons and, most importantly, dermal fibroblasts synergistically partake in this intricate phenomenon of wound healing (Gonzalez et al., 2016; Correa-Gallegos and Rinkevich, 2022). Most importantly, mesenchymal responses are indispensable for tissue regeneration (Grose and Werner, 2003; Jiang and Rinkevich, 2020).
Mouse skin dermis comprises at least two functionally distinct fibroblast lineages which develop from a common progenitor: the papillary fibroblasts which contribute to the upper dermis including hair-follicle-associated fibroblasts of the dermal sheath (DS) and dermal papilla (DP) and are essential for HF development, and the reticular fibroblasts giving rise to adipocytes and preadipocytes of the hypodermis (Driskell et al., 2013). In vivo lineage tracing revealed that fibroblast diversification toward functional lineages occurs before embryonic day 16.5 (E16.5) (Driskell et al., 2013), possibly as early as E12.5 (Jacob et al., 2023). Additional heterogeneity within these two fibroblast lineages was demonstrated by scRNA-Seq of adult mouse skin (Joost et al., 2020). Recent spatial and single cell transcriptomic data confirm that also human skin harbors two major fibroblast subsets (Philippeos et al., 2018; Tabib et al., 2018; Korosec et al., 2019; Vorstandlechner et al., 2020). While differences in fibroblast subsets and markers exist between mouse and human skin, the two main subsets are functionally similar, e.g. reticular fibroblasts can undergo adipogenic differentiation, while papillary cannot (Driskell et al., 2013; Korosec et al., 2019). Reticular fibroblasts represent the majority of fibroblasts in adult tissue (Driskell et al., 2013; Lichtenberger et al., 2016) and have been shown to play a predominant role in fibrosis and cutaneous wound healing (Driskell et al., 2013; Rinkevich et al., 2015; Mastrogiannaki et al., 2016; Jiang and Rinkevich, 2020).
In mammalian postnatal skin, tissue damage that exceeds the epidermis and reaches into the papillary dermis, the upper layer of the skin dermis, or beyond, usually results in healing by scarring, which entails the reestablishment of the skin’s basic barrier to external threats and water loss while failing to restore its original architecture (Correa-Gallegos and Rinkevich, 2022). Scarred skin lacks secondary appendages such as HFs and sebaceous glands but also tensile strength and the skin’s original resilience to injury, as a dense plug of often stiff scar tissue is produced to seal the wound immediately (Erickson and Echeverri, 2018). During wound closure, resident dermal fibroblasts strongly proliferate and invade the wound edges. Using adhesive wound bed fibronectin as a scaffold for migration, these fibroblasts manage to perambulate the coagulation tissue to reach the wound bed (Yates et al., 2012), where they produce collagens and other extracellular matrix (ECM) proteins such as hyaluronic acid, fibronectin and tenascin C, thus, ultimately forming the bulk of connective tissue that constitutes the granulation tissue of the emerging scar (Sorg et al., 2017). Importantly, Driskell et al. demonstrated that this initial phase of wound repair and ECM deposition is primarily mediated by Dlk1+ fibroblasts of reticular origin, while Blimp1+ fibroblasts of papillary origin repopulate the wound bed only when a neo-epidermis has already formed (Driskell et al., 2013). In line, Rinkevich et al. showed that fibroblasts with transient Engrailed-1 expression during embryogenesis (En1 lineage positive fibroblasts, EPFs), represent the matrix-producing fibroblast lineage that mediates the deposition of granulation tissue and that likely corresponds to the reticular lineage (Rinkevich et al., 2015). Recent scRNA-Seq analyses delineated multiple unique wound fibroblast populations in regenerating murine wounds, including a rare fibroblast subpopulation that derives from myeloid progenitor cells and that gives rise to specific regenerated wound adipocytes (Guerrero-Juarez et al., 2019). The release of TGFβ by immune cells induces a transition from reticular fibroblasts into activated fibroblasts, so called “contractile myofibroblasts” (Bochaton-Piallat et al., 2016). These activated fibroblasts together with their ECM are responsible for wound contraction and new collagen and ECM deposition, which will subsequently form the scar. Apart from TGFβ signaling, the Wnt/β-catenin pathway has been shown to be a key player in dermal wound repair. While Wnt/β-catenin activity plays a critical role in epidermal stem cell maintenance, HF morphogenesis and regeneration (Andl et al., 2002; Fuchs and Raghavan, 2002; Clevers, 2006; Klaus and Birchmeier, 2008; Holland et al., 2013; Lien and Fuchs, 2014; Gonzales and Fuchs, 2017), it is also well known to have opposite effects in fibroblasts, where Wnt-signaling mediates skin fibrosis and pathological scarring (Sato, 2006; Akhmetshina et al., 2012; Hamburg-Shields et al., 2015; Lichtenberger et al., 2016; Mastrogiannaki et al., 2016).
