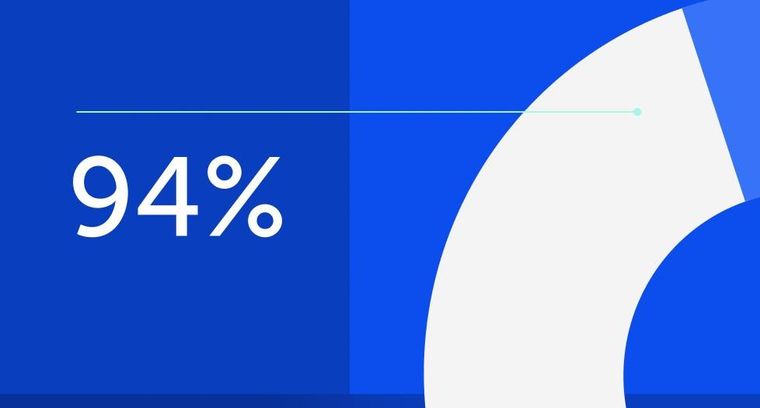
94% of researchers rate our articles as excellent or good
Learn more about the work of our research integrity team to safeguard the quality of each article we publish.
Find out more
REVIEW article
Front. Physiol., 20 March 2024
Sec. Vascular Physiology
Volume 15 - 2024 | https://doi.org/10.3389/fphys.2024.1294369
This article is part of the Research TopicNew Insights on Vascular and Metabolic Diabetic ComplicationsView all 9 articles
The significant morbidity and premature mortality of type 2 diabetes mellitus (T2DM) is largely associated with its cardiovascular consequences. Focus has long been on the arterial atheromatosis of DM giving rise to early stroke and myocardial infarctions, whereas less attention has been given to its non-ischemic cardiovascular consequences. Irrespective of ischemic changes, T2DM is associated with heart failure (HF) most commonly with preserved ejection fraction (HFpEF). Largely due to increasing population ages, hypertension, obesity and T2DM, HFpEF is becoming the most prevalent form of heart failure. Unfortunately, randomized controlled trials of HFpEF have largely been futile, and it now seems logical to address the important different phenotypes of HFpEF to understand their underlying pathophysiology. In the early phases, HFpEF is associated with a significantly impaired ability to increase cardiac output with exercise. The lowered cardiac output with exercise results from both cardiac and peripheral causes. T2DM is associated with left ventricular (LV) diastolic dysfunction based on LV hypertrophy with myocardial disperse fibrosis and significantly impaired ability for myocardial blood flow increments with exercise. T2DM is also associated with impaired ability for skeletal muscle vasodilation during exercise, and as is the case in the myocardium, such changes may be related to vascular rarefaction. The present review discusses the underlying phenotypical changes of the heart and peripheral vascular system and their importance for an adequate increase in cardiac output. Since many of the described cardiovascular changes with T2DM must be considered difficult to change if fully developed, it is suggested that patients with T2DM are early evaluated with respect to their cardiovascular compromise.
