- 1Graduate School, Wuhan Sports University, Wuhan, China
- 2Department of Physical Education, Suzhou Vocational University, Suzhou, China
- 3Department of Basic Teaching, Suzhou City University, Suzhou, China
Objectives: This review aims to summarize the common physiological mechanisms associated with both mild cognitive impairment (MCI) and musculoskeletal aging while also examining the relevant literature on how exercise regulation influences the levels of shared myokines in these conditions.
Methods: The literature search was conducted via databases such as PubMed (including MEDLINE), EMBASE, and the Cochrane Library of Systematic Reviews. The searches were limited to full-text articles published in English, with the most recent search conducted on 16 July 2024. The inclusion criteria for this review focused on the role of exercise and myokines in delaying musculoskeletal aging and enhancing cognitive health. The Newcastle‒Ottawa Scale (NOS) was utilized to assess the quality of nonrandomized studies, and only those studies with moderate to high quality scores, as per these criteria, were included in the final analysis. Data analysis was performed through narrative synthesis.
Results: The primary outcome of this study was the evaluation of myokine expression, which included IL-6, IGF-1, BDNF, CTSB, irisin, and LIF. A total of 16 studies involving 633 older adults met the inclusion criteria. The current exercise modalities utilized in these studies primarily consisted of resistance training and moderate-to high-intensity cardiovascular exercise. The types of interventions included treadmill training, elastic band training, aquatic training, and Nordic walking training. The results indicated that both cardiovascular exercise and resistance exercise could delay musculoskeletal aging and enhance the cognitive functions of the brain. Additionally, different types and intensities of exercise exhibited varying effects on myokine expression.
Conclusion: Current evidence suggests that exercise mediates the secretion of specific myokines, including IL-6, IGF-1, BDNF, CTSB, irisin, and LIF, which establish self-regulatory circuits between the brain and muscle. This interaction enhances cognitive function in the brain and improves skeletal muscle function. Future research should focus on elucidating the exact mechanisms that govern the release of myokines, the correlation between the intensity of exercise and the secretion of these myokines, and the distinct processes by which myokines influence the interaction between muscle and the brain.
1 Introduction
With a global increase in life expectancy, sarcopenia, cognitive impairment, frailty, and age-related anorexia, have become prominent issues in geriatric care (Morley, 2017b). A previous study reported that the global prevalence of dementia is expected to double every 20 years, potentially reaching 131.5 million patients by 2050 (Prince et al., 2015). A significant majority (60%–80%) of dementia cases comprise individuals with Alzheimer’s disease (AD), a neurodegenerative disorder. Mild cognitive impairment (MCI), on the other hand, serves as a transitional stage between mild dementia and normal aging, and often precedes the onset of AD (Grundman et al., 2004). Unlike the typical cognitive changes associated with aging, MCI often involves a decline in cognitive abilities, such as memory loss and learning difficulties, but does not meet the criteria for dementia. However, over 50% of individuals with MCI often progress to AD or other forms of dementia within 4–6 years (Hansson et al., 2006).
Sarcopenia and cognition, both of which are linked to aging, are also closely associated with each other and are crucial areas of ongoing research. Components of sarcopenia, such as gait speed and muscle strength, have been linked to cognitive impairment. Buchman et al. reported a 9% increase in the risk of AD for every 1-lb annual decrease in grip strength (Buchman et al., 2007). Additionally, gait speed is also a well-established predictor of dementia, especially in individuals with underlying cognitive impairment (Montero-Odasso et al., 2020). The EWGSOP2 criteria for muscle mass and gait speed correlate with a higher risk of mortality, indicating that a decrease in muscle mass or function is linked to an elevated risk of death (Cruz-Jentoft et al., 2019). Therefore, sarcopenia may lead to a strong bidirectional association between muscular dysfunction, diminished gait speed, and cognitive decline, which is often referred to as the muscle-brain loop.
Multiple studies have highlighted the importance of regular exercise in preventing and managing metabolic disorders, such as obesity, type 2 diabetes, and sarcopenia (Booth et al., 2012). Physical activity has also been linked to improved cognitive function and a reduced risk of neurodegenerative diseases (Tyndall et al., 2018). With chronic exposure to exercise, the body undergoes a series of adaptations to meet increased demands. This can involve changes in muscle strength, cardiovascular capacity, and metabolic efficiency, among other factors. The allostasis-interoception model posits that the brain plays a key role in predicting and meeting physiological needs resulting from regular exercise. By constantly monitoring internal conditions, the brain can adjust the body’s systems to maintain optimal function (Bobba-Alves et al., 2022). Regular physical activity plays a crucial role in maintaining the body’s ability to effectively anticipate and respond to stressors. Conversely, a sedentary lifestyle can disrupt this ability, leading to a decline in overall health and an increased risk of chronic conditions. Studies have indicated that sedentary behavior and a lack of physical activity are risk factors for sarcopenia in individuals with MCI and AD (Sugimoto et al., 2016), and consistent exercise or physical training can lead to various physiological changes that enhance skeletal muscle size and strength, ultimately aiding in counteracting the negative effects of sarcopenia in older adults. Despite these findings, a complete understanding of the molecular mechanisms involved is lacking.
A noteworthy contribution to this field is the concept of myokines (cytokines produced by muscles) by Pedersen and colleagues (Pedersen et al., 2003). Subsequently, Safdar and his team introduced the concept of exerkines, which include myokines as well as other compounds, such as metabolites, extracellular vesicles, and nucleic acids that are released during muscle contraction, all of which together form the myometabokiome (Safdar et al., 2016). The interactions among myokines, which are transported to various organs through the circulatory system, present a promising area of research as it may potentially offer insights into their access to the central nervous system (CNS). Therefore, in this research, we examined the molecular mechanisms underlying the role of exercise-regulated myokines in musculoskeletal aging and MCI, to provide new insights and identify therapeutic targets for the treatment of aging-associated skeletal muscle atrophy and MCI.
2 Methods
2.1 Search strategy and selection criteria
This review was conducted in accordance with the Preferred Reporting Items for Systematic Reviews and Meta-Analyses (PRISMA 2020) guidelines (Page et al., 2021). The literature search was carried out across various databases, such as PubMed (including MEDLINE), EMBASE, and the Cochrane Library of Systematic Reviews. The search was restricted to full-text articles published in English, with the most recent search conducted on 16 July 2024. The search terms used were “physical activity,” “physical exercise,” “exercise,” “sport,” “myokine,” “musculoskeletal aging,” “sarcopenia,” “aging-associated skeletal muscle atrophy,” “mild cognitive impairment,” “cognition,” and “Alzheimer’s disease”. Sixteen articles meeting the inclusion criteria were included after a thorough review process. The flowchart detailing the literature screening process can be found in Figure 1. Furthermore, a manual search of reference lists from review papers and articles in the final review was carried out. Three independent reviewers assessed papers for inclusion at three stages: title assessment, abstract assessment, and full paper assessment.
2.2 Selection criteria
The inclusion criteria for the studies focused on the role of exercise and myokines in delaying musculoskeletal aging and improving cognitive health. Studies had to be published in English language peer-reviewed journals and could include original research, randomized controlled trials, observational studies, cohort studies, and case‒control studies conducted on human subjects. The intervention had to involve exercise as the primary stimulus for myokine release, with reported quantitative outcomes related to musculoskeletal aging (e.g., muscle mass, strength, exercise performance) and/or mild cognitive impairment (e.g., cognitive function, memory). Attempts were made to contact the authors for additional information when possible. The exclusion criteria included animal and in vitro studies, as well as reviews; studies not directly focusing on the effects of exercise and myokines on musculoskeletal aging or cognitive health; studies with incomplete or unclear outcome reporting; and non-English language publications. Two reviewers independently screened titles, abstracts, and full texts of the articles for inclusion, with a third reviewer providing input in cases of disagreement.
2.3 Data extraction
Data extraction was performed systematically by two independent reviewers via a standardized data extraction form. The following information was extracted from each study: author(s) and publication year; study design (RCT, observational, cohort, case‒control); sample size and participant characteristics; exercise intervention details (type, intensity, duration, frequency); measured outcomes related to musculoskeletal aging and mild cognitive impairment; statistical methods used for analysis; and key findings and conclusions. Any discrepancies in data extraction were resolved through discussion between the reviewers or by consulting a third reviewer.
2.4 Quality assessment
The Newcastle‒Ottawa Scale (NOS) was utilized to evaluate the quality of nonrandomized studies (observational, cohort, case‒control) in three domains: selection of study groups, comparability of groups, and ascertainment of the outcome of interest. Only studies with moderate to high quality scores, as determined by these criteria, were included in the final analysis.
2.5 Data analysis
Data analysis was conducted via narrative synthesis. The narrative synthesis involved summarizing the findings of each study and identifying common themes and patterns across studies. The results of the review were presented in a nonstructured format, including the main advancements in this topic.
3 Result
3.1 Study characteristics
The section detailing the study characteristics highlights the essential components of the systematic review methodology and the studies included, in accordance with PRISMA guidelines. Illustrated in Figure 1, the flowchart demonstrates the meticulous selection procedure employed to identify and screen relevant studies. This review incorporated a comprehensive search strategy designed to encompass all pertinent investigations assessing the potential of exercise to leverage myokines for delaying musculoskeletal aging and enhancing cognitive health. The search spanned several electronic databases without restrictions regarding publication dates or languages, thereby ensuring a diverse and up-to-date array of evidence. Musculoskeletal aging is defined as a process characterized by a reduction in skeletal muscle mass and functionality, typically resulting from the natural aging process and a decline in physical activity levels.
In addition, the studies included in this review had different designs, including randomized controlled trials (RCTs), observational studies, cohort studies, and case-control studies. To ensure the robustness of study results, NOS rigorously evaluates the study quality of non-randomized studies. Specifically, NOS is used to assess study group selection and comparability between groups and to identify exposures or outcomes in cohort and case-control studies. It is worth noting that the NOS score of all studies was 7 out of 9, indicating that the included retrospective cohort studies had acceptable bias. Furthermore, most of the studies included in this review were preclinical studies designed to investigate the role of myokines in delaying and ameliorating musculoskeletal aging. The studies are summarized in Table 1. This review includes sixteen studies, from which data extraction was systematically conducted by two independent reviewers using a standardized form. This approach was implemented to minimize errors and ensure consistency. Key information extracted from each study encompassed the author(s) and publication year, study design, sample size, participant characteristics, details of the exercise intervention (including type, intensity, duration, and frequency), outcomes measured in relation to musculoskeletal aging and mild cognitive impairment, and the statistical methods utilized for analysis. This rigorous methodology in data extraction and analysis ensures that the findings of the review are based on a thorough and precise synthesis of the available evidence. In summary, this review offers a comprehensive and reliable synthesis of the existing evidence regarding the potential of exercise to utilize myokines in mitigating musculoskeletal aging and enhancing cognitive health.
4 Discussion
4.1 Musculoskeletal aging is an independent risk factor for MCI, and vice versa
Meta-analyses of the studies published over the past decade have revealed a consistent two-fold increase in the likelihood of cognitive impairment among older adults with sarcopenia compared to those without this condition (Peng et al., 2020). Sarcopenia and cognitive impairment are significant contributors to disability in elderly individuals. For instance, in patients with AD, the prevalence of sarcopenia is considerably high even in the early stages of the disease. Moreover, advanced age, a reduced BMI, and a decreased Mini-Mental State Examination (MMSE) score are also linked to sarcopenia in both male and female patients with AD (Ogawa et al., 2018). One cross-sectional survey confirmed a positive correlation between sarcopenia and MCI in low- and middle-income countries (LMICs) (Jacob et al., 2021). Another previous study indicated that the scores on the Short Physical Performance Battery (SPPB) correlate positively with the risk of cognitive impairment in older individuals (Moon J. H. et al., 2016). Additionally, a slow gait has also been suggested as an early indicator of cognitive impairment and the onset of dementia (Verghese et al., 2007; Mielke et al., 2013). According to one study, cognitive impairment is linked to sarcopenia primarily due to its correlation with a slow gait. Cognitive impairments in elderly men, including issues with processing speed and executive functions, are connected to sarcopenia, as well as a slow and weak gait (Kim and Won, 2019). These findings suggest a strong relationship between sarcopenia and the cognitive impairment associated with AD and dementia, thereby highlighting the potential role of sarcopenia in the pathophysiology of these disorders.