The wound-induced hair follicle neogenesis (WIHN) model provides an outstanding platform for exploring mammalian skin regeneration. This concept was first introduced in 1956, when Billingham et al. observed HF neogenesis at the center of wounds in rabbits that were too large to close entirely by contraction (Billingham and Russell, 1956). WIHN closely recapitulates the molecular and morphological events of embryonic HF development, resulting in functional HFs comprising all cell types present in normal HFs. Of note, WIHN is much more frequent in neonatal wounds than in injuries of adult skin (Rognoni et al., 2016), see Figure 1. Like HF neogenesis during skin development, also WIHN depends on tightly regulated reciprocal signaling between epidermal cells and fibroblasts of the underlying dermis.
FIGURE 1. Wound-induced hair follicle de novo formation is more frequent in neonatal than in adult wound beds. Immunofluorescence-stainings of reepithelialized wound beds from (A) adult wounds 14 days after injury, (B) neonatal wounds 7 days after injury and (C) magnification of de novo hair follicles (HFs) in neonatal wound beds. (A) and (B) Yellow dotted lines indicate wound margins, white dotted lines demarcate the border between epidermis and dermis, and white arrows indicate de novo HFs. (C) Red dotted lines demarcate epithelial and mesenchymal compartments of de novo HFs and red arrows indicate de novo dermal papillae. Scale bars represent 500 µm.
Importantly, certain non-mammalian species such as amphibians or flatworms manage to regenerate entire limbs or even their full body, respectively, after injury (Reddien, 2018; Dwaraka and Voss, 2021). Scarless healing in mammals, however, is a much more infrequent phenomenon and only fetal and early neonatal wounds exhibit an inherent capacity to heal by complete regeneration (Leavitt et al., 2016). The Wnt/β-catenin signaling pathway, as one of the main embryonically activated pathways during skin morphogenesis, has become one of the main protagonists for the reconstitution of a fetal- or neonatal-like regenerative skin state. For instance, Collins et al. demonstrated that a neonatal-like state of the skin dermis with prominent fibroblasts proliferation, a production of a neonatal-like extracellular matrix and the formation of ectopic HFs can be induced by epidermal activation of β-catenin (Collins et al., 2011). Importantly, this de novo HF formation from sebaceous gland and interfollicular epithelial cells upon transient epidermal β-catenin activation depends on the Hedgehog (Hh)-signaling pathway in unwounded skin (Silva-Vargas et al., 2005).
Correspondingly, epidermal Wnt-signaling has prominently been implicated in WIHN as well. First, Ito et al. demonstrated that epidermal overexpression of Wnt7a increased WIHN at the centre of large murine adult wounds (Ito et al., 2007). Several more recent reports support these data. For instance, Wang et al. demonstrated that tumor-necrosis factor alpha (TNFα) produced by wound-infiltrating macrophages promotes the activation of β-catenin via AKT and subsequently entails the expansion of Lgr5+ HF stem cells and the de novo formation of HFs in healing wound beds (Wang et al., 2017). Furthermore, inhibition of CXXC5, which is overexpressed in HFs of human balding skin and acts as a prominent antagonist to Wnt-signaling, led to hair regrowth and increased WIHN (Lee et al., 2017).
The modulation of other embryonic signaling pathways such as the Hh-signaling pathway has become of equal interest when it comes to the restoration of a neonatal-like skin state. Importantly, Sun et al. demonstrated that Hh-activation in naturally hairless paw skin led to the formation of neogenic HFs, however only upon concomitant activation in both, the epidermis and the dermis. Epidermal or dermal activation alone produced HF-associated tumors or the formation of a stromal condensate without subsequent HF formation, respectively (Sun et al., 2020). A recent report has established the muscle segment homeobox or Msx gene family as an important contributor to WIHN, as epidermal deletion of Msx inhibited HF de novo formation in large adult wounds. Importantly, in contrast to its prominent role in embryonic HF morphogenesis, modulation of bone-morphogenic protein (BMP)-signaling did not affect WIHN, which suggests that wound bed regeneration is independent of BMP-signaling (Hughes et al., 2018).
As previously described, inflammatory cells in the wound bed such as macrophages (Gay et al., 2020) and released factors like CXXC5 (Lee et al., 2017) strongly interfere in wound bed regeneration. In line, IL1β derived from wound-bed colonizing bacteria such as Staphylococcus aureus was shown to promote WIHN (Wang et al., 2021). Furthermore, dsRNA that is released into the wound microenvironment from damaged cells binds to TLR3, which entails subsequent activation of canonical embryonic signaling pathways such as the EDAR-, Hh- and Wnt-signaling pathways and increased WIHN (Nelson et al., 2015). Non-coding dsRNA moreover positively influences wound bed regeneration and de novo HF formation by upregulating retinoic-acid synthesis via TL3-activation (Kim et al., 2019) as well as through promotion of Wnt7b expression mediated by upregulation of wound bed prostaglandins (Zhu et al., 2017). Moreover, IL36α has been implicated in WIHN as its expression spikes surrounding neogenic HFs and treatment with recombinant IL36α promoted WIHN via the IL6/Stat3 pathway (Gong et al., 2020).