The significant morbidity and premature mortality of type 2 diabetes mellitus (T2DM) is largely associated with its cardiovascular consequences. Focus has long been on the arterial atheromatosis of DM giving rise to early stroke and myocardial infarctions, whereas less attention has been given to its non-ischemic cardiovascular consequences. In 1954, the Danish endocrinologist Knud Lundbæk was the first to document arterial wall changes associated with DM per se, and in 1969 he suggested that DM may be associated with heart disease even if un-accompanied by coronary atheromatosis. In the early 1970′s, studies of patients (pts.) with DM, who had died from heart failure (HF) revealed an association with vast islands of myocardial fibrosis despite normal coronary arteries. These findings proved Lundbæk right and confirmed the existence of a non-ischemic “diabetic cardiomyopathy” (Rubler et al., 1972). While DM may be associated with dilated non-ischemic cardiomyopathy, i.e., HF with reduced ejection fraction (HFrEF), T2DM in general gives rise to HF with preserved ejection fraction (HFpEF; Lav Madsen, 2022). Secondary to increasing age and high prevalence of obesity, hypertension, and T2DM, HFpEF is now the dominant form of HF worldwide, and the prevalence is expected to rise (Borlaug et al., 2023a). Years of randomized controlled studies (RCTs) of HFpEF treatment, with pts. stratified mainly on echo-cardiographically determined cardiac diastolic movement patterns, have been largely futile (Borlaug et al., 2023a), and it now seems logical to turn to the specific phenotypes of HFpEF, i.e., if HFpEF-symptoms are based on, for example, amyloidosis or diabetic cardiomyopathy. Weight-loss through glucagon like peptide (GLP) 1 receptor analogues will improve exercise capacity and lower natriuretic peptide levels in patients with obesity (Borlaug et al., 2023b; Kosiborod et al., 2023), but besides this no treatments for frank HFpEF exists except for limited symptom alleviation with sodium-glucose cotransporter-2 inhibitors (SGLT2i) and (possibly) spironolactone (Borlaug et al., 2023a). Medicinal regulatory authorities increasingly accept that new medication for HFpEF can now be approved based on symptom-improvement, since in pts. with HFpEF dyspnea and impaired exercise capacity can be so devastating that pts. favor improved life-quality as much as prolonged longevity. The scarcity of effective therapies for HFpEF emphasizes the importance of identifying the underlying phenotypes, and distinct pathophysiological mechanisms, if meaningful interventions to test in RCTs are to be found. It is now recognized that once frank HF is diagnosed in T2DM, the phenotypical changes leading to HF have developed over years and will be challenging to reverse. Consequently, there is growing interest in studying early stages of HFpEF, referred to as “pre-HFpEF” (Gori et al., 2021). In pts. with T2DM, this may mean already at the time diagnosis of T2DM before overt and irreversible exercise intolerance occurs.
In this review, cardiovascular changes with T2DM, among the most prevalent structural reason for HFpEF, both cardiac and peripheral changes are reviewed with the aim of addressing the major cardio-physiological systems implicated in the ability to provide for a high enough cardiac output (CO) to honor the whole-body oxygen uptake (VO2) needed for an active life without overt breathlessness. Changes with T2DM are compared with findings in the well-trained (that will be used to describe the normal biological changes seen with a systematically increased VO2 demand), and findings from pts. with obesity and hypertension are included to the extend they shed light on findings in pts. with T2DM, since obesity, hypertension, and T2DM often go hand-in-hand (hence the sometimes-used overarching terms “diabesity” and “metabolic syndrome”).
The syndrome of HFpEF is an overarching heterogenous “umbrella” term, describing exercise incapacity, overt dyspnea, and pulmonary congestion in pts. with a seemingly normal heart function. Recent reviews have highlighted major gaps in our knowledge of its causes, but reviews often remain “cardio-centric,” with little emphasis on peripheral cardiovascular causes. In T2DM, the hypertrophic “stiff” left ventricle (LV) with increased wall thickness (Mohan et al., 2015) and the associated impaired filling and increased end-diastolic pressure remains a well-documented cause of HFpEF (“diastolic dysfunction”; Abudiab et al., 2013; Borlaug et al., 2023a), but several complementary pathophysiologic mechanisms exist including abnormal ventricular-arterial coupling with equally “stiff” peripheral circulations have been documented (Shibata et al., 2011; Nesti et al., 2020; Kyhl et al., 2021). Also, integrative cardiovascular studies have documented detrimental changes to the peripheral circulation with DM, but such changes remain to be incorporated into clinical perspectives.