Sarcopenia and cognitive impairment share certain common risk factors, such as cerebrovascular disease, diabetes, and hypertension. Studies have shown that following a Mediterranean diet rich in high-quality protein may reduce the risk of developing MCI (Wu et al., 2019). However, a lack of physical activity due to a sedentary lifestyle and a lack of exercise in patients with sarcopenia can further reduce cognitive function and stability in these patients (Witham et al., 2020). In summary, sarcopenia is a potential risk factor for cognitive impairment and a potential predictor of the likelihood of cognitive impairment. However, further investigations are warranted to identify the causal association between sarcopenia and MCI.
4.2 Musculoskeletal aging and MCI: overlapping risk factors and potential mechanisms
Skeletal muscle atrophy and cognitive impairment are frequently observed in aging individuals, suggesting a potential connection between the two. While the negative impacts of muscle loss on daily functioning and wellbeing are well documented, the specific biological pathways linking sarcopenia and cognitive decline are not fully understood. However, the potential physiological mechanisms common between the two include nutritional deficiencies, hormonal imbalances, mitochondrial dysfunction, chronic inflammation, and irregularities in autophagy (Figure 2).
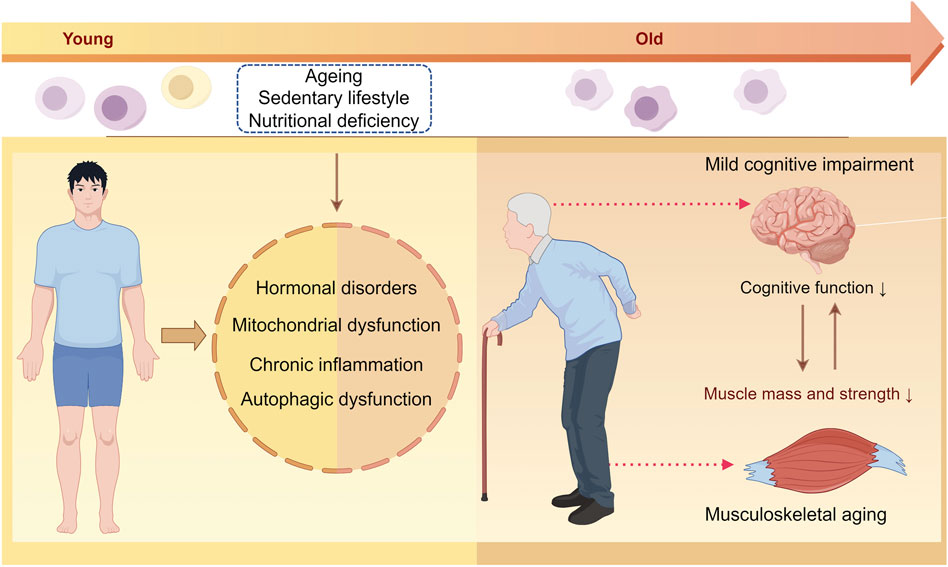
Figure 2. Illustration of the potential mechanisms underlying musculoskeletal aging and mild cognitive impairment. With aging, factors such as a sedentary lifestyle, insufficient physical activity, and nutritional deficiencies can lead to hormonal imbalances, mitochondrial dysfunction, chronic inflammation, and impaired autophagy in both the skeletal muscles and brain tissues. These changes ultimately affect the maintenance of the skeletal muscle mass and impact cognitive function.
4.2.1 Hormonal disorders
The dysregulation of endocrine hormones, such as insulin-like growth factor (IGF), growth hormone (GH), testosterone, estrogen, and cortisol, during aging is closely associated with sarcopenia and cognitive impairment (Zeng et al., 2020; Dabin et al., 2022). A decrease in IGF expression with advancing age leads to limited IGF/PI3K/Akt signaling-mediated protein synthesis, a reduction in the number of satellite cells available for damage repair, and ultimately, a decrease in muscle mass and strength (Serra et al., 2007). IGF-1 plays a crucial role in preventing cell death, promoting neurogenesis in the hippocampus, facilitating the normal phosphorylation of tau proteins, and clearing Aβ. Therefore, reduced IGF-1 levels in the bloodstream of patients with AD have been linked to increased Aβ deposition in the brain of these individuals (Westwood et al., 2014). GH, on the other hand, promotes muscle growth directly via GHR or indirectly by stimulating IGF-1 production. In the brain, GH activation in the hypothalamus triggers the release of GH-releasing hormone (GHRH), leading to elevated circulating levels of IGF-1, thereby supporting neuronal growth and survival, regulating tau protein phosphorylation, and counteracting the negative effects of Aβ in vivo (Winston et al., 2018). Furthermore, in the context of aging, a decrease in the level of testosterone, a critical sex hormone, has been associated with a decrease in muscle mass and strength (Mouser et al., 2016). Testosterone also plays a role in inhibiting SMAD-mediated protein degradation through the ubiquitin-proteasome system, thus reducing protein atrophy. While age-related decreases in testosterone levels appear to increase disease risk in humans, estrogen activates dormant muscle satellite cells, aiding in the repair of damaged muscle tissues (Morley, 2017a; Liao et al., 2019). However, compared to testosterone, estrogen, which possesses potential neuroprotective functions, appears to have a reduced ability to protect microglia from Aβ toxicity and promote microglial Aβ clearance (Keyvani et al., 2018).
In addition to anabolic hormones, glucocorticoids, particularly cortisol, also play a significant role in influencing human skeletal muscles (Kaplan and Shimizu, 1963). Cortisol levels are regulated at both the systemic and tissue levels to maintain glucocorticoid homeostasis. Elevated cortisol levels can result in muscle atrophy by increasing proteolysis through the ubiquitin-proteasome and lysosome systems (Sato et al., 2017). Additionally, glucocorticoids can decrease muscle protein synthesis by inhibiting IGF-I signaling and increasing myostatin signaling. This dual effect of cortisol on the muscles can impact muscle function and overall muscle health (Schakman et al., 2013). Furthermore, studies have revealed a correlation between plasma cortisol levels and cognitive impairment in individuals with AD, suggesting that the hyperactivity of the hypothalamic-pituitary-adrenal axis, which leads to elevated cortisol levels, may contribute to the progression of cognitive decline in these individuals (Zvěřová et al., 2013; Popp et al., 2015). Moreover, cortisol levels tend to increase with age, and higher levels of cortisol are associated with poorer cognitive function (Roelfsema et al., 2017; Qiu et al., 2021). These findings highlight the importance of understanding the impact of hormone levels on both muscle health and cognitive function, especially in the context of aging and neurodegenerative diseases.
4.2.2 Mitochondrial dysfunction
The increased production of reactive oxygen species (ROS) during aging is associated with increased damage to biomolecules (Radak et al., 2016). This increase in oxidative damage results in the development of different pathophysiological states, such as aging-related skeletal muscle loss and neurodegenerative disorders (Naudí et al., 2007; Tang et al., 2019). Additionally, the decrease in antioxidant activity with aging leads to the activation of nuclear factor kappa-B (NF-κB) and forkhead box O (FoxO) through the ROS pathways, thus triggering apoptosis and protein degradation in skeletal muscles. These transcription factors subsequently promote the expression of the muscle atrophy F-box protein (MAFbx/Atrogin-1) and the muscle-specific ring finger protein 1 (MuRF1), ultimately resulting in skeletal muscle atrophy.
Compromised mitochondrial function leads to the expression of oxidative stress markers, such as IL-6, CRP, and IL-1RA, which are closely associated with reduced physical function and muscle strength in elderly individuals (Bellanti et al., 2018). Therefore, the maintenance of mitochondrial health is crucial for the viability and functioning of neurons, as their structural and functional integrity are closely intertwined (Rugarli and Langer, 2012). Moreover, the maintenance of normal mitochondrial function is essential for maintaining the stability of the internal environment in mammals, and any disruption in the ROS scavenging system during mitochondrial oxidative phosphorylation can lead to brain aging and neurodegenerative disorders (Bernardo et al., 2013). Recent studies have highlighted a strong relationship between oxidative stress, aging, and neurodegenerative conditions. These findings suggest that the accumulation of Aβ triggers detrimental cellular changes, including oxidative damage to the mitochondria (Bozner et al., 1997; Pappolla et al., 1998). However, the precise mechanisms by which Aβ operates in vivo are not fully understood. Some studies have proposed that the translocase of the outer mitochondrial membrane 40 (TOMM40) plays a role in regulating the entry of Aβ into the mitochondria through the Tom40 outer membrane pore, underscoring its role as a potential target for the treatment of cognitive impairment (Yu et al., 2007). Collectively, these reports suggest that the restoration of mitochondrial function is crucial for the effective management of nerve disorders, especially cognitive dysfunction.
4.2.3 Chronic inflammation
Aging is commonly associated with a chronic low-grade inflammation known as inflammaging, which results in an elevation in the levels of inflammatory factors, which in turn cause the heightened metabolic degradation of skeletal muscles (Sato et al., 2016). Multiple potential mechanisms underlie chronic low-grade inflammation and its negative impacts on muscle health during the aging process. Age-related redox imbalances can lead to the upregulation of pro-inflammatory cytokines, which are key players in inflammatory pathways. Additionally, ROS can trigger inflammation, causing the release of TNF-α, leptin, and GH, and this cascade ultimately results in insulin resistance, accelerated muscle breakdown, and the loss of muscle mass (Thoma and Lightfoot, 2018). Proinflammatory cytokines, on the other hand, can counteract the anabolic effects of GH and IGF-1, causing a negative muscle protein balance, thereby affecting muscle function and strength (Kim et al., 2018). Recent evidence suggests that age-related chronic inflammation is correlated with elevated levels of circulating biomarkers, such as TNF-alpha, IL-1beta, IL-6, COX-2, iNOS (Chung et al., 2009). The findings reveal that this inflammatory response contributes to increased muscle breakdown and depletion through various pathophysiological pathways, thus contributing significantly to the aging of skeletal muscles (Kedlian et al., 2024). TNF-α, another key cytokine, has also been implicated in chronic inflammation and the onset of neurodegeneration (Hofman et al., 1989; Mogi et al., 1994). Furthermore, inflammatory cytokines have been shown to interfere with insulin function at the cellular level and worsen its neurotoxic effects, thus exacerbating cognitive decline (Fava et al., 2017). Therefore, chronic inflammation may serve as a shared pathogenic factor for aging-associated muscular dystrophy as well as MCI.
4.2.4 Nutritional deficiency
Aging-associated muscular dystrophy is a distinct disease associated with malnutrition. Reduced dietary intake and protein synthesis in patients with cognitive impairment can result in muscle loss, exacerbating the development of skeletal muscle atrophy (Cederholm et al., 2017). Malnutrition in elderly individuals, on the other hand, can result in decreased physical fitness and muscle strength and fitness, thus creating a vicious cycle. Inadequate nutritional intake can downregulate the Akt/mTORC1 signaling pathway, thus reducing muscle protein synthesis by inhibiting the phosphorylation of the downstream markers p70S6K and rpS6, and ultimately resulting in sarcopenia (Margolis et al., 2016). Inadequate nutrient intake can also increase oxidative stress or inhibit antioxidant mechanisms, contributing to the onset and progress of dementia. Evidence suggests that decreased food intake and activity in elderly individuals can cause vitamin D deficiency, which in turn impacts muscle protein synthesis and its metabolism through disturbances in the calcium and phosphorus levels and via insulin imbalance, ultimately leading to the development of sarcopenia (Ceglia et al., 2010). Moreover, since vitamin D and physical activity act synergistically and promote muscle protein ubiquitination and degradation, physical inactivity and vitamin D deficiency can worsen muscle atrophy (Yang et al., 2020). Furthermore, the risk of cognitive impairment is also greater in individuals with vitamin D deficiency, as vitamin D regulates calcium balance and Aβ deposition and displays antioxidant and anti-inflammatory properties in patients with AD.