Various studies have addressed the role of Wnt-signaling in fibroblasts in the context of wound regeneration. Intriguingly, Rognoni et al. showed that the postnatal loss of hair forming ability correlated with a significant upregulation of Wnt-signaling in healing dermis (Rognoni et al., 2016). Inhibition of β-catenin-signaling in dermal fibroblasts of adult mice led to increased HF de novo formation in the wound, whereas β-catenin activation reduced HF regeneration in neonatal wounds (Rognoni et al., 2016; Lim et al., 2018), suggesting that highly activated dermal Wnt-signaling during wound closure might be responsible for scarce WIHN in adult skin. Correspondingly, macrophage-mediated phagocytosis of the Wnt-inhibitor SFRP4 promoted a non-regeneratory fibrotic wound phenotype while abrogation of SFRP4-phagocytosis promoted regeneration and WIHN by reducing dermal Wnt-signaling (Gay et al., 2020). In contrast to these reports, Phan et al. observed enhanced skin repair and increased WIHN upon Lef1 overexpression and Wnt-activation in Twist2-expressing dermal fibroblasts (Phan et al., 2020). Along the same line, Gay et al. reported that γδ T cell-derived FGF9 triggered Lef1 expression in dermal fibroblasts, and enhanced WIHN (Gay et al., 2013). Mascharak et al. reported that disrupting YAP-dependent mechanotransduction induces regenerative repair by fibroblasts with activated Trps1 and Wnt signaling (Mascharak et al., 2022). These contradicting results might be explained by different Lef/Tcf co-transcription factors that direct a context-dependent Wnt/β-catenin-specific response. Thus, the role of dermal Wnt-signaling in WIHN and regeneration during wounding needs to be explored in more detail.
Another important embryonic signaling pathway is the Hh pathway. Intriguingly, modulation of epidermal or dermal Hh-signaling polarized dermal cells towards a dermal papilla fate and thereby promoted a regenerative wound phenotype with prominent WIHN (Lim et al., 2018). Frech et al. were able to observe a dual role for dermal Hh-signaling in distinct dermal fibroblasts lineages during wound healing. In fact, they demonstrated that Hh-signaling in the reticular fibroblast lineage promotes wound closure, possibly via increased angiogenesis and the expansion of PDGFRα+ wound bed fibroblasts, while Hh-signaling in the papillary fibroblast lineage is essential for the expression of HF morphogenesis-associated genes and de novo HF formation within the healing wound (Frech et al., 2022). Accordingly, scRNA-Seq revealed that direct effectors of Hh-signaling, such as Gli1, are solely active in fibroblasts within the central area of large wounds, where HF neogenesis occurs (Abbasi et al., 2020). This study also suggested that retinoic acid (RA) and Runx1 are master regulators of WIHN, and demonstrated that fibroblast-specific deletion of the quiescence-associated factor hypermethylated in cancer 1 (Hic1) enhanced WIHN by increasing fibroblast density in the early repair phase of large wounds. Altogether, these data indicate that Hh-signaling contributes to the mesenchymal competence for de novo HF regeneration within interfollicular epidermis, and that many other factors with regeneration-enhancing capability remain to be identified for future therapeutic approaches.
The papillary fibroblast lineage not only gives rise to all HF-associated mesenchymal cells such as the dermal papilla and the dermal sheath, it is also essential for HF development during embryogenesis (Driskell et al., 2013; Gupta et al., 2019; Mok et al., 2019). Papillary and reticular fibroblasts are not only functionally distinct, they also respond to different epidermis-derived signals in a unique manner (Lichtenberger et al., 2016). As WIHN recapitulates the major steps of embryonic HF morphogenesis, it is therefore not surprising that the papillary fibroblast lineage has proven crucial in this context as well. While it has long been postulated that HFs might be a source of mesenchymal cells with regenerative potential during wound healing (Jahoda and Reynolds, 2001), and a subset of HF-associated fibroblasts continuously replenishes HFs with fibroblasts as they undergo repeated cycles of degeneration, remodeling, and regeneration (Rahmani et al., 2014), these HF-associated fibroblasts migrate into the wounds but only contribute a minority of cells to newly formed HFs and the granulation tissue (Abbasi et al., 2020). Instead, fibroblasts of the upper interfollicular dermis (fate-mapped with the quiescence-associated factor hypermethylated in cancer 1, Hic1) contribute largely to both the mesenchymal compartment of neogenic HFs and the wound neo-dermis (Abbasi et al., 2020). In their study, Rognoni et al. demonstrated that impaired hair forming ability correlated with the age-dependent reduction in the abundance of papillary fibroblasts (Rognoni et al., 2016). Accordingly, single-cell transcriptomics revealed that WIHN depends on upper dermal fibroblasts that are closely related to cells of the DP at transcriptomic level, and that migrate from the wound edge towards the wound centre, where WIHN typically occurs (Phan et al., 2021), thus indicating that increasing papillary fibroblast density within the wound might promote WIHN (see Figure 2C). In fact, increasing the number of papillary fibroblasts in adult skin to neonatal levels prior to wounding via transient Wnt/β-catenin activation in the epidermis, restored the skin’s ability to form new HFs within the wound (Driskell et al., 2013). Rinkevich et al. discovered that during embryogenesis En1 lineage negative fibroblasts (ENFs), which are the dominant population in the developing dermis, minimally contribute to scar formation in adult wounds (Rinkevich et al., 2015). Transplantation of purified ENFs into donor wounds, led to a phenotypic transition of wounds from scarring to regeneration (Jiang et al., 2018). Importantly, Hh-signaling in the papillary fibroblast lineage but not the reticular fibroblast lineage is crucial for HF regeneration during wound healing (Frech et al., 2022). While it has been suggested that reprogramming scarring fibroblasts during wound healing might contribute to a more regenerative wound phenotype that entails WIHN (Mascharak et al., 2022), the majority of data points towards the expansion of fibroblasts of papillary origin itself, either via activation of embryonic signaling pathways and subsequent proliferation (Lichtenberger et al., 2016; Rognoni et al., 2016; Phan et al., 2020) or via transplantation (Rinkevich et al., 2015), or by inhibiting reticular fibroblasts, as the key event that mediates the switch from scarring to regenerative healing within the wound bed.