Numerous studies have documented lower VO2max during exercise in pts. with T2DM in comparison with age-matched controls. The VO2max values are usually 20%–30% lower than in controls (Nesti et al., 2020), although the magnitude can vary depending on the control group studied. Initially, the reduced VO2max is a consequence of a sedentary lifestyle (Hamilton et al., 2007). With time and progression of T2DM, however, significant and possibly irreversible cardiovascular changes will pose their own limit to the ability to exercise, and even in well-regulated pts. with T2DM, a reduction in the efficiency with which oxygen is converted to external work has been demonstrated (reduced slope of the VO2/workload relationship during graded exercise; Regensteiner et al., 1998). Frank and Starling at the turn of the 20th Century demonstrated the heart to be “permissive” and that the main function of the heart is to forward-deliver the blood provided from the systemic veins (Frank, 1895; Frank, 1898; Patterson et al., 1914; Patterson and Starling, 1914; Starling and Visscher, 1926). In line, Guyton demonstrated that the prevailing CO results from an integration of venous return with cardiac function (Guyton et al., 1957). It is mainly when the heart cannot forward-deliver the venous blood returned to it (or can only do so with increased end-diastolic pressure) that the term HF should be applied, but long before that, pts. can have had exercise incapacity from lowered venous return by impaired skeletal muscle vasodilation.
In normal subjects, at the onset of exercise, heart rate (HR) and ventricular stroke volume (SV) increase thereby allowing CO to closely match the metabolic demand of the working skeletal muscles (Higginbotham et al., 1986; Mortensen et al., 2005). In normal subjects, CO increases from ∼5 L/min at rest to ∼15 L/min at maximal whole body exercise in young females (Wang et al., 2012) and ∼20 L/min in young males (Mortensen et al., 2008) and up to ∼25–30 L/min (Wang et al., 2012) and ∼35–40 L/min (Ekblom and Hermansen, 1968) in elite female and male athletes. The increase in HR is responsible for most of this CO augmentation, but as HR is not increased by training, the large increase in exercise CO of the well-trained is the result of a larger SV (Bada et al., 2012; Munch et al., 2014). SV rises during exercise as a result of increases in LV end-diastolic volume and, to a lesser extent, sympathetically mediated reduction in LV end-systolic volume. LV end-diastolic volume is determined by diastolic filling, which is determined by a complex interplay between HR, intrinsic myocardial relaxation, ventricular compliance, ventricular filling pressures, atrial contraction and pericardial and pulmonary constraints (Baggish and Wood, 2011). Accordingly, increased volumes of the heart chambers are now well-established hallmarks of the “athlete’s heart” (Pelliccia et al., 1996). While overall few differences are found in resting systolic and diastolic LV function among trained athletes as compared to sedentary controls (Pluim et al., 2000), it seems LV diastolic function in exercise is enhanced by prolonged systematic training (Levine, 2008).
In pts. with T2DM, the CO increase during exercise is lower than in normal age-matched subjects (Kim et al., 2015; Figure 1). Together with notably a smaller SV, but also a lower HR at comparable workloads, the increase in CO is easily attenuated by ∼20% that is also matched by a ∼20% lower maximal workload in comparison with controls (Pinto et al., 2014; Kim et al., 2015). Even without differences in LV end-systolic volumes, during sub-maximal exercise LV end-diastolic volume is low in pts. with DM in comparison with normal age-matched controls (Wilson et al., 2017; Roberts et al., 2018). Albeit not yet studied in pure T2DM populations, it is generally observed that the common finding is that a CO increase in CO with exercise is severely restricted in pts. with frank HFpEF. When compared to normal subjects, CO is blunted in relation to the obtained VO2max, with 20% lower CO-to-VO2 slope seen in pts. with HFpEF than in age-matched normal subjects (Abudiab et al., 2013). The significantly reduced VO2max in HFpEF pts. is predominantly attributable to the CO limitation, as the HFpEF pts.’ low VO2max is coupled with blunted increases in HR (Keytsman et al., 2015), SV as well as LVEF (Abudiab et al., 2013). In one study, age-matched normal subjects increased CO to 13 vs. only 9 L/min in the pts. with severe HFpEF. In frank HFpEF, the CO increase with exercise is therefore easily 50% reduced in comparison to what is normally seen in even untrained young healthy adults (Abudiab et al., 2013).