4.2.5 Autophagic dysfunction
Autophagy plays a crucial role in breaking down dysfunctional organelles and damaged macromolecules in aging cells, thus aiding in the maintenance of the internal environment of the muscle cells. Evidence suggests that the inhibition of autophagy in skeletal muscles can lead to neuromuscular synaptic issues and reduced muscle strength, potentially impacting the quality of life and the lifespan of animals (Carnio et al., 2014). This close association between autophagy and aging results in reduced autophagy or disrupted autophagic flux, leading to the excessive degradation of muscle proteins, thus exacerbating skeletal muscle loss. The age-associated reduction in neuronal autophagy, on the other hand, hinders mitochondrial renewal, causing the accumulation of impaired mitochondria, accelerated cell death, and inflammation (Sheng et al., 2010). Mounting evidence indicates that the interplay between cellular autophagy and mitochondrial dysfunction can contribute to the onset of various age-associated disorders (Green et al., 2011).
Similar to replicating cells, neurons, which are terminally differentiated post-mitotic cells during early development, do not possess the ability to eliminate misfolded proteins and damaged organelles through cell division (Metaxakis et al., 2018). Therefore, the dysfunction of the autophagic lysosomal degradation process disrupts neuroendocrine homeostasis, causes lipofuscin accumulation, inhibits autophagy, and establishes a detrimental cycle. Evidence suggests that the accumulation of autophagosomes in the hippocampus of aged mice due to reduced degradation is possibly linked with cognitive impairment (Soontornniyomkij et al., 2012). In addition, brain aging is also characterized by a gradual decline in neuronal autophagic lysosome function, which plays a crucial role in the progression from normal aging to pathological aging, ultimately resulting in neurodegeneration in patients with AD (Ling and Salvaterra, 2011). These findings suggest that impaired autophagy can contribute significantly to cognitive decline in elderly individuals.
4.3 Crosstalk between the muscles and the brain in musculoskeletal aging and MCI
Exercise stimulates the production and autocrine secretion of myokines, which regulate muscle health (Boström et al., 2012; Eaton et al., 2018; Cai et al., 2019). With age and decreased physical activity, there is a decline in skeletal muscle mass, which leads to reduced myokine secretion and a detrimental cycle. Conversely, physical activity has a neuroprotective effect, counteracting the cognitive decline caused by neurodegenerative processes. Notably, physical activity may have fewer side effects compared to the therapies currently in use for neurodegenerative disorders. The myokines released during physical activity can improve cognitive function, memory, neuroplasticity, appetite, and mood, and reduce neuroinflammation through peripheral mechanisms. IL-6, IGF-1, BDNF, CTSB, irisin, and LIF are some of the key myokines that jointly participate in the regulation of musculoskeletal aging and MCI (Figure 3).
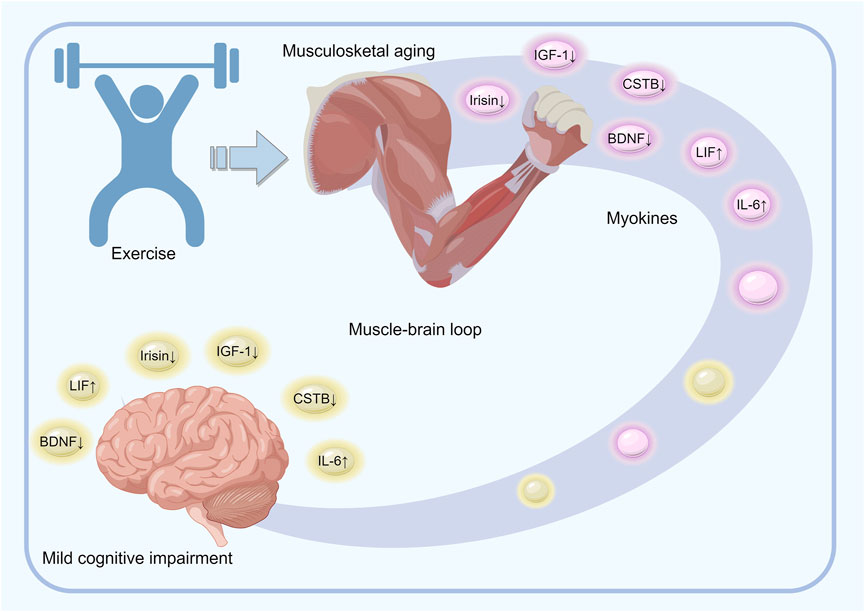
Figure 3. Overview of the mechanisms underlying the role of exercise-regulated myokines in musculoskeletal aging and mild cognitive impairment. Physical activity induces the release of various muscle-derived factors, such as IL-6, IGF-1, BDNF, CTSB, irisin, and LIF, into the bloodstream during muscle contraction. These factors either circulate freely or are enclosed in small vesicles that are capable of crossing the blood-brain barrier and impacting the cognitive and executive brain regions. The brain, on the other hand, modulates muscle activity and peripheral metabolism by directly influencing the target tissues and regulating cortisol levels through the hypothalamic–pituitary–adrenal axis. Effective coordination between the brain and muscles is thus essential for maintaining brain function, preventing cognitive decline, and preserving muscle mass.
4.3.1 IL-6
Prolonged exercise leads to the production and release of IL-6, which is classified as a myokine, by the skeletal muscles. However, the release of IL-6 during exercise is associated with muscle damage (Bruunsgaard et al., 1997), and increased levels of circulating IL-6 have been linked to mortality in healthy elderly individuals (Harris et al., 1999). In a previous study, individuals with elevated levels of both IL-6 and CRP exhibited increased fibrinogen levels, indicating the potential presence of a chronic low-level inflammation associated with aging (Cohen et al., 1997). While elevated levels of IL-6 in the plasma lead to skeletal muscle atrophy through the STAT3/5 signaling pathway, the inhibition of the IL-6 receptor reduces muscle atrophy by suppressing the expression of MuRF1 (Yakabe et al., 2018). Therefore, IL-6 not only impedes muscle growth and disrupts energy balance but also actively contributes to muscle breakdown and subsequent atrophy (Goodman, 1994; Tsujinaka et al., 1995). Studies on transgenic mice with increased expression levels of human IL-6 have demonstrated significant muscle wasting in the mice by 10 weeks of age, along with the activation of lysosomal enzymes and increased expression of proteasomal subunits (Tsujinaka et al., 1995; Tsujinaka et al., 1996). However, the inhibition of IL-6 signaling by the prolonged administration of an IL-6R antibody led to the reversal of the muscular alterations observed in the IL-6 transgenic mice, and the alleviation of muscle atrophy in wild-type mice with tumors. Various studies have indicated that the Otago exercise program (Gde Agung Mahendra et al., 2022) and moderate-intensity strengthening resistance exercise (Heo and Jee, 2024) effectively decrease the risk of falls among elderly individuals by increasing muscle strength and stability and lowering the levels of IL-6.
IL-6, a key player in the early stages of amyloid plaque formation in the brain of patients with AD (Huell et al., 1995), has also been linked to tau phosphorylation, synapse loss, and cognitive impairment in mouse models of AD (Quintanilla et al., 2004). Despite certain conflicting opinions from the academic community, previous meta-analyses have shown that IL-6 levels are elevated in both the cerebrospinal fluid (CSF) and the plasma of individuals with MCI and AD compared to that in the CSF and plasma of control subjects (Brosseron et al., 2014). In individuals with AD, the elevation in the levels of IL-6 in both the brain and the bloodstream is associated with the severity of dementia (Huell et al., 1995; Kálmán et al., 1997). However, recent research has shown that the neutralization of IL-6 and the inhibition of the STAT3 signaling pathway in the brain of AD mouse models can improve memory impairment (Lyra et al., 2021). Despite its proinflammatory properties, IL-6 displays different effects during physiological and pathological conditions. One study revealed that the positive effects of Nordic walking are manifested by a decreased concentration of proinflammatory proteins such as HMGB1 and IL-6 and increased irisin (Gmiat et al., 2017). IL-6 can be considered both a pro-inflammatory protein and an anti-inflammatory protein. Previous research has reported significant variations in IL-6 levels in response to exercise, which are influenced by factors such as intensity, duration, working muscle mass, and an individual’s endurance capacity (Febbraio and Pedersen, 2005; Petersen and Pedersen, 2005). Overall, regular exercise has been associated with a significant reduction in inflammation by decreasing the circulating levels of specific inflammatory markers, including IL-6 (Bautmans et al., 2021).
4.3.2 IGF-1
IGF-1 plays a crucial role in promoting mesenchymal stem cell differentiation and migration, as well as enhancing muscle fiber proliferation, differentiation, and contraction. IGF-1 also contributes to nerve remodeling and skeletal muscle regeneration by acting as a powerful neurotrophic factor that supports nerve regeneration and facilitates neuromuscular repair (Apel et al., 2010). The IGF-1/Akt/mTOR signaling pathway is known to regulate skeletal muscle hypertrophy (Rommel et al., 2001), and higher levels of IGF-1 can inhibit the expression of the protein degradation markers Atrogin-1 and MuRF1, thereby alleviating skeletal muscle atrophy (Schulze et al., 2005). Nevertheless, research indicates that this age-related decrease in IGF-1 levels in the skeletal muscles can be partially counteracted by progressive resistance training (Urso et al., 2005).
In addition to its role in the growth and development of the body, IGF-1 plays a key role in the development and metabolism of the CNS. A recent study in male mice has shown that the overexpression of IGF-1 in the CNS can protect against age-related cognitive dysfunction (Farias Quipildor et al., 2019). For example, in a rat model of sporadic Alzheimer’s disease (sAD), adenovirus-mediated IGF-1 gene transfer into the brain led to an increase in the levels of synaptic markers, leading to enhanced hippocampal synaptic plasticity, thereby improving recognition and spatial memory (Zappa Villar et al., 2021). In humans, on the other hand, IGF-1 deficiency results in cognitive dysfunction, which can be improved by interventions that increase circulating IGF-1 levels (Sonntag et al., 2013). Additionally, exercise has also been found to counteract the negative effects of MCI by regulating the serum IGF-1 levels (Tsai C. L. et al., 2018). In aging rats, an 8-week swimming training program led to the activation of the IGF-1/PI3K/Akt signaling pathway in the hippocampus, resulting in the reduced apoptosis of the hippocampal neurons and improved hippocampal function (Lin et al., 2020). In humans, while studies on older women have shown that 16 weeks of aquatic exercise and 24 months of progressive resistance exercise can increase serum IGF-1 levels (Kang et al., 2020; Molina-Sotomayor et al., 2020), a study on 30 elderly males demonstrated that strength and endurance training can elevate the serum IGF-1 levels and enhance cognitive function in elderly individuals (Arazi et al., 2021).
4.3.3 BDNF
BDNF, a protein belonging to the neurotrophic family, is primarily found in the brain and skeletal muscles and is vital for the development and differentiation of myoblasts and myofibers. It has also been detected in various tissues and body parts, including the periphery and the CNS, suggesting the presence of a neurotrophic connection between the different systems of the body and the blood-brain barrier (BBB) (Ribeiro et al., 2021). The level of BDNF decreases during myoblast maturation and myogenic differentiation, thus impacting the satellite cells or muscle progenitor cells (MPCs) and supporting the early differentiation of myoblasts (Mousavi and Jasmin, 2006). A study on a murine injury model revealed that BDNF functions via the tropomyosin-related kinase-B receptor (TrkBR) and the p75 neurotrophin receptor (p75NTR), both of which are markers of highly differentiated muscle precursor cells, thus enhancing myogenesis and myofiber maturation. Moreover, the expression of BDNF in the muscle satellite cells is upregulated during muscle injury, thereby facilitating the activation of the satellite cells (Yarrow et al., 2010).