FIGURE 2. Reinstating a neonatal-like state during wounding promotes regeneration. (A) The reticular fibroblast lineage mediates the initial phase of wound repair including the deposition of collagens and other components of the ECM, while (B) the papillary fibroblast lineage repopulates the wound bed only at later stages of wound repair. Manipulation of the dermal cellular composition like (C) expanding the papillary fibroblast lineage or (D) modulation of embryonic signaling pathways such as the Wnt- and Hh-signaling pathways in fibroblasts increases WIHN and regeneration during wounding.
Regeneration is the best possible outcome of tissue repair, but skin injury typically leads to fibrotic, non-functional scars. Our inability to regenerate fully functional skin and the appendages contained within the dermis is a major impediment to human skin wound healing. Apart from imperfectly healed wounds in adult skin, the aging-related decline in the skin’s capability to heal completely and skin fragility are a major risk of morbidity in the elderly. Similar to mouse skin, also in human skin the number of fibroblasts, specifically that of papillary fibroblasts, declines as we age, concomitant with accumulating senescent fibroblasts, resulting in skin thinning (Harper and Grove, 1979; Gilchrest, 1982; Gilchrest, 1996; Varani et al., 2001) and a progressive loss of the adipocyte layer (Kruglikov and Scherer, 2016). Importantly, aging not only affects the expression of a plethora of genes in fibroblasts (Haydont et al., 2019a; Haydont et al., 2019b; Sole-Boldo et al., 2020) but also substantially reduces the interactions of dermal fibroblasts with other skin cell types (Sole-Boldo et al., 2020), which likely further affects skin function. Since WIHN is age-dependent, reactivation of embryonic signals in aged fibroblasts might also counteract impaired wound healing. Alopecia and chronic wounds are also debilitating complications of diabetes mellitus (Liu et al., 2022). Intriguingly, like hair growth and cycling, also WIHN was affected in diabetic mice but could be restored by a small molecule activating Wnt/β-catenin signaling (Ryu et al., 2022). Interestingly, recent findings suggest that high glucose levels also affect the immunomodulatory functions of fibroblasts (Al-Rikabi et al., 2021; Liu et al., 2022). The development of pro-regenerative therapies requires detailed understanding of the cellular and molecular events that determine if an injury heals with fibrosis and scarring, or regenerates scarlessly towards a fully functional skin comprising its appendages. Since the overall architecture of murine and human skin are similar and the major fibroblast subsets in both tissues are alike, the WIHN model is an excellent tool to study how tissues mobilize and coordinate distinct cell populations to initiate embryonic-like skin regeneration. This model has already provided important insights into the cellular and molecular mechanisms of wound healing, and thus for the development of future regenerative medicine approaches. Both, increasing papillary fibroblast density and modulating dermal signaling seem to be promising approaches to shift wound repair with scarring towards complete regeneration. Reactivating high-fidelity tissue morphogenesis signaling pathways in adults following a severe injury of the skin could replace or reduce the need for transplantation therapies.
SF: Conceptualization, Visualization, Writing–original draft, Writing–review and editing. BL: Conceptualization, Funding acquisition, Writing–original draft, Writing–review and editing.
The author(s) declare financial support was received for the research, authorship, and/or publication of this article. This project was funded in part, by the Austrian Science Fund (FWF) [P 35307-B] and the LEO Foundation [LF-AW_EMEA-21-400116].
We apologize to colleagues whose contributions could not be cited due to space constraints. We would like to thank Ana Korosec for graphical design of Figure 2. This project was funded in part, by the Austrian Science Fund (FWF) [P 35307-B] and the LEO Foundation [LF-AW_EMEA-21-400116]. For the purpose of open access, the author has applied a CC BY public copyright license to any Author Accepted Manuscript version arising from this submission.
The authors declare that the research was conducted in the absence of any commercial or financial relationships that could be construed as a potential conflict of interest.
All claims expressed in this article are solely those of the authors and do not necessarily represent those of their affiliated organizations, or those of the publisher, the editors and the reviewers. Any product that may be evaluated in this article, or claim that may be made by its manufacturer, is not guaranteed or endorsed by the publisher.