Figure 1. Cardiac output, stroke volume and heart rate in relation to systemic oxygen uptake (VO2) in patients with heart failure with preserved ejection fraction and T2DM vs. age-matched untrained and master athletes (example with representative values from various published sources). The same amount of needed daily work to–say - walk to the near-by grocery are performed by the athlete and the sedentary obese patient with T2DM in two markedly different ways: For the well-trained athlete, the systemic VO2 required for this task will only be a fraction of what she or he can maximally deliver, and will be delivered with a high stroke volume and only need for a very small increase in heart rate, probably a heart rate increase that will only encompass a lowered vagal tone on the heart, and hardly any need for further increments in heart rate from increased sympathetic drive. For the sedentary subjects and notably for patients with HFpEF and type 2 diabetes, the systemic VO2 required is much closer to sub-maximal work, and will in these be performed with only a small or negligent increase in cardiac stroke volume but a considerably higher heart rate, - a heart rate that will be much closer to the maximally attainable heart rate, especially so if chronotropic incompetence has occurred.
Abundant echocardiographic studies demonstrate that LV hypertrophy is a notable consequence of T2DM, and that this hypertrophy is an independent predictor of adverse cardiovascular outcomes including HF (Dawson et al., 2005; Gosse, 2005). The arterial hypertension seen in seven out of 10 pts. with T2DM increases afterload to the heart and hence induces hypertrophy, but while regression of LV hypertrophy with antihypertensive treatment reduces cardiovascular morbidity and mortality, insufficient regression of LV hypertrophy is often seen (Gerdts et al., 2008; de Simone et al., 2009; de Simone et al., 2013; Lønnebakken et al., 2017) and indeed LV mass increases for other reasons than explained by mere myocyte hypertrophy. Insufficient regression of LV hypertrophy is associated with a 1.7 increased hazard ratio of cardiovascular events within the next 7 years (Lønnebakken et al., 2017). The LV hypertrophy of pts. with T2DM is associated with minor signs of both systolic and diastolic impairment even at rest. The most important cardiac changes predictive of future HFpEF, are discrete signs of systolic impairment (from global longitudinal strain) and signs of diastolic dysfunction with impaired e` (early diastolic longitudinal relaxation velocity of the left ventricle) and increased E/e’ (blood velocity during early diastolic inflow to the left ventricle as compared to the e’; Jørgensen et al., 2019). With a normal E/e’ <8, frank HFpEF is associated with values > 12–14, and many pts. with T2DM will have values in-between. Patients with T2DM therefore develop small chambered thick-walled hearts that are in many aspects virtually the antithesis to athletes’ hearts with increased chamber volumes.
The information that HFpEF in T2DM relates to the heart having hypertrophied and “stiffened” with subtle signs of systolic and diastolic dysfunction does not help much for the selection of future intervention goals, if the mechanisms which underlie these alterations are not understood. It has been convincingly demonstrated in rodents, but now also demonstrated in humans from recent cardiac magnetic resonance imaging scans, that the initial hypertrophy (often inward giving rise to small LV cavities) seen in T2DM cannot be solely explained by myocyte hypertrophy from increased afterload but is also due to the expansion of the myocardial extracellular volume (ECV) from fibrosis (Sørensen et al., 2020a; Sørensen et al., 2020b; Sørensen et al., 2020c; Bojer et al., 2020; Bojer et al., 2021a; Bojer et al., 2021b; Bojer et al., 2022; Bojer et al., 2023; Lav Madsen, 2022). In pts. with T2DM, the increase in extracellular volume is the consequence of interstitially situated advanced glycation end-products cross-linking abundant collagen micro-fibrils (Russo