BDNF also plays a crucial role in the CNS, overseeing neuronal survival, growth, and maintenance. It also regulates synaptic plasticity, cell survival, and brain cell differentiation (Aguado et al., 2003). Studies in mice have shown that BDNF positively affects brain function by promoting hippocampal cell regeneration, increasing BDNF gene expression, enhancing spatial memory, improving motor performance, and preserving the overall functionality of the brain (Marlatt et al., 2012). BDNF also acts as a key factor in exercise-induced neuroprotection, mediating the changes in synaptic strength and enhancing neurotransmitter release. Even in case of advanced memory impairment, cardiovascular exercise has been found to elevate BDNF levels, which in turn enhance hippocampal neuroplasticity and memory function in mice across various age groups from young to old (Tsai S. F. et al., 2018). Both human and animal studies have shown that acute/long-term cardiovascular/resistant exercise elevates circulating BDNF levels. In women, high-intensity cardiovascular exercise results in increased insulin sensitivity and reduced circulating levels of cortisol and BDNF, along with cognition-enhancing effects that are most pronounced for executive control tasks (Baker et al., 2010). In aging rats, cardiovascular exercise and strength training improve spatial memory by inducing neuroplasticity through distinct molecular mechanisms. Both exercise protocols also led to an increase in BDNF levels after training. While cardiovascular exercise specifically increased the levels of glutamatergic proteins (the NMDA receptor and PSD-95), strength training increased the levels of PKCα and the pro-inflammatory factors TNF-α and IL-1β (Vilela et al., 2017). These results suggest that cardiovascular exercise can positively impact brain functionality by affecting BDNF levels.
4.3.4 Irisin
A study from 2012 revealed that prolonged exercise training in mice led to a significant increase in the expression of PGC-1α in the muscles, which in turn led to the enhanced expression of the target gene, FNDC5, resulting in the production of irisin, a myokine that converts white adipose tissue to brown fat (Boström et al., 2012). Irisin is a novel cytokine that is primarily found in the brain and skeletal muscles. Although its secretion decreases with age, it can be partially restored by resistance exercise training (Kim et al., 2015). Generally, individuals with sarcopenia exhibit lower levels of skeletal muscle protein biomarkers, including irisin, compared to those without sarcopenia (Yen et al., 2022). Irisin, an exercise-induced polypeptide secreted by skeletal muscles, crosses the blood-brain barrier and brings about certain exercise-mediated effects in the brain. Previous studies have shown a positive correlation between irisin levels and aging-induced cognitive dysfunction. The primary pathological process of AD involves the over-accumulation of Aβ in the brain due to an imbalance in Aβ production and clearance (Lourenco et al., 2019). This excessive accumulation of Aβ leads to neurotoxicity, resulting in a decrease in the number of neurons, loss of synapses, and impaired nerve signal conduction, ultimately leading to cognitive impairment. Notably, FNDC5, the irisin precursor, has been found in various regions of the brain and is associated with neural differentiation (Hashemi et al., 2013). Physical exercise has been shown to enhance FNDC5 expression (Wrann et al., 2013) and irisin levels (Siteneski et al., 2018) in the hippocampus. In a study involving rats, the administration of Aβ1‒42 to the hippocampus led to spatial learning and memory impairments, along with reduced hippocampal FNDC5 expression. However, 4 weeks of moderate-intensity running exercise increased the hippocampal FNDC5 mRNA levels and improved spatial learning and memory loss in the rats.
4.3.5 Cathepsin B
Cathepsin B (CTSB), which is expressed in various tissues, including the skeletal muscles and the brain, can play a crucial role in brain flexibility. Moon et al. identified CTSB as a myokine in a study utilizing rat myotubes treated with the AMPK agonist AICAR (Moon H. Y. et al., 2016). Typically, physical activity increases the CTSB level in the blood and the CTSB gene expression in the hippocampus, indicating that CTSB impacts brain function both directly and indirectly. In mice, the deletion of the CTSB gene prevented the exercise-induced improvement in spatial memory retention and adult neurogenesis, reduced the inhibitory signals transmitted to dentate granule cells (GCs), and reduced the levels of hippocampal P11, a crucial protein necessary for the effect of CTSB on the differentiation and migration of neurons (Moon H. Y. et al., 2016). Additionally, CTSB also possessed the ability to penetrate the blood-brain barrier. While CTSB administration did not affect hippocampal cell proliferation in the mice, it increased the BDNF mRNA and protein expression levels. Additionally, CTSB treatment also elevated the level of doublecortin, a protein known for its neuroprotective effect and ability to promote neuronal migration.
CTSB is a potential therapeutic target for neurodegenerative diseases such as AD. Plasma levels of CTSB increased after adults at high risk for AD participated in 26 weeks of moderate-to-vigorous intensity cardiovascular exercise, with this alteration in CTSB positively correlated with cognitive performance (Gaitán et al., 2021). In a mouse model of AD, CTSB was found to reduce the levels of Aβ42 and improve behavioral abnormalities (Hwang et al., 2019). Conversely, another study revealed a correlation between an increase in the plasma CTSB levels and the progression of MCI and AD from the mild to the severe forms of the disease, with the pathological worsening of the disease being mediated by protein accumulation (Morena et al., 2017). However, CTSB also possesses neuroprotective and anti-amyloidogenic properties (Mueller-Steiner et al., 2006), and mice studies have revealed neuronal loss and brain atrophy in double-KO mice lacking both CTSB and CTSL (Felbor et al., 2002). Additionally, CTSB may also be involved in the exercise-mediated enhancement of hippocampal neurogenesis, memory, and learning. However, further research is needed to determine the extent to which this myokine influences the exercise-mediated improvement in cognitive function in humans.
4.3.6 LIF
LIF was first identified as a factor that promotes macrophage differentiation. It is produced by cardiac, neural, and skeletal muscles and acts as a versatile myokine (Hilton, 1992). LIF plays a role in controlling the proliferation and differentiation of satellite cells, which are crucial for muscle hypertrophy (Spangenburg and Booth, 2006). Studies have shown that LIF stimulates human myoblast proliferation and induces JunB and c-Myc expression in human myotubes. Conversely, the suppression of the LIF receptor has been shown to reduce myoblast proliferation (Broholm et al., 2011). LIF also has the ability to cross the blood‒brain barrier and enter the nervous system, where it plays a role in astrocyte development and oligodendrocyte survival. Treatment of mouse hippocampal cells with LIF has been found to activate the Akt/mTOR and STAT3 signaling pathways, downregulate LC3-II, increase c-fos expression (a marker of neuronal activation), and enhance cell survival. Similar results have also been observed in a Drosophila model of AD wherein LIF was found to inhibit LC3-II (Lee et al., 2019). Multiple studies have revealed elevated LIF levels in patients with AD, suggesting a possible connection between Aβ and the initiation of LIF-induced inflammation (Soilu-Hänninen et al., 2010). Theoretically, Aβ acts as a trigger for LIF, leading to an inflammatory response in individuals with AD. However, LIF also possesses neuroprotective properties, which it exerts by inducing oligodendrocyte apoptosis via TNF-α (Vanderlocht et al., 2006). In a drug trial involving rats with CNS injury, LIF was found to boost neurotrophin expression and facilitate corticospinal axon growth (Pan et al., 2000). However, owing to its potential instability, the impact of LIF on neural tissues was limited to a short duration. Further exploration is needed to address these limitations and establish LIF as a promising therapeutic option for the treatment of AD.
4.4 Optimal exercise prescription for myokine secretion and function
Previous research has confirmed that different types of physical activities affect skeletal muscle metabolic processes via unique mechanisms. Similarly, the secretion of myokines is also influenced by the specific nature of the physical activity (Little et al., 2018). The myokines produced during exercise have been shown to impact the muscle-brain communication surrounding musculoskeletal aging and MCI. Nevertheless, the secretion patterns of many other myokines remain somewhat unclear and are subject to various influences, including the intensity, type, and duration of exercise, as well as individual traits. It appears that exercise can trigger incremental changes ranging from minor to substantial, with peak levels observed immediately post-workout and within the first hour, followed by a return to baseline levels between 180 min and 24 h post-exercise (Bettariga et al., 2024). Both age and training status influence the myokine response to an acute bout of blood-flow restricted resistance exercise (Cordingley et al., 2023). Research on resistance exercise in individuals susceptible to probable MCI has revealed higher levels of IL-6 in blood markers than in controls do, particularly in older adults at high risk of MCI (Vints et al., 2024). A possible interpretation for the increase in IL-6 could be that resistance exercise was too intense for the participating older adults (Morishita et al., 2019). Additionally, sex appears to be a significant determinant of the IGF-1 response to exercise training. Research has indicated that older women exhibit variations in IGF-1 levels following elastic band resistance exercise (Hofmann et al., 2016), whereas younger men show increased IGF-1 levels in response to strength training programs (Takano et al., 2005). This discrepancy may be attributed to the fact that, with aging, persistent inflammation can inhibit the GH/IGF-1 axis (Schmidt et al., 2011). Additionally, while it is known that BDNF can traverse the blood-brain barrier, the release of myogenic BDNF into the bloodstream and its impact on the brain are still debatable (Rasmussen et al., 2009). Conversely, other myokines such as CTSB and irisin, which are regulated by exercise, are released into the bloodstream and cross the blood-brain barrier to increase the brain BDNF levels (Moon H. Y. et al., 2016; Momenzadeh et al., 2022). Maass et al. (2016) demonstrated that the inhibition of the IGF-1 receptor leads to the suppression of the exercise-induced upregulation of pro-BDNF and BDNF expression in the hippocampus, indicating that IGF-1, an upstream factor of BDNF, plays a role in hippocampal neurogenesis and BDNF gene regulation. In an 8-week study comparing cardiovascular and resistance exercise, resistance exercise alone was found to activate the IGF-1/Akt signaling pathway, enhancing hippocampal synaptic plasticity. This study revealed that the molecular mechanisms underlying hippocampal synaptic plasticity varied depending on the type of exercise (Cassilhas et al., 2012).
Different types of exercise may have different effects on the blood IGF-1 levels and facilitate the transport of IGF-1 from the bloodstream to the brain. For instance, a study that tracked IGF-1 levels overnight after intense resistance training revealed that the IGF-1 levels remained unaltered overnight (Nindl et al., 2001). In contrast, a lower-intensity resistance exercise routine may be more advantageous for older adults who prefer to avoid exerting greater effort and wish to mitigate potential injuries associated with heavier loads. Additionally, increasing the total load can be more easily achieved by increasing the number of repetitions at lower intensities (Cornish et al., 2018). It is crucial to consider the duration and intensity of exercise when aiming to increase blood IGF-1 levels, especially when dealing with resistance exercise, to prevent injuries in elderly individuals or patients. Although exercise is a recognized method for promoting good health and maintaining normal brain function and research shows that physical activity can have positive effects on human health and help individuals with conditions such as MCI and AD, caution is important, as high-impact and high-intensity exercise can have negative consequences. Studies indicate that engaging in low-intensity exercise, rather than high-intensity exercise, is more beneficial for protecting and revitalizing the aging brain in individuals with AD (Kim et al., 2003). It is important to consider the duration and intensity of exercise when aiming to increase blood IGF-1 levels, especially when dealing with resistance exercise, to prevent injuries in elderly individuals or patients. Although exercise is a recognized method for promoting good health and maintaining normal brain function and research shows that physical activity can have positive effects on human health and help individuals with conditions such as MCI and AD, caution is important, as high-impact and high-intensity exercise can have negative consequences. Studies indicate that engaging in low-intensity exercise, rather than high-intensity exercise, is more beneficial for protecting and revitalizing the aging brain in individuals with AD (Kim et al., 2003). Additionally, certain studies have indicated that cardiovascular and resistance training may have a more significant impact on cognitive function in individuals with MCI than multimodal exercise interventions do. Therefore, incorporating cardiovascular or resistance training into exercise regimens could serve as an effective strategy to mitigate cognitive decline in this population (Akalp et al., 2024). Consequently, implementing suitable exercise might orchestrate the interplay between the various myokines and provide health benefits.