Abbasi S., Sinha S., Labit E., Rosin N. L., Yoon G., Rahmani W., et al. (2020). Distinct regulatory programs control the latent regenerative potential of dermal fibroblasts during wound healing. Cell Stem Cell 27, 396–412. doi:10.1016/j.stem.2020.07.008
Akhmetshina A., Palumbo K., Dees C., Bergmann C., Venalis P., Zerr P., et al. (2012). Activation of canonical Wnt signalling is required for TGF-β-mediated fibrosis. Nat. Commun. 3, 735. doi:10.1038/ncomms1734
Al-Rikabi A. H. A., Tobin D. J., Riches-Suman K., Thornton M. J. (2021). Dermal fibroblasts cultured from donors with type 2 diabetes mellitus retain an epigenetic memory associated with poor wound healing responses. Sci. Rep. 11, 1474. doi:10.1038/s41598-020-80072-z
Andl T., Reddy S. T., Gaddapara T., Millar S. E. (2002). WNT signals are required for the initiation of hair follicle development. Dev. Cell 2, 643–653. doi:10.1016/s1534-5807(02)00167-3
Billingham R. E., Russell P. S. (1956). Incomplete wound contracture and the phenomenon of hair neogenesis in rabbits' skin. Nature 177, 791–792. doi:10.1038/177791b0
Bochaton-Piallat M. L., Gabbiani G., Hinz B. (2016). The myofibroblast in wound healing and fibrosis: answered and unanswered questions. F1000Res 5. doi:10.12688/f1000research.8190.1
Breedis C. (1954). Regeneration of hair follicles and sebaceous glands from the epithelium of scars in the rabbit. Cancer Res. 14, 575–579.
Clevers H. (2006). Wnt/beta-catenin signaling in development and disease. Cell 127, 469–480. doi:10.1016/j.cell.2006.10.018
Collins C. A., Kretzschmar K., Watt F. M. (2011). Reprogramming adult dermis to a neonatal state through epidermal activation of β-catenin. Development 138, 5189–5199. doi:10.1242/dev.064592
Correa-Gallegos D., Rinkevich Y. (2022). Cutting into wound repair. FEBS J. 289, 5034–5048. doi:10.1111/febs.16078
Driskell R. R., Lichtenberger B. M., Hoste E., Kretzschmar K., Simons B. D., Charalambous M., et al. (2013). Distinct fibroblast lineages determine dermal architecture in skin development and repair. Nature 504, 277–281. doi:10.1038/nature12783
Dwaraka V. B., Voss S. R. (2021). Towards comparative analyses of salamander limb regeneration. J. Exp. Zool. B Mol. Dev. Evol. 336, 129–144. doi:10.1002/jez.b.22902
Erickson J. R., Echeverri K. (2018). Learning from regeneration research organisms: the circuitous road to scar free wound healing. Dev. Biol. 433, 144–154. doi:10.1016/j.ydbio.2017.09.025
Frech S., Forsthuber A., Korosec A., Lipp K., Kozumov V., Lichtenberger B. M. (2022). Hedgehog signaling in papillary fibroblasts is essential for hair follicle regeneration during wound healing. J. Invest. Dermatol 142, 1737–1748.e5. doi:10.1016/j.jid.2021.11.026
Fuchs E. (2007). Scratching the surface of skin development. Nature 445, 834–842. doi:10.1038/nature05659
Fuchs E., Raghavan S. (2002). Getting under the skin of epidermal morphogenesis. Nat. Rev. Genet. 3, 199–209. doi:10.1038/nrg758
Gay D., Ghinatti G., Guerrero-Juarez C. F., Ferrer R. A., Ferri F., Lim C. H., et al. (2020). Phagocytosis of Wnt inhibitor SFRP4 by late wound macrophages drives chronic Wnt activity for fibrotic skin healing. Sci. Adv. 6, doi:10.1126/sciadv.aay3704
Gay D., Kwon O., Zhang Z., Spata M., Plikus M. V., Holler P. D., et al. (2013). Fgf9 from dermal γδ T cells induces hair follicle neogenesis after wounding. Nat. Med. 19, 916–923. doi:10.1038/nm.3181
Gilchrest B. A. (1982). Age-associated changes in the skin. J. Am. Geriatr. Soc. 30, 139–143. doi:10.1111/j.1532-5415.1982.tb01289.x
Gilchrest B. A. (1996). A review of skin ageing and its medical therapy. Br. J. Dermatology 135, 867–875. doi:10.1046/j.1365-2133.1996.d01-1088.x
Gong L., Xiao J., Li X., Li Y., Gao X., Xu X. (2020). IL-36α promoted wound induced hair follicle neogenesis via hair follicle stem/progenitor cell proliferation. Front. Cell Dev. Biol. 8, 627. doi:10.3389/fcell.2020.00627
Gonzales K. A. U., Fuchs E. (2017). Skin and its regenerative powers: an alliance between stem cells and their niche. Dev. Cell 43, 387–401. doi:10.1016/j.devcel.2017.10.001
Gonzalez A. C., Costa T. F., Andrade Z. A., Medrado A. R. (2016). Wound healing - a literature review. Bras Dermatol 91, 614–620. doi:10.1590/abd1806-4841.20164741