and Frangogiannis, 2016), and hence determination of the ECV is an imaging biomarker of disperse fibrosis. The formation of advanced glycation end-products is not only seen in the myocardium but also in vascular walls (Gonzalez et al., 2018; Frangogiannis, 2021). Perivascular fibrosis provides for “tight sheets” around the myocardial arterioles; whereas the more diffuse fibrosis that develops later becomes dispersed between cardiac myocytes (Gonzalez et al., 2018; Frangogiannis, 2021; Figure 2). In coronary arteries of pts. with ischemic heart disease including pts. with T2DM, nitric oxide production switches to hydrogen peroxide production lowering flow-mediated vasodilation (Juguilon et al., 2022). Additionally, and perhaps even more important, the perivascular fibrosis that results in vascular rarefaction, and as shown from combined endomyocardial biopsy and angiography perivascular fibrosis vascular rarefaction, perivascular fibrosis is responsible for microvascular dysfunction, i.e., lowering of myocardial blood flow during stress (Dai et al., 2012). Of interest for future clinical target determination, the perivascular fibrosis is linked to expression of mRNA signaling in pathways related to both fibrosis and osteochondrosis, whereas interstitial fibrosis is mainly related to expression of mRNA signaling related to fibrosis (Thisted et al., 2021). A detailed account of the reasons for cardiac fibrosis in T2DM is beyond the scope of this review, but in short, increased fibrosis has been attributed to inflammation, oxidative stress, an increase in endothelin production (Chen et al., 2000), AMP-activated kinase and notably insulin resistance and hyperglycemia (Rekhraj et al., 2013; Szwejkowski et al., 2013; Mohan et al., 2015; Russo and Frangogiannis, 2016).
Figure 2. The three different aspects of fibrotic myocardial changes (Masson’s trichrome staining of the mouse heart demonstrating fibrosis (blue) in the healthy myocardium) not exclusively but often seen in diabetes (from Thomas and Grisanti, 2020). These changes are in patients with type 2 diabetes non-invasively determined with cardiac magnetic resonance imaging from the extracellular volume fraction (increasing with diffuse interstitial fibrosis), gadolinium late-enhancement imaging (depicting areas of non-ischaemic replacement fibrosis), and the myocardial perfusion ratio (decreasing with perivascular fibrosis).
Cardiac magnetic resonance imaging studies document that myocardial fibrosis and microvascular dysfunction also are prevalent in pts. with T2DM (Sørensen et al., 2020a; Sørensen et al., 2020b; Sørensen et al., 2020c; Bojer et al., 2020; Bojer et al., 2021a; Bojer et al., 2021b; Bojer et al., 2022; Bojer et al., 2023; Lav Madsen, 2022). Even in pre-HFpEF, pts. with T2DM have not only myocardial hypertrophy but also significantly lowered maximal myocardial blood flow during adenosine-stress (microvascular dysfunction); and widespread myocardial fibrosis with increased ECV. In some pts. even “islands of fibrosis” (“replacement fibrosis”) are associated with increased biomarkers related to HF (pro-ANP and pro-BNP; Sørensen et al., 2020a; Sørensen et al., 2020b; Sørensen et al., 2020c; Bojer et al., 2020; Bojer et al., 2021a; Bojer et al., 2021b; Bojer et al., 2022; Bojer et al., 2023; Lav Madsen, 2022; Figure 3). Whereas the normal myocardial perfusion reserve (MPR) is ∼5, i.e., myocardial blood flow can increase by a factor of 5 with adenosine-infusion, in pts. with T2DM, it is ∼3.5, and in some pts. little or no increase in perfusion is seen with stress (Sørensen et al., 2020a; Sørensen et al., 2020b; Sørensen et al., 2020c). In normal young subjects, the ECV is ∼25%, but increases to 26%–27% in middle age, only to increase up to >28% in pts. with T2DM without complications and to >29% in pts with complications and even 40% in some (Bojer et al., 2022; Lav Madsen, 2022; Figure 3). In a broad cohort, replacement fibrosis is noted in ∼10% of all pts. with T2DM, often situated in the basal inferolateral part of the LV (Bojer et al., 2020).