Exercise, as an effective non-pharmacological intervention, can impact multiple organs of the body simultaneously. Different organs and tissues respond to exercise at the same time, highlighting the intricate network of mechanisms involved. While neuro-endocrine-immune network regulation is important, the communication between organs and tissues through exercise-induced myokines is also crucial. However, the relationship between physical activity parameters (such as type, intensity, and frequency) and the secretion of myokines remains unclear, impeding the development of targeted exercise programs. Future research should focus on understanding how physical activity influences the secretion of myokines and the effects of myokines on distant target organs. This knowledge will help establish connections between exercise, myokines, and chronic diseases, offering new insights into the health benefits of exercise. Moreover, it is essential to not only identify the role of individual myokines but also evaluate how different factors interact synergistically to impact the entire body. Furthermore, Various research studies have demonstrated the diverse nature of cognitive function changes with age, as some older individuals retain their cognitive abilities, while others experience mild or severe declines (Yaffe et al., 2009). Moving forward, artificial intelligence (AI) algorithms and biomarkers (D'Amico et al., 2020), such as physiological and myokines integrated into AI indices, hold promise in offering more precise and dependable approaches for identifying and addressing the biological markers of cognitive decline or sarcopenia across different age groups.
4.5 Limitation
This review examines the myokines that are known to play a significant role in the pathophysiology of musculoskeletal aging and mild cognitive impairment, aiming to inspire researchers to identify new strategies for developing drugs or interventions that mimic the health effects of exercise. Exploring the interactions between tissues and organs, one of the health benefits of exercise, along with their molecular mechanisms, will provide a solid foundation for creating personalized exercise prescriptions for musculoskeletal aging and mild cognitive impairment and is poised to become a leading focus in the field of exercise health studies. However, this review has certain limitations; the main limitation is that this is a narrative review rather than a complete systematic review. Nevertheless, this study represents the first attempt to investigate the relationships among myokines, aging-associated muscle atrophy, and cognitive dysfunction from an exercise perspective. There is a clear need for further research and a systematic review in this area. Only well-designed studies without methodological information will help expand our understanding of the connections between musculoskeletal aging, cognitive dysfunction due to aging, and their treatment implications.
5 Conclusion and outlook
The muscles and the brain, which are the primary organs responsible for locomotion, are interconnected systems that communicate with each other and share vital functions, including immunity and nutrition, in addition to locomotion. Their developmental processes are mutually regulated, with their crosstalk and the underlying mechanisms seen as complementary and reciprocal physiological mechanisms rather than independent functional regulations. Exercise acts as a mediator for the secretion of certain muscle-secreted factors, such as IL-6, IGF-1, BDNF, CTSB, irisin, and LIF, establishing a self-regulating loop between the brain and the muscles, thus enhancing the cognitive functions of the brain and the skeletal muscle functions.
Research on the regulation of skeletal muscle atrophy and mild cognitive impairment by myokines has gained significant attention in the fields of contemporary geriatrics and exercise physiology. Myokines may play an important role in the interplay between sarcopenia and cognitive function by regulating their pathogenesis and influencing clinical and pathological injuries. The identification of characteristic risk factors and markers for muscular atrophy in patients with mild cognitive impairment is a new direction. The myokines discussed here are only the tip of the iceberg, with some questions surrounding them requiring further clarification: the exact mechanisms underlying myokine release, the dose-effect relationship between exercise and exercise-induced myokine release, and the precise mechanisms underlying the regulation of the muscle-brain loop by myokines. Further research on the cross-talk in the muscle-brain axis will deepen the understanding of the mechanisms by which exercise promotes health and could pave the way for new avenues in exercise physiology research.
Author contributions
XG: Writing–original draft. YC: Writing–review and editing. PC: Writing–review and editing.
Funding
The author(s) declare that financial support was received for the research, authorship, and/or publication of this article. This work was supported by the Talent Introduction Project of Suzhou Vocational University in 2023 (No. 202305000021).
Conflict of interest
The authors declare that the research was conducted in the absence of any commercial or financial relationships that could be construed as a potential conflict of interest.
Publisher’s note
All claims expressed in this article are solely those of the authors and do not necessarily represent those of their affiliated organizations, or those of the publisher, the editors and the reviewers. Any product that may be evaluated in this article, or claim that may be made by its manufacturer, is not guaranteed or endorsed by the publisher.
References
Aguado F., Carmona M. A., Pozas E., Aguiló A., Martínez-Guijarro F. J., Alcantara S., et al. (2003). BDNF regulates spontaneous correlated activity at early developmental stages by increasing synaptogenesis and expression of the K+/Cl-co-transporter KCC2. Development 130 (7), 1267–1280. doi:10.1242/dev.00351
Akalp K., Ferreira J. P., Soares C. M., Ribeiro M. J., Teixeira A. M. (2024). The effects of different types of exercises on cognition in older persons with mild cognitive impairment: a systematic review and meta-analysis. Arch. Gerontol. Geriatr. 126, 105541. doi:10.1016/j.archger.2024.105541
Apel P. J., Ma J., Callahan M., Northam C. N., Alton T. B., Sonntag W. E., et al. (2010). Effect of locally delivered IGF-1 on nerve regeneration during aging: an experimental study in rats. Muscle Nerve 41 (3), 335–341. doi:10.1002/mus.21485
Arazi H., Babaei P., Moghimi M., Asadi A. (2021). Acute effects of strength and endurance exercise on serum BDNF and IGF-1 levels in older men. BMC Geriatr. 21 (1), 50. doi:10.1186/s12877-020-01937-6
Baker L. D., Frank L. L., Foster-Schubert K., Green P. S., Wilkinson C. W., McTiernan A., et al. (2010). Effects of aerobic exercise on mild cognitive impairment: a controlled trial. Arch. Neurol. 67 (1), 71–79. doi:10.1001/archneurol.2009.307
Bautmans I., Salimans L., Njemini R., Beyer I., Lieten S., Liberman K. (2021). The effects of exercise interventions on the inflammatory profile of older adults: a systematic review of the recent literature. Exp. Gerontol. 146, 111236. doi:10.1016/j.exger.2021.111236
Bellanti F., Romano A. D., Lo Buglio A., Castriotta V., Guglielmi G., Greco A., et al. (2018). Oxidative stress is increased in sarcopenia and associated with cardiovascular disease risk in sarcopenic obesity. Maturitas 109, 6–12. doi:10.1016/j.maturitas.2017.12.002
Bernardo T. C., Cunha-Oliveira T., Serafim T. L., Holy J., Krasutsky D., Kolomitsyna O., et al. (2013). Dimethylaminopyridine derivatives of lupane triterpenoids cause mitochondrial disruption and induce the permeability transition. Bioorg Med. Chem. 21 (23), 7239–7249. doi:10.1016/j.bmc.2013.09.066
Bettariga F., Taaffe D. R., Galvão D. A., Lopez P., Bishop C., Markarian A. M., et al. (2024). Exercise training mode effects on myokine expression in healthy adults: a systematic review with meta-analysis. J. Sport Health Sci. 13, 764–779. doi:10.1016/j.jshs.2024.04.005
Bobba-Alves N., Juster R. P., Picard M. (2022). The energetic cost of allostasis and allostatic load. Psychoneuroendocrinology 146, 105951. doi:10.1016/j.psyneuen.2022.105951
Booth F. W., Roberts C. K., Laye M. J. (2012). Lack of exercise is a major cause of chronic diseases. Compr. Physiol. 2 (2), 1143–1211. doi:10.1002/cphy.c110025
Boström P., Wu J., Jedrychowski M. P., Korde A., Ye L., Lo J. C., et al. (2012). A PGC1-α-dependent myokine that drives brown-fat-like development of white fat and thermogenesis. Nature 481 (7382), 463–468. doi:10.1038/nature10777
Bozner P., Grishko V., LeDoux S. P., Wilson G. L., Chyan Y. C., Pappolla M. A. (1997). The amyloid beta protein induces oxidative damage of mitochondrial DNA. J. Neuropathol. Exp. Neurol. 56 (12), 1356–1362. doi:10.1097/00005072-199712000-00010
Broholm C., Laye M. J., Brandt C., Vadalasetty R., Pilegaard H., Pedersen B. K., et al. (2011). LIF is a contraction-induced myokine stimulating human myocyte proliferation. J. Appl. Physiol. 111 (1), 251–259. doi:10.1152/japplphysiol.01399.2010
Brosseron F., Krauthausen M., Kummer M., Heneka M. T. (2014). Body fluid cytokine levels in mild cognitive impairment and Alzheimer's disease: a comparative overview. Mol. Neurobiol. 50 (2), 534–544. doi:10.1007/s12035-014-8657-1
Bruunsgaard H., Galbo H., Halkjaer-Kristensen J., Johansen T. L., MacLean D. A., Pedersen B. K. (1997). Exercise-induced increase in serum interleukin-6 in humans is related to muscle damage. J. Physiol. 499 (Pt 3), 833–841. doi:10.1113/jphysiol.1997.sp021972
Buchman A. S., Wilson R. S., Boyle P. A., Bienias J. L., Bennett D. A. (2007). Grip strength and the risk of incident Alzheimer's disease. Neuroepidemiology 29 (1-2), 66–73. doi:10.1159/000109498
Cai L., Tan M., Tan W., Zeng X., Wan N., Wong S. H., et al. (2019). Associations of circulating irisin concentrations with cardiometabolic risk factors among children vary by physical activity or sedentary time levels. Front. Endocrinol. (Lausanne) 10, 549. doi:10.3389/fendo.2019.00549
Carnio S., LoVerso F., Baraibar M. A., Longa E., Khan M. M., Maffei M., et al. (2014). Autophagy impairment in muscle induces neuromuscular junction degeneration and precocious aging. Cell Rep. 8 (5), 1509–1521. doi:10.1016/j.celrep.2014.07.061
Cassilhas R. C., Lee K. S., Fernandes J., Oliveira M. G., Tufik S., Meeusen R., et al. (2012). Spatial memory is improved by aerobic and resistance exercise through divergent molecular mechanisms. Neuroscience 202, 309–317. doi:10.1016/j.neuroscience.2011.11.029