Grose R., Werner S. (2003). Wound healing studies in transgenic and knockout mice. A review. Methods Mol. Med. 78, 191–216. doi:10.1385/1-59259-332-1:191
Guerrero-Juarez C. F., Dedhia P. H., Jin S., Ruiz-Vega R., Ma D., Liu Y., et al. (2019). Single-cell analysis reveals fibroblast heterogeneity and myeloid-derived adipocyte progenitors in murine skin wounds. Nat. Commun. 10, 650. doi:10.1038/s41467-018-08247-x
Gupta K., Levinsohn J., Linderman G., Chen D., Sun T. Y., Dong D., et al. (2019). Single-cell analysis reveals a hair follicle dermal niche molecular differentiation trajectory that begins prior to morphogenesis. Dev. Cell 48, 17–31. doi:10.1016/j.devcel.2018.11.032
Hamburg-Shields E., DiNuoscio G. J., Mullin N. K., Lafyatis R., Atit R. P. (2015). Sustained β-catenin activity in dermal fibroblasts promotes fibrosis by up-regulating expression of extracellular matrix protein-coding genes. J. Pathol. 235, 686–697. doi:10.1002/path.4481
Harper R. A., Grove G. (1979). Human skin fibroblasts derived from papillary and reticular dermis: differences in growth potential in vitro. Science 204, 526–527. doi:10.1126/science.432659
Haydont V., Neiveyans V., Fortunel N. O., Asselineau D. (2019a). Transcriptome profiling of human papillary and reticular fibroblasts from adult interfollicular dermis pinpoints the 'tissue skeleton' gene network as a component of skin chrono-ageing. Mech. Ageing Dev. 179, 60–77. doi:10.1016/j.mad.2019.01.003
Haydont V., Neiveyans V., Zucchi H., Fortunel N. O., Asselineau D. (2019b). Genome-wide profiling of adult human papillary and reticular fibroblasts identifies ACAN, Col XI α1, and PSG1 as general biomarkers of dermis ageing, and KANK4 as an exemplary effector of papillary fibroblast ageing, related to contractility. Mech. Ageing Dev. 177, 157–181. doi:10.1016/j.mad.2018.06.003
Holland J. D., Klaus A., Garratt A. N., Birchmeier W. (2013). Wnt signaling in stem and cancer stem cells. Curr. Opin. Cell Biol. 25, 254–264. doi:10.1016/j.ceb.2013.01.004
Hughes M. W., Jiang T. X., Plikus M. V., Guerrero-Juarez C. F., Lin C. H., Schafer C., et al. (2018). Msx2 supports epidermal competency during wound-induced hair follicle neogenesis. J. Invest. Dermatol 138, 2041–2050. doi:10.1016/j.jid.2018.02.043
Ito M., Yang Z., Andl T., Cui C., Kim N., Millar S. E., et al. (2007). Wnt-dependent de novo hair follicle regeneration in adult mouse skin after wounding. Nature 447, 316–320. doi:10.1038/nature05766
Jacob T., Annusver K., Czarnewski P., Dalessandri T., Kalk C., Levra Levron C., et al. (2023). Molecular and spatial landmarks of early mouse skin development. Dev. Cell 58, 2140–2162.e5. doi:10.1016/j.devcel.2023.07.015
Jahoda C. A., Reynolds A. J. (2001). Hair follicle dermal sheath cells: unsung participants in wound healing. Lancet 358, 1445–1448. doi:10.1016/S0140-6736(01)06532-1
Jiang D., Correa-Gallegos D., Christ S., Stefanska A., Liu J., Ramesh P., et al. (2018). Two succeeding fibroblastic lineages drive dermal development and the transition from regeneration to scarring. Nat. Cell Biol. 20, 422–431. doi:10.1038/s41556-018-0073-8
Jiang D., Rinkevich Y. (2020). Scars or regeneration? dermal fibroblasts as drivers of diverse skin wound responses. Int. J. Mol. Sci. 21, 617. doi:10.3390/ijms21020617
Joost S., Annusver K., Jacob T., Sun X., Dalessandri T., Sivan U., et al. (2020). The molecular anatomy of mouse skin during hair growth and rest. Cell Stem Cell 26, 441–457. doi:10.1016/j.stem.2020.01.012
Kim D., Chen R., Sheu M., Kim N., Kim S., Islam N., et al. (2019). Noncoding dsRNA induces retinoic acid synthesis to stimulate hair follicle regeneration via TLR3. Nat. Commun. 10, 2811. doi:10.1038/s41467-019-10811-y
Klaus A., Birchmeier W. (2008). Wnt signalling and its impact on development and cancer. Nat. Rev. Cancer 8, 387–398. doi:10.1038/nrc2389
Korosec A., Frech S., Gesslbauer B., Vierhapper M., Radtke C., Petzelbauer P., et al. (2019). Lineage identity and location within the dermis determine the function of papillary and reticular fibroblasts in human skin. J. Invest. Dermatol 139, 342–351. doi:10.1016/j.jid.2018.07.033
Kruglikov I. L., Scherer P. E. (2016). Skin aging: are adipocytes the next target? Aging (Albany NY) 8, 1457–1469. doi:10.18632/aging.100999