Figure 3. Co-variation of myocardial extracellular volume (an imaging biomarker of disperse interstitial fibrosis) and adenosine-stress induced myocardial blood flow (an imaging biomarker of perivascular fibrosis and the accompanying vascular rarefaction) in patients with type 2 diabetes (Sørensen et al., 2020c). With abundant diffuse fibrosis resulting in an extracellular volume >30%–32% also stress myocardial blood flow is significantly lowered and cannot increase much with stress.
In animal studies, diastolic dysfunction in DM largely stems from myocardial fibrosis and microvascular dysfunction with vascular rarefaction, and in diabetic animals, fibrosis impacts negatively on systolic strain parameters (Shi et al., 2022). In pts. with T2DM, we have shown that impaired MPR and ECV both negatively impacts on diastolic function at rest, among the most significant early predictors of imminent HFpEF in pts. with T2DM (Bojer et al., 2023; Bojer et al., 2024). Of interest, the different fibrosis fractions affect different aspects of diastole, as “early” (ventricular unwinding) is more affected by impaired myocardial blood flow, i.e., the perivascular fibrosis, whereas “late” (diastasis) diastolic filling of the LV is affected by an increased ECV (i.e., diffuse myocardial fibrosis; Bojer et al., 2023; Figure 4). In pts. with T2DM, an increased ECV has been associated with a reduced VO2max (Jellis et al., 2011), but no studies exist on the precise impact of the different aspects of fibrosis for the risk of developing frank HFpEF. The LV fibrosis of T2DM is associated with autonomic neuropathy, concomitant ischemic heart disease and active (but not former) smoking, implicating a role for not only further anti-lipid therapy but potentially also anti-inflammatory therapies (Bojer et al., 2022). In broader pt. cohorts, where cardiac magnetic resonance imaging scans have been performed for clinical reasons, DM and increased HbA1c are independent predictors of an increased ECV, and an increased ECV independently of myocardial scars (replacement fibrosis) adds to the risk of premature HF or death (Yang et al., 2019). The recent magnetic resonance imaging studies points to more longstanding cardiac changes in T2DM as the reason for increased cardiac “stiffness” that–based on animal studies of the reason for such changes - must be considered difficult to reverse and hence to be found and treated well before overt HFpEF ensues, and it is likely that the pts. with T2DM with the worst MPR and the most prevalent fibrosis will also be the pts. that are first affected by clinically significant HFpEF.
Figure 4. The different phases of diastole and their relation to myocardial blood flow reserve and extracellular volume (an imaging biomarker of disperse fibrosis) (from Bojer et al., 2023). In short, myocardial blood flow impairment affects early diastole (i.e., the active and adenosine triphosphate-requiring phase of diastole), whereas disperse fibrosis predominantly affects later parts of diastole (non-energy requiring).
Not much is still known on how to reverse fibrosis of the myocardium once developed, and it is reasonable to assume that it will be difficult to fully reverse. In aged and diabetic animals, agents that can chemically break pre-existing cross-linking of collagen molecules are capable of reverting indices of vascular and myocardial compliance to levels seen in younger or non-diabetic animals, and the development of advanced glycation end-product cross-link breakers (like alagebrium) have been suggested for HFpEF in these conditions, but have not yet materialized (Fujimoto et al., 2013; Toprak and Yigitaslan, 2019). In frank HFpEF, even 1 year of intense training cannot significantly improve LV compliance and volumes, arterial stiffness, exercise capacity or ventricular-arterial coupling (Fujimoto et al., 2012), and it must be assumed that exercise at best can be used to hinder development of fibrosis. In animals, SGLT2i ameliorated myocardial oxidative stress injury and histology-verified cardiac fibrosis in diabetic mice (Li et al., 2019). Bojer et al. (2022) found a tendency for treatment with SGLT2i to lower the ECV (p = 0.06), and in one magnetic resonance imaging RCT, empagliflozine significantly lowered the ECV in pts. with T2DM and ischaemic heart disease (Mason et al., 2021), but it is still not known if in humans, this also reflects a lowered fibrosis content or merely a general improvement in extracellular volume content as shown in renal failure pts. (Ohara et al., 2019). Thus, these changes may be influenced by increased systemic inflammation, and although the impact of inflammation on development of fibrosis is not yet well-established, it is of interest that in pts. with obesity (but not T2DM) and signs of HF, weight-loss by GLP1 receptor agonists also improve systemic inflammation (Borlaug et al., 2023a). Mainly follow-up studies of such changes will document if treatment with SGLT2i will significantly impact on the development of HFpEF.