Cederholm T., Barazzoni R., Austin P., Ballmer P., Biolo G., Bischoff S. C., et al. (2017). ESPEN guidelines on definitions and terminology of clinical nutrition. Clin. Nutr. 36 (1), 49–64. doi:10.1016/j.clnu.2016.09.004
Ceglia L., da Silva Morais M., Park L. K., Morris E., Harris S. S., Bischoff-Ferrari H. A., et al. (2010). Multi-step immunofluorescent analysis of vitamin D receptor loci and myosin heavy chain isoforms in human skeletal muscle. J. Mol. Histol. 41 (2-3), 137–142. doi:10.1007/s10735-010-9270-x
Chung H. Y., Cesari M., Anton S., Marzetti E., Giovannini S., Seo A. Y., et al. (2009). Molecular inflammation: underpinnings of aging and age-related diseases. Ageing Res. Rev. 8 (1), 18–30. doi:10.1016/j.arr.2008.07.002
Cohen H. J., Pieper C. F., Harris T., Rao K. M., Currie M. S. (1997). The association of plasma IL-6 levels with functional disability in community-dwelling elderly. J. Gerontol. A Biol. Sci. Med. Sci. 52 (4), M201–M208. doi:10.1093/gerona/52a.4.m201
Cordingley D. M., Anderson J. E., Cornish S. M. J. J. O.S. I. S., and (2023). Myokine response to blood-flow restricted resistance exercise in younger and older males in an untrained and resistance-trained state: a Pilot Study. J. Sci. Sport Exerc. 5 (3), 203–217. doi:10.1007/s42978-022-00164-2
Cornish S. M., Chase J. E., Bugera E. M., Giesbrecht G. G. (2018). Systemic il-6 and myoglobin response to three different resistance exercise intensities in older men. J. Aging Phys. Act. 26 (3), 451–456. doi:10.1123/japa.2017-0167
Cruz-Jentoft A. J., Bahat G., Bauer J., Boirie Y., Bruyère O., Cederholm T., et al. (2019). Sarcopenia: revised European consensus on definition and diagnosis. Age Ageing 48 (4), 601. doi:10.1093/ageing/afz046
Dabin R., Wei C., Liang S., Ke C., Zhihan W., Ping Z. (2022). Astrocytic igf-1 and igf-1r orchestrate mitophagy in traumatic brain injury via exosomal mir-let-7e. Oxid. Med. Cell Longev. 2022, 3504279. doi:10.1155/2022/3504279
D'Amico D., Amestoy M. E., Fiocco A. J. (2020). The association between allostatic load and cognitive function: a systematic and meta-analytic review. Psychoneuroendocrinology 121, 104849. doi:10.1016/j.psyneuen.2020.104849
Eaton M., Granata C., Barry J., Safdar A., Bishop D., Little J. P. (2018). Impact of a single bout of high-intensity interval exercise and short-term interval training on interleukin-6, FNDC5, and METRNL mRNA expression in human skeletal muscle. J. Sport Health Sci. 7 (2), 191–196. doi:10.1016/j.jshs.2017.01.003
Farias Quipildor G. E., Mao K., Hu Z., Novaj A., Cui M. H., Gulinello M., et al. (2019). Central IGF-1 protects against features of cognitive and sensorimotor decline with aging in male mice. Geroscience 41 (2), 185–208. doi:10.1007/s11357-019-00065-3
Fava A., Colica C., Plastino M., Messina D., Cristiano D., Opipari C., et al. (2017). Cognitive impairment is correlated with insulin resistance degree: the “PA-NICO-study”. Metab. Brain Dis. 32 (3), 799–810. doi:10.1007/s11011-017-9977-4
Febbraio M. A., Pedersen B. K. (2005). Contraction-induced myokine production and release: is skeletal muscle an endocrine organ? Exerc Sport Sci. Rev. 33 (3), 114–119. doi:10.1097/00003677-200507000-00003
Felbor U., Kessler B., Mothes W., Goebel H. H., Ploegh H. L., Bronson R. T., et al. (2002). Neuronal loss and brain atrophy in mice lacking cathepsins B and L. Proc. Natl. Acad. Sci. U. S. A. 99 (12), 7883–7888. doi:10.1073/pnas.112632299
Gaitán J. M., Moon H. Y., Stremlau M., Dubal D. B., Cook D. B., Okonkwo O. C., et al. (2021). Effects of aerobic exercise training on systemic biomarkers and cognition in late middle-aged adults at risk for alzheimer's disease. Front. Endocrinol. (Lausanne) 12, 660181. doi:10.3389/fendo.2021.660181
Gde Agung Mahendra I. D., Subadi I., Wardhani I. L., Satyawati R., Alit Pawana I. P., Melaniani S. (2022). Effects of otago exercise program on serum interleukin-6 level in older women. Ann. Med. Surg. (Lond) 78, 103733. doi:10.1016/j.amsu.2022.103733
Gmiat A., Mieszkowski J., Prusik K., Prusik K., Kortas J., Kochanowicz A., et al. (2017). Changes in pro-inflammatory markers and leucine concentrations in response to Nordic Walking training combined with vitamin D supplementation in elderly women. Biogerontology 18 (4), 535–548. doi:10.1007/s10522-017-9694-8
Goodman M. N. (1994). Interleukin-6 induces skeletal muscle protein breakdown in rats. Proc. Soc. Exp. Biol. Med. 205 (2), 182–185. doi:10.3181/00379727-205-43695
Green D. R., Galluzzi L., Kroemer G. (2011). Mitochondria and the autophagy-inflammation-cell death axis in organismal aging. Science 333 (6046), 1109–1112. doi:10.1126/science.1201940
Grundman M., Petersen R. C., Ferris S. H., Thomas R. G., Aisen P. S., Bennett D. A., et al. (2004). Mild cognitive impairment can be distinguished from Alzheimer disease and normal aging for clinical trials. Arch. Neurol. 61 (1), 59–66. doi:10.1001/archneur.61.1.59
Hansson O., Zetterberg H., Buchhave P., Londos E., Blennow K., Minthon L. (2006). Association between CSF biomarkers and incipient Alzheimer's disease in patients with mild cognitive impairment: a follow-up study. Lancet Neurol. 5 (3), 228–234. doi:10.1016/s1474-4422(06)70355-6
Harris T. B., Ferrucci L., Tracy R. P., Corti M. C., Wacholder S., Ettinger W. H., et al. (1999). Associations of elevated interleukin-6 and C-reactive protein levels with mortality in the elderly. Am. J. Med. 106 (5), 506–512. doi:10.1016/s0002-9343(99)00066-2
Hashemi M. S., Ghaedi K., Salamian A., Karbalaie K., Emadi-Baygi M., Tanhaei S., et al. (2013). Fndc5 knockdown significantly decreased neural differentiation rate of mouse embryonic stem cells. Neuroscience 231, 296–304. doi:10.1016/j.neuroscience.2012.11.041
Heo S. J., Jee Y. S. (2024). Intensity-effects of strengthening exercise on thigh muscle volume, pro- or anti-inflammatory cytokines, and immunocytes in the older adults: a randomized controlled trial. Arch. Gerontol. Geriatr. 116, 105136. doi:10.1016/j.archger.2023.105136
Hilton D. J. (1992). LIF: lots of interesting functions. Trends Biochem. Sci. 17 (2), 72–76. doi:10.1016/0968-0004(92)90505-4
Hofman F. M., Hinton D. R., Johnson K., Merrill J. E. (1989). Tumor necrosis factor identified in multiple sclerosis brain. J. Exp. Med. 170 (2), 607–612. doi:10.1084/jem.170.2.607
Hofmann M., Schober-Halper B., Oesen S., Franzke B., Tschan H., Bachl N., et al. (2016). Effects of elastic band resistance training and nutritional supplementation on muscle quality and circulating muscle growth and degradation factors of institutionalized elderly women: the Vienna Active Ageing Study (VAAS). Eur. J. Appl. Physiol. 116 (5), 885–897. doi:10.1007/s00421-016-3344-8
Huell M., Strauss S., Volk B., Berger M., Bauer J. (1995). Interleukin-6 is present in early stages of plaque formation and is restricted to the brains of Alzheimer's disease patients. Acta Neuropathol. 89 (6), 544–551. doi:10.1007/bf00571510
Hwang J., Estick C. M., Ikonne U. S., Butler D., Pait M. C., Elliott L. H., et al. (2019). The role of lysosomes in a broad disease-modifying approach evaluated across transgenic mouse models of Alzheimer's disease and Parkinson's disease and models of mild cognitive impairment. Int. J. Mol. Sci. 20 (18), 4432. doi:10.3390/ijms20184432
Jacob L., Kostev K., Smith L., Oh H., López-Sánchez G. F., Shin J. I., et al. (2021). Sarcopenia and mild cognitive impairment in older adults from six low- and middle-income countries. J. Alzheimers Dis. 82 (4), 1745–1754. doi:10.3233/jad-210321
Jensen C. S., Bahl J. M., Østergaard L. B., Høgh P., Wermuth L., Heslegrave A., et al. (2019). Exercise as a potential modulator of inflammation in patients with Alzheimer's disease measured in cerebrospinal fluid and plasma. Exp. Gerontol. 121, 91–98. doi:10.1016/j.exger.2019.04.003
Kálmán J., Juhász A., Laird G., Dickens P., Járdánházy T., Rimanóczy A., et al. (1997). Serum interleukin-6 levels correlate with the severity of dementia in Down syndrome and in Alzheimer's disease. Acta Neurol. Scand. 96 (4), 236–240. doi:10.1111/j.1600-0404.1997.tb00275.x
Kang D. W., Bressel E., Kim D. Y. (2020). Effects of aquatic exercise on insulin-like growth factor-1, brain-derived neurotrophic factor, vascular endothelial growth factor, and cognitive function in elderly women. Exp. Gerontol. 132, 110842. doi:10.1016/j.exger.2020.110842
Kaplan S. A., Shimizu C. S. (1963). Effects of cortisol on amino acids in skeletal muscle and plasma. Endocrinology 72, 267–272. doi:10.1210/endo-72-2-267
Kedlian V. R., Wang Y., Liu T., Chen X., Bolt L., Tudor C., et al. (2024). Human skeletal muscle aging atlas. Nat. Aging 4 (5), 727–744. doi:10.1038/s43587-024-00613-3
Keyvani K., Münster Y., Kurapati N. K., Rubach S., Schönborn A., Kocakavuk E., et al. (2018). Higher levels of kallikrein-8 in female brain may increase the risk for Alzheimer's disease. Brain Pathol. 28 (6), 947–964. doi:10.1111/bpa.12599
Kim B. J., Lee S. H., Kwak M. K., Isales C. M., Koh J. M., Hamrick M. W. (2018). Inverse relationship between serum hsCRP concentration and hand grip strength in older adults: a nationwide population-based study. Aging (Albany NY) 10 (8), 2051–2061. doi:10.18632/aging.101529
Kim H. J., So B., Choi M., Kang D., Song W. (2015). Resistance exercise training increases the expression of irisin concomitant with improvement of muscle function in aging mice and humans. Exp. Gerontol. 70, 11–17. doi:10.1016/j.exger.2015.07.006
Kim M., Won C. W. (2019). Sarcopenia is associated with cognitive impairment mainly due to slow gait speed: results from the Korean frailty and aging cohort study (kfacs). Int. J. Environ. Res. Public Health 16 (9), 1491. doi:10.3390/ijerph16091491
Kim Y. P., Kim H. B., Jang M. H., Lim B. V., Kim Y. J., Kim H., et al. (2003). Magnitude- and time-dependence of the effect of treadmill exercise on cell proliferation in the dentate gyrus of rats. Int. J. Sports Med. 24 (2), 114–117. doi:10.1055/s-2003-38202
Lee H. J., Lee J. O., Lee Y. W., Kim S. A., Seo I. H., Han J. A., et al. (2019). LIF, a novel myokine, protects against amyloid-beta-induced neurotoxicity via akt-mediated autophagy signaling in hippocampal cells. Int. J. Neuropsychopharmacol. 22 (6), 402–414. doi:10.1093/ijnp/pyz016
Liao Z. H., Huang T., Xiao J. W., Gu R. C., Ouyang J., Wu G., et al. (2019). Estrogen signaling effects on muscle-specific immune responses through controlling the recruitment and function of macrophages and T cells. Skelet. Muscle 9 (1), 20. doi:10.1186/s13395-019-0205-2
Lin J. Y., Kuo W. W., Baskaran R., Kuo C. H., Chen Y. A., Chen W. S., et al. (2020). Swimming exercise stimulates IGF1/PI3K/Akt and AMPK/SIRT1/PGC1α survival signaling to suppress apoptosis and inflammation in aging hippocampus. Aging (Albany NY) 12 (8), 6852–6864. doi:10.18632/aging.103046