Leavitt T., Hu M. S., Marshall C. D., Barnes L. A., Lorenz H. P., Longaker M. T. (2016). Scarless wound healing: finding the right cells and signals. Cell tissue Res. 365, 483–493. doi:10.1007/s00441-016-2424-8
Lee S. H., Seo S. H., Lee D. H., Pi L. Q., Lee W. S., Choi K. Y. (2017). Targeting of CXXC5 by a competing peptide stimulates hair regrowth and wound-induced hair neogenesis. J. Invest. Dermatol 137, 2260–2269. doi:10.1016/j.jid.2017.04.038
Lichtenberger B. M., Mastrogiannaki M., Watt F. M. (2016). Epidermal β-catenin activation remodels the dermis via paracrine signalling to distinct fibroblast lineages. Nat. Commun. 7, 10537. doi:10.1038/ncomms10537
Lien W. H., Fuchs E. (2014). Wnt some lose some: transcriptional governance of stem cells by Wnt/β-catenin signaling. Genes Dev. 28, 1517–1532. doi:10.1101/gad.244772.114
Lim C. H., Sun Q., Ratti K., Lee S. H., Zheng Y., Takeo M., et al. (2018). Hedgehog stimulates hair follicle neogenesis by creating inductive dermis during murine skin wound healing. Nat. Commun. 9, 4903. doi:10.1038/s41467-018-07142-9
Liu Y., Liu Y., He W., Mu X., Wu X., Deng J., et al. (2022). Fibroblasts: immunomodulatory factors in refractory diabetic wound healing. Front. Immunol. 13, 918223. doi:10.3389/fimmu.2022.918223
Mascharak S., Talbott H. E., Januszyk M., Griffin M., Chen K., Davitt M. F., et al. (2022). Multi-omic analysis reveals divergent molecular events in scarring and regenerative wound healing. Cell Stem Cell 29, 315–327.e6. doi:10.1016/j.stem.2021.12.011
Mastrogiannaki M., Lichtenberger B. M., Reimer A., Collins C. A., Driskell R. R., Watt F. M. (2016). β-Catenin stabilization in skin fibroblasts causes fibrotic lesions by preventing adipocyte differentiation of the Reticular Dermis. J. Invest. Dermatol 136, 1130–1142. doi:10.1016/j.jid.2016.01.036
Millar S. E. (2002). Molecular mechanisms regulating hair follicle development. J. Invest. Dermatol 118, 216–225. doi:10.1046/j.0022-202x.2001.01670.x
Mok K. W., Saxena N., Heitman N., Grisanti L., Srivastava D., Muraro M. J., et al. (2019). Dermal condensate niche fate specification occurs prior to formation and is placode progenitor dependent. Dev. Cell 48, 32–48. doi:10.1016/j.devcel.2018.11.034
Nelson A. M., Reddy S. K., Ratliff T. S., Hossain M. Z., Katseff A. S., Zhu A. S., et al. (2015). dsRNA released by tissue damage activates TLR3 to drive skin regeneration. Cell Stem Cell 17, 139–151. doi:10.1016/j.stem.2015.07.008
Phan Q. M., Fine G. M., Salz L., Herrera G. G., Wildman B., Driskell I. M., et al. (2020). Lef1 expression in fibroblasts maintains developmental potential in adult skin to regenerate wounds. Elife 9, e60066. doi:10.7554/eLife.60066
Philippeos C., Telerman S. B., Oules B., Pisco A. O., Shaw T. J., Elgueta R., et al. (2018). Spatial and single-cell transcriptional profiling identifies functionally distinct human dermal fibroblast subpopulations. J. Invest. Dermatol 138, 811–825. doi:10.1016/j.jid.2018.01.016
Rahmani W., Abbasi S., Hagner A., Raharjo E., Kumar R., Hotta A., et al. (2014). Hair follicle dermal stem cells regenerate the dermal sheath, repopulate the dermal papilla, and modulate hair type. Dev. Cell 31, 543–558. doi:10.1016/j.devcel.2014.10.022
Reddien P. W. (2018). The cellular and molecular basis for planarian regeneration. Cell 175, 327–345. doi:10.1016/j.cell.2018.09.021
Rinkevich Y., Walmsley G. G., Hu M. S., Maan Z. N., Newman A. M., Drukker M., et al. (2015). Skin fibrosis. Identification and isolation of a dermal lineage with intrinsic fibrogenic potential. Science 348, aaa2151. doi:10.1126/science.aaa2151
Rognoni E., Gomez C., Pisco A. O., Rawlins E. L., Simons B. D., Watt F. M., et al. (2016). Inhibition of β-catenin signalling in dermal fibroblasts enhances hair follicle regeneration during wound healing. Development 143, 2522–2535. doi:10.1242/dev.131797
Ryu Y. C., Kim Y. R., Park J., Choi S., Kim G. U., Kim E., et al. (2022). Wnt/β-catenin signaling activator restores hair regeneration suppressed by diabetes mellitus. BMB Rep. 55, 559–564. doi:10.5483/BMBRep.2022.55.11.081
Sato M. (2006). Upregulation of the Wnt/beta-catenin pathway induced by transforming growth factor-beta in hypertrophic scars and keloids. Acta Derm. Venereol. 86, 300–307. doi:10.2340/00015555-0101