As important as it is to understand how well the heart functions in T2DM, it is no less important to study the degree of balancing of heart function with venous return. Besides the important treatment objectives for T2DM, it is therefore important to also look at cardiovascular physiological parameters that will relate to exercise tolerance.
Of importance for exercise capacity, one notable circulatory change in DM is a limited circulating blood volume (Montero et al., 2019). In general, physical work acutely reduces plasma volume by activating the renin-angiotensin-aldosterone cascade with resultant renal water retention (Convertino et al., 1980; Convertino et al., 1981). With training, the erythrocyte volume also increases until plasma volume and the erythrocyte volume have increased by 8%–10%, with the hematocrit stabilizing at a level at or slightly below the level of sedentary subjects. The hypervolemia of athletes hence reflects a larger total body water volume with increased interstitial fluid available to sweat glands allowing for greater conductive heat exchange (Convertino, 1991; Ho et al., 1997) and a lower viscosity of the blood reducing the cardiac workload (El-Sayed et al., 2005). Further, a lower hematocrit is associated with more pronounced endothelial-derived vasodilatation (Madsen et al., 2006) probably by lesser scavenging of nitric oxide (Madsen et al., 2006; Hoiland et al., 2020). In pts. with DM, the regulation of intravascular volumes is altered by chronic activation of the renin-angiotensin-aldosterone system and the vasopressin axis (Montero et al., 2019) and from albumin-loss reducing the osmotic potential of plasma (Montero et al., 2019). Hypovolemia is hence prevalent in pts. with DM, irrespective of sex, age, and even of physical activity levels (Montero et al., 2019). Part of the difference in SV between trained and untrained has been suggested to be related to differences in blood volume (Krip et al., 1997; Bonne et al., 2014), and the lowered blood volume of pts. with T2DM may in part explain their small SV. Furthermore, the lowered blood volume may in obesity and T2DM be re-distributed away from the central circulation toward veins of the lower extremities (Ubels et al., 2001; Willenberg et al., 2010; Engelberger et al., 2014), of particular importance during upright exercise.
Arterial hypertension is seen in ∼70% of pts. with T2DM, and treatment with angiotensin converting enzyme inhibitors is a cornerstone of T2DM treatment (Gaede and Pedersen, 2004). A detailed account of the reasons for hypertension in T2DM is beyond the scope of this review, however, its independent impact on cardiovascular function and its relation to HFpEF in T2DM must be addressed. If endured for years, hypertension will by way of increased cardiac afterload lead to cardiac hypertrophy probably exacerbating fibrotic cardiac changes seen with T2DM. While hypertension-research commonly has focused on resting or 24-h ambulatory blood pressure, recently exercise-induced hypertension with a maximal blood pressure (usually defined as >210 mmHg in men and >190 mmHg in women) and increased blood pressure at sub-maximal exercise have been demonstrated to have independent prognostic importance (Schultz et al., 2013). Exercise hypertension is notably prevalent in pts. with T2DM (Schultz et al., 2022) and reflects baroreflex dysfunction in pts. with metabolic syndrome even without known hypertension (Dutra-Marques et al., 2021). In general, resting hypertension can be managed not only by antihypertensive medication but also by aerobic exercise (Cornelissen and Fagard, 2005; Börjesson et al., 2016). The mechanisms underlying the effect of endurance training on