Ling D., Salvaterra P. M. (2011). Brain aging and Aβ₁₋₄₂ neurotoxicity converge via deterioration in autophagy-lysosomal system: a conditional Drosophila model linking Alzheimer's neurodegeneration with aging. Acta Neuropathol. 121 (2), 183–191. doi:10.1007/s00401-010-0772-0
Little H. C., Tan S. Y., Cali F. M., Rodriguez S., Lei X., Wolfe A., et al. (2018). Multiplex quantification identifies novel exercise-regulated myokines/cytokines in plasma and in glycolytic and oxidative skeletal muscle. Mol. Cell Proteomics 17 (8), 1546–1563. doi:10.1074/mcp.RA118.000794
Lourenco M. V., Frozza R. L., de Freitas G. B., Zhang H., Kincheski G. C., Ribeiro F. C., et al. (2019). Exercise-linked FNDC5/irisin rescues synaptic plasticity and memory defects in Alzheimer's models. Nat. Med. 25 (1), 165–175. doi:10.1038/s41591-018-0275-4
Lyra E. S. N. M., Gonçalves R. A., Pascoal T. A., Lima-Filho R. A. S., Resende E. P. F., Vieira E. L. M., et al. (2021). Pro-inflammatory interleukin-6 signaling links cognitive impairments and peripheral metabolic alterations in Alzheimer's disease. Transl. Psychiatry 11 (1), 251. doi:10.1038/s41398-021-01349-z
Maass A., Düzel S., Brigadski T., Goerke M., Becke A., Sobieray U., et al. (2016). Relationships of peripheral IGF-1, VEGF and BDNF levels to exercise-related changes in memory, hippocampal perfusion and volumes in older adults. Neuroimage 131, 142–154. doi:10.1016/j.neuroimage.2015.10.084
Margolis L. M., Rivas D. A., Berrone M., Ezzyat Y., Young A. J., McClung J. P., et al. (2016). Prolonged calorie restriction downregulates skeletal muscle mtorc1 signaling independent of dietary protein intake and associated microrna expression. Front. Physiol. 7, 445. doi:10.3389/fphys.2016.00445
Marlatt M. W., Potter M. C., Lucassen P. J., van Praag H. (2012). Running throughout middle-age improves memory function, hippocampal neurogenesis, and BDNF levels in female C57BL/6J mice. Dev. Neurobiol. 72 (6), 943–952. doi:10.1002/dneu.22009
Metaxakis A., Ploumi C., Tavernarakis N. (2018). Autophagy in age-associated neurodegeneration. Cells 7 (5), 37. doi:10.3390/cells7050037
Mielke M. M., Roberts R. O., Savica R., Cha R., Drubach D. I., Christianson T., et al. (2013). Assessing the temporal relationship between cognition and gait: slow gait predicts cognitive decline in the Mayo Clinic Study of Aging. J. Gerontol. A Biol. Sci. Med. Sci. 68 (8), 929–937. doi:10.1093/gerona/gls256
Mogi M., Harada M., Riederer P., Narabayashi H., Fujita K., Nagatsu T. (1994). Tumor necrosis factor-alpha (TNF-alpha) increases both in the brain and in the cerebrospinal fluid from parkinsonian patients. Neurosci. Lett. 165 (1-2), 208–210. doi:10.1016/0304-3940(94)90746-3
Molina-Sotomayor E., Castillo-Quezada H., Martínez-Salazar C., González-Orb M., Espinoza-Salinas A., Gonzalez-Jurado J. A. (2020). Effects of progressive resistance training on cognition and igf-1 levels in elder women who live in areas with high air pollution. Int. J. Environ. Res. Public Health 17 (17), 6203. doi:10.3390/ijerph17176203
Momenzadeh S., Jami M. S., Jalalvand A., Esfarjani F., Shahabi S., Zamani S. (2022). Irisin, a mediator of muscle crosstalk with other organs: from metabolism regulation to protective and regenerative effects. Curr. Protein Pept. Sci. 23 (2), 89–104. doi:10.2174/1389203723666220217141918
Montero-Odasso M., Speechley M., Muir-Hunter S. W., Pieruccini-Faria F., Sarquis-Adamson Y., Hachinski V., et al. (2020). Dual decline in gait speed and cognition is associated with future dementia: evidence for a phenotype. Age Ageing 49 (6), 995–1002. doi:10.1093/ageing/afaa106
Moon H. Y., Becke A., Berron D., Becker B., Sah N., Benoni G., et al. (2016a). Running-induced systemic cathepsin b secretion is associated with memory function. Cell Metab. 24 (2), 332–340. doi:10.1016/j.cmet.2016.05.025
Moon J. H., Moon J. H., Kim K. M., Choi S. H., Lim S., Park K. S., et al. (2016b). Sarcopenia as a predictor of future cognitive impairment in older adults. J. Nutr. Health Aging 20 (5), 496–502. doi:10.1007/s12603-015-0613-x
Morena F., Argentati C., Trotta R., Crispoltoni L., Stabile A., Pistilli A., et al. (2017). A comparison of lysosomal enzymes expression levels in peripheral blood of mild- and severe-alzheimer's disease and mci patients: implications for regenerative medicine approaches. Int. J. Mol. Sci. 18 (8), 1806. doi:10.3390/ijms18081806
Morishita S., Tsubaki A., Nakamura M., Nashimoto S., Fu J. B., Onishi H. (2019). Rating of perceived exertion on resistance training in elderly subjects. Expert Rev. Cardiovasc Ther. 17 (2), 135–142. doi:10.1080/14779072.2019.1561278
Morley J. E. (2017a). Hormones and sarcopenia. Curr. Pharm. Des. 23 (30), 4484–4492. doi:10.2174/1381612823666161123150032
Morley J. E. (2017b). The new geriatric giants. Clin. Geriatr. Med. 33 (3), xi–xii. doi:10.1016/j.cger.2017.05.001
Mousavi K., Jasmin B. J. (2006). BDNF is expressed in skeletal muscle satellite cells and inhibits myogenic differentiation. J. Neurosci. 26 (21), 5739–5749. doi:10.1523/jneurosci.5398-05.2006
Mouser J. G., Loprinzi P. D., Loenneke J. P. (2016). The association between physiologic testosterone levels, lean mass, and fat mass in a nationally representative sample of men in the United States. Steroids 115, 62–66. doi:10.1016/j.steroids.2016.08.009
Mueller-Steiner S., Zhou Y., Arai H., Roberson E. D., Sun B., Chen J., et al. (2006). Antiamyloidogenic and neuroprotective functions of cathepsin B: implications for Alzheimer's disease. Neuron 51 (6), 703–714. doi:10.1016/j.neuron.2006.07.027
Naudí A., Caro P., Jové M., Gómez J., Boada J., Ayala V., et al. (2007). Methionine restriction decreases endogenous oxidative molecular damage and increases mitochondrial biogenesis and uncoupling protein 4 in rat brain. Rejuvenation Res. 10 (4), 473–484. doi:10.1089/rej.2007.0538
Nindl B. C., Kraemer W. J., Marx J. O., Arciero P. J., Dohi K., Kellogg M. D., et al. (2001). Overnight responses of the circulating IGF-I system after acute, heavy-resistance exercise. J. Appl. Physiol. 90 (4), 1319–1326. doi:10.1152/jappl.2001.90.4.1319
Ogawa Y., Kaneko Y., Sato T., Shimizu S., Kanetaka H., Hanyu H. (2018). Sarcopenia and muscle functions at various stages of Alzheimer disease. Front. Neurol. 9, 710. doi:10.3389/fneur.2018.00710
Page M. J., McKenzie J. E., Bossuyt P. M., Boutron I., Hoffmann T. C., Mulrow C. D., et al. (2021). The PRISMA 2020 statement: an updated guideline for reporting systematic reviews. Bmj 372, n71. doi:10.1136/bmj.n71
Pan W., Kastin A. J., Brennan J. M. (2000). Saturable entry of leukemia inhibitory factor from blood to the central nervous system. J. Neuroimmunol. 106 (1-2), 172–180. doi:10.1016/s0165-5728(00)00241-1
Pappolla M. A., Chyan Y. J., Omar R. A., Hsiao K., Perry G., Smith M. A., et al. (1998). Evidence of oxidative stress and in vivo neurotoxicity of beta-amyloid in a transgenic mouse model of Alzheimer's disease: a chronic oxidative paradigm for testing antioxidant therapies in vivo. Am. J. Pathol. 152 (4), 871–877.
Pedersen B. K., Steensberg A., Fischer C., Keller C., Keller P., Plomgaard P., et al. (2003). Searching for the exercise factor: is IL-6 a candidate? J. Muscle Res. Cell Motil. 24 (2-3), 113–119. doi:10.1023/a:1026070911202
Peng T. C., Chen W. L., Wu L. W., Chang Y. W., Kao T. W. (2020). Sarcopenia and cognitive impairment: a systematic review and meta-analysis. Clin. Nutr. 39 (9), 2695–2701. doi:10.1016/j.clnu.2019.12.014
Petersen A. M., Pedersen B. K. (2005). The anti-inflammatory effect of exercise. J. Appl. Physiol. 98 (4), 1154–1162. doi:10.1152/japplphysiol.00164.2004
Popp J., Wolfsgruber S., Heuser I., Peters O., Hüll M., Schröder J., et al. (2015). Cerebrospinal fluid cortisol and clinical disease progression in MCI and dementia of Alzheimer's type. Neurobiol. Aging 36 (2), 601–607. doi:10.1016/j.neurobiolaging.2014.10.031
Prince M., Wimo A., Guerchet M., Ali G. C., Prina M. (2015). “World alzheimer report 2015. the global impact of dementia,” in An analysis of prevalence, incidence, cost and trends.
Qiu Q., Zhou X., Wu L., Zhang Y., Yu Z., Wang M., et al. (2021). Serum cortisol is associated with cerebral small vessel disease-related brain changes and cognitive impairment. Front. Aging Neurosci. 13, 809684. doi:10.3389/fnagi.2021.809684
Quintanilla R. A., Orellana D. I., González-Billault C., Maccioni R. B. (2004). Interleukin-6 induces Alzheimer-type phosphorylation of tau protein by deregulating the cdk5/p35 pathway. Exp. Cell Res. 295 (1), 245–257. doi:10.1016/j.yexcr.2004.01.002
Radak Z., Suzuki K., Higuchi M., Balogh L., Boldogh I., Koltai E. (2016). Physical exercise, reactive oxygen species and neuroprotection. Free Radic. Biol. Med. 98, 187–196. doi:10.1016/j.freeradbiomed.2016.01.024
Rasmussen P., Brassard P., Adser H., Pedersen M. V., Leick L., Hart E., et al. (2009). Evidence for a release of brain-derived neurotrophic factor from the brain during exercise. Exp. Physiol. 94 (10), 1062–1069. doi:10.1113/expphysiol.2009.048512
Ribeiro D., Petrigna L., Pereira F. C., Muscella A., Bianco A., Tavares P. (2021). The impact of physical exercise on the circulating levels of bdnf and nt 4/5: a review. Int. J. Mol. Sci. 22 (16), 8814. doi:10.3390/ijms22168814
Roelfsema F., van Heemst D., Iranmanesh A., Takahashi P., Yang R., Veldhuis J. D. (2017). Impact of age, sex and body mass index on cortisol secretion in 143 healthy adults. Endocr. Connect. 6 (7), 500–509. doi:10.1530/ec-17-0160
Rommel C., Bodine S. C., Clarke B. A., Rossman R., Nunez L., Stitt T. N., et al. (2001). Mediation of IGF-1-induced skeletal myotube hypertrophy by PI(3)K/Akt/mTOR and PI(3)K/Akt/GSK3 pathways. Nat. Cell Biol. 3 (11), 1009–1013. doi:10.1038/ncb1101-1009
Rugarli E. I., Langer T. (2012). Mitochondrial quality control: a matter of life and death for neurons. Embo J. 31 (6), 1336–1349. doi:10.1038/emboj.2012.38
Safdar A., Saleem A., Tarnopolsky M. A. (2016). The potential of endurance exercise-derived exosomes to treat metabolic diseases. Nat. Rev. Endocrinol. 12 (9), 504–517. doi:10.1038/nrendo.2016.76
Sato A. Y., Richardson D., Cregor M., Davis H. M., Au E. D., McAndrews K., et al. (2017). Glucocorticoids induce bone and muscle atrophy by tissue-specific mechanisms upstream of e3 ubiquitin ligases. Endocrinology 158 (3), 664–677. doi:10.1210/en.2016-1779
Sato E., Mori T., Mishima E., Suzuki A., Sugawara S., Kurasawa N., et al. (2016). Metabolic alterations by indoxyl sulfate in skeletal muscle induce uremic sarcopenia in chronic kidney disease. Sci. Rep. 6, 36618. doi:10.1038/srep36618
Schakman O., Kalista S., Barbé C., Loumaye A., Thissen J. P. (2013). Glucocorticoid-induced skeletal muscle atrophy. Int. J. Biochem. Cell Biol. 45 (10), 2163–2172. doi:10.1016/j.biocel.2013.05.036
Schmidt D., Kwetkat A., Gogol M. (2011). Chronic inflammation and biomarkers. Is ageing an expression of chronic inflammation? Z Gerontol. Geriatr. 44 (3), 153–157. doi:10.1007/s00391-011-0198-x
Schulze P. C., Fang J., Kassik K. A., Gannon J., Cupesi M., MacGillivray C., et al. (2005). Transgenic overexpression of locally acting insulin-like growth factor-1 inhibits ubiquitin-mediated muscle atrophy in chronic left-ventricular dysfunction. Circ. Res. 97 (5), 418–426. doi:10.1161/01.RES.0000179580.72375.c2
Serra C., Palacios D., Mozzetta C., Forcales S. V., Morantte I., Ripani M., et al. (2007). Functional interdependence at the chromatin level between the MKK6/p38 and IGF1/PI3K/AKT pathways during muscle differentiation. Mol. Cell 28 (2), 200–213. doi:10.1016/j.molcel.2007.08.021
Sheng R., Zhang L. S., Han R., Liu X. Q., Gao B., Qin Z. H. (2010). Autophagy activation is associated with neuroprotection in a rat model of focal cerebral ischemic preconditioning. Autophagy 6 (4), 482–494. doi:10.4161/auto.6.4.11737
Siteneski A., Cunha M. P., Lieberknecht V., Pazini F. L., Gruhn K., Brocardo P. S., et al. (2018). Central irisin administration affords antidepressant-like effect and modulates neuroplasticity-related genes in the hippocampus and prefrontal cortex of mice. Prog. Neuropsychopharmacol. Biol. Psychiatry 84 (Pt A), 294–303. doi:10.1016/j.pnpbp.2018.03.004
Soilu-Hänninen M., Broberg E., Röyttä M., Mattila P., Rinne J., Hukkanen V. (2010). Expression of LIF and LIF receptor beta in Alzheimer's and Parkinson's diseases. Acta Neurol. Scand. 121 (1), 44–50. doi:10.1111/j.1600-0404.2009.01179.x
Sonntag W. E., Deak F., Ashpole N., Toth P., Csiszar A., Freeman W., et al. (2013). Insulin-like growth factor-1 in CNS and cerebrovascular aging. Front. Aging Neurosci. 5, 27. doi:10.3389/fnagi.2013.00027
Soontornniyomkij V., Risbrough V. B., Young J. W., Soontornniyomkij B., Jeste D. V., Achim C. L. (2012). Increased hippocampal accumulation of autophagosomes predicts short-term recognition memory impairment in aged mice. Age (Dordr) 34 (2), 305–316. doi:10.1007/s11357-011-9234-4
Spangenburg E. E., Booth F. W. (2006). Leukemia inhibitory factor restores the hypertrophic response to increased loading in the LIF(-/-) mouse. Cytokine 34 (3-4), 125–130. doi:10.1016/j.cyto.2006.05.001
Sugimoto T., Ono R., Murata S., Saji N., Matsui Y., Niida S., et al. (2016). Prevalence and associated factors of sarcopenia in elderly subjects with amnestic mild cognitive impairment or Alzheimer disease. Curr. Alzheimer Res. 13 (6), 718–726. doi:10.2174/1567205013666160211124828
Takano H., Morita T., Iida H., Asada K., Kato M., Uno K., et al. (2005). Hemodynamic and hormonal responses to a short-term low-intensity resistance exercise with the reduction of muscle blood flow. Eur. J. Appl. Physiol. 95 (1), 65–73. doi:10.1007/s00421-005-1389-1
Tang H., Inoki K., Brooks S. V., Okazawa H., Lee M., Wang J., et al. (2019). mTORC1 underlies age-related muscle fiber damage and loss by inducing oxidative stress and catabolism. Aging Cell 18 (3), e12943. doi:10.1111/acel.12943
Thoma A., Lightfoot A. P. (2018). NF-kB and inflammatory cytokine signalling: role in skeletal muscle atrophy. Adv. Exp. Med. Biol. 1088, 267–279. doi:10.1007/978-981-13-1435-3_12
Tsai C. L., Ukropec J., Ukropcová B., Pai M. C. (2018a). An acute bout of aerobic or strength exercise specifically modifies circulating exerkine levels and neurocognitive functions in elderly individuals with mild cognitive impairment. Neuroimage Clin. 17, 272–284. doi:10.1016/j.nicl.2017.10.028
Tsai S. F., Ku N. W., Wang T. F., Yang Y. H., Shih Y. H., Wu S. Y., et al. (2018b). Long-term moderate exercise rescues age-related decline in hippocampal neuronal complexity and memory. Gerontology 64 (6), 551–561. doi:10.1159/000488589
Tsujinaka T., Ebisui C., Fujita J., Kishibuchi M., Morimoto T., Ogawa A., et al. (1995). Muscle undergoes atrophy in association with increase of lysosomal cathepsin activity in interleukin-6 transgenic mouse. Biochem. Biophys. Res. Commun. 207 (1), 168–174. doi:10.1006/bbrc.1995.1168
Tsujinaka T., Fujita J., Ebisui C., Yano M., Kominami E., Suzuki K., et al. (1996). Interleukin 6 receptor antibody inhibits muscle atrophy and modulates proteolytic systems in interleukin 6 transgenic mice. J. Clin. Invest 97 (1), 244–249. doi:10.1172/jci118398
Tyndall A. V., Clark C. M., Anderson T. J., Hogan D. B., Hill M. D., Longman R. S., et al. (2018). Protective effects of exercise on cognition and brain health in older adults. Exerc Sport Sci. Rev. 46 (4), 215–223. doi:10.1249/jes.0000000000000161
Urso M. L., Fiatarone Singh M. A., Ding W., Evans W. J., Cosmas A. C., Manfredi T. G. (2005). Exercise training effects on skeletal muscle plasticity and IGF-1 receptors in frail elders. Age (Dordr) 27 (2), 117–125. doi:10.1007/s11357-005-1629-7
Vanderlocht J., Hellings N., Hendriks J. J., Vandenabeele F., Moreels M., Buntinx M., et al. (2006). Leukemia inhibitory factor is produced by myelin-reactive T cells from multiple sclerosis patients and protects against tumor necrosis factor-alpha-induced oligodendrocyte apoptosis. J. Neurosci. Res. 83 (5), 763–774. doi:10.1002/jnr.20781
Verghese J., Wang C., Lipton R. B., Holtzer R., Xue X. (2007). Quantitative gait dysfunction and risk of cognitive decline and dementia. J. Neurol. Neurosurg. Psychiatry 78 (9), 929–935. doi:10.1136/jnnp.2006.106914
Vilela T. C., Muller A. P., Damiani A. P., Macan T. P., da Silva S., Canteiro P. B., et al. (2017). Strength and aerobic exercises improve spatial memory in aging rats through stimulating distinct neuroplasticity mechanisms. Mol. Neurobiol. 54 (10), 7928–7937. doi:10.1007/s12035-016-0272-x
Vints W. A. J., Šeikinaitė J., Gökçe E., Kušleikienė S., Šarkinaite M., Valatkeviciene K., et al. (2024). Resistance exercise effects on hippocampus subfield volumes and biomarkers of neuroplasticity and neuroinflammation in older adults with low and high risk of mild cognitive impairment: a randomized controlled trial. Geroscience 46 (4), 3971–3991. doi:10.1007/s11357-024-01110-6
Westwood A. J., Beiser A., Decarli C., Harris T. B., Chen T. C., He X. M., et al. (2014). Insulin-like growth factor-1 and risk of Alzheimer dementia and brain atrophy. Neurology 82 (18), 1613–1619. doi:10.1212/wnl.0000000000000382
Winston C. N., Goetzl E. J., Baker L. D., Vitiello M. V., Rissman R. A. (2018). Growth hormone-releasing hormone modulation of neuronal exosome biomarkers in mild cognitive impairment. J. Alzheimers Dis. 66 (3), 971–981. doi:10.3233/jad-180302
Witham M. D., Chawner M., Biase S., Offord N., Todd O., Clegg A., et al. (2020). Content of exercise programmes targeting older people with sarcopenia or frailty - findings from a UK survey. J. Frailty Sarcopenia Falls 5 (1), 17–23. doi:10.22540/jfsf-05-017
Wrann C. D., White J. P., Salogiannnis J., Laznik-Bogoslavski D., Wu J., Ma D., et al. (2013). Exercise induces hippocampal BDNF through a PGC-1α/FNDC5 pathway. Cell Metab. 18 (5), 649–659. doi:10.1016/j.cmet.2013.09.008
Wu J., Song X., Chen G. C., Neelakantan N., van Dam R. M., Feng L., et al. (2019). Dietary pattern in midlife and cognitive impairment in late life: a prospective study in Chinese adults. Am. J. Clin. Nutr. 110 (4), 912–920. doi:10.1093/ajcn/nqz150
Yaffe K., Fiocco A. J., Lindquist K., Vittinghoff E., Simonsick E. M., Newman A. B., et al. (2009). Predictors of maintaining cognitive function in older adults: the Health ABC study. Neurology 72 (23), 2029–2035. doi:10.1212/WNL.0b013e3181a92c36
Yakabe M., Ogawa S., Ota H., Iijima K., Eto M., Ouchi Y., et al. (2018). Inhibition of interleukin-6 decreases atrogene expression and ameliorates tail suspension-induced skeletal muscle atrophy. PLoS One 13 (1), e0191318. doi:10.1371/journal.pone.0191318
Yang A., Lv Q., Chen F., Wang Y., Liu Y., Shi W., et al. (2020). The effect of vitamin D on sarcopenia depends on the level of physical activity in older adults. J. Cachexia Sarcopenia Muscle 11 (3), 678–689. doi:10.1002/jcsm.12545
Yarrow J. F., White L. J., McCoy S. C., Borst S. E. (2010). Training augments resistance exercise induced elevation of circulating brain derived neurotrophic factor (BDNF). Neurosci. Lett. 479 (2), 161–165. doi:10.1016/j.neulet.2010.05.058
Yen C. H., Chang P. S., Chang Y. H., Lin P. T. (2022). Identification of coenzyme q10 and skeletal muscle protein biomarkers as potential factors to assist in the diagnosis of sarcopenia. Antioxidants (Basel) 11 (4), 725. doi:10.3390/antiox11040725
Yu C. E., Seltman H., Peskind E. R., Galloway N., Zhou P. X., Rosenthal E., et al. (2007). Comprehensive analysis of APOE and selected proximate markers for late-onset Alzheimer's disease: patterns of linkage disequilibrium and disease/marker association. Genomics 89 (6), 655–665. doi:10.1016/j.ygeno.2007.02.002
Zappa Villar M. F., López Hanotte J., Crespo R., Pardo J., Reggiani P. C. (2021). Insulin-like growth factor 1 gene transfer for sporadic Alzheimer's disease: new evidence for trophic factor mediated hippocampal neuronal and synaptic recovery-based behavior improvement. Hippocampus 31 (10), 1137–1153. doi:10.1002/hipo.23379
Zeng J., Zhang X., Wang J., Cheng X., Zhang Y., Zhou W. (2020). Comparison of donepezil, memantine, melatonin, and liuwei dihuang decoction on behavioral and immune endocrine responses of aged senescence-accelerated mouse resistant 1 mice. Front. Pharmacol. 11, 350. doi:10.3389/fphar.2020.00350
Keywords: exercise, musculoskeletal aging, mild cognitive impairment, Alzheimer’s disease, myokines, muscle-brain loop
Citation: Gao X, Chen Y and Cheng P (2024) Unlocking the potential of exercise: harnessing myokines to delay musculoskeletal aging and improve cognitive health. Front. Physiol. 15:1338875. doi: 10.3389/fphys.2024.1338875
Received: 15 November 2023; Accepted: 20 August 2024;
Published: 02 September 2024.
Edited by:
Diego A. Bonilla, Dynamical Business and Science Society - DBSS International SAS, ColombiaCopyright © 2024 Gao, Chen and Cheng. This is an open-access article distributed under the terms of the Creative Commons Attribution License (CC BY). The use, distribution or reproduction in other forums is permitted, provided the original author(s) and the copyright owner(s) are credited and that the original publication in this journal is cited, in accordance with accepted academic practice. No use, distribution or reproduction is permitted which does not comply with these terms.
*Correspondence: Peng Cheng, Y2hlbmdwZW5nMTk5MjEwMDlAMTI2LmNvbQ==