Sennett R., Rendl M. (2012). Mesenchymal-epithelial interactions during hair follicle morphogenesis and cycling. Seminars Cell & Dev. Biol. 23, 917–927. doi:10.1016/j.semcdb.2012.08.011
Silva-Vargas V., Lo Celso C., Giangreco A., Ofstad T., Prowse D. M., Braun K. M., et al. (2005). Beta-catenin and Hedgehog signal strength can specify number and location of hair follicles in adult epidermis without recruitment of bulge stem cells. Dev. Cell 9, 121–131. doi:10.1016/j.devcel.2005.04.013
Sole-Boldo L., Raddatz G., Schutz S., Mallm J. P., Rippe K., Lonsdorf A. S., et al. (2020). Single-cell transcriptomes of the human skin reveal age-related loss of fibroblast priming. Commun. Biol. 3, 188. doi:10.1038/s42003-020-0922-4
Sorg H., Tilkorn D. J., Hager S., Hauser J., Mirastschijski U. (2017). Skin wound healing: an update on the current knowledge and concepts. Eur. Surg. Res. 58, 81–94. doi:10.1159/000454919
Sun X., Are A., Annusver K., Sivan U., Jacob T., Dalessandri T., et al. (2020). Coordinated hedgehog signaling induces new hair follicles in adult skin. Elife 9, e46756. doi:10.7554/eLife.46756
Tabib T., Morse C., Wang T., Chen W., Lafyatis R. (2018). SFRP2/DPP4 and FMO1/LSP1 define major fibroblast populations in human skin. J. Invest. Dermatol 138, 802–810. doi:10.1016/j.jid.2017.09.045
Varani J., Spearman D., Perone P., Fligiel S. E., Datta S. C., Wang Z. Q., et al. (2001). Inhibition of type I procollagen synthesis by damaged collagen in photoaged skin and by collagenase-degraded collagen in vitro. Am. J. Pathol. 158, 931–942. doi:10.1016/S0002-9440(10)64040-0
Vorstandlechner V., Laggner M., Kalinina P., Haslik W., Radtke C., Shaw L., et al. (2020). Deciphering the functional heterogeneity of skin fibroblasts using single-cell RNA sequencing. FASEB J. 34, 3677–3692. doi:10.1096/fj.201902001RR
Wang G., Sweren E., Liu H., Wier E., Alphonse M. P., Chen R., et al. (2021). Bacteria induce skin regeneration via IL-1β signaling. Cell Host Microbe 29, 777–791.e6. doi:10.1016/j.chom.2021.03.003
Wang X., Chen H., Tian R., Zhang Y., Drutskaya M. S., Wang C., et al. (2017). Macrophages induce AKT/β-catenin-dependent Lgr5+ stem cell activation and hair follicle regeneration through TNF. Nat. Commun. 8, 14091. doi:10.1038/ncomms14091
Watt F. M., Fujiwara H. (2011). Cell-extracellular matrix interactions in normal and diseased skin. Cold Spring Harb. Perspect. Biol. 3, a005124. doi:10.1101/cshperspect.a005124
Yates C. C., Hebda P., Wells A. (2012). Skin wound healing and scarring: fetal wounds and regenerative restitution. Birth Defects Res. C Embryo Today 96, 325–333. doi:10.1002/bdrc.21024
Keywords: skin regeneration, fibroblast, hair follicle, WIHN, embryonic signaling pathways
Citation: Frech S and Lichtenberger BM (2024) Modulating embryonic signaling pathways paves the way for regeneration in wound healing. Front. Physiol. 15:1367425. doi: 10.3389/fphys.2024.1367425
Received: 08 January 2024; Accepted: 05 February 2024;
Published: 16 February 2024.
Edited by:
John Connelly, Queen Mary University of London, United KingdomReviewed by:
Brett Shook, George Washington University, United StatesCopyright © 2024 Frech and Lichtenberger. This is an open-access article distributed under the terms of the Creative Commons Attribution License (CC BY). The use, distribution or reproduction in other forums is permitted, provided the original author(s) and the copyright owner(s) are credited and that the original publication in this journal is cited, in accordance with accepted academic practice. No use, distribution or reproduction is permitted which does not comply with these terms.
*Correspondence: Beate M. Lichtenberger, YmVhdGUubGljaHRlbmJlcmdlckBtZWR1bml3aWVuLmFjLmF0
Disclaimer: All claims expressed in this article are solely those of the authors and do not necessarily represent those of their affiliated organizations, or those of the publisher, the editors and the reviewers. Any product that may be evaluated in this article or claim that may be made by its manufacturer is not guaranteed or endorsed by the publisher.
Research integrity at Frontiers
Learn more about the work of our research integrity team to safeguard the quality of each article we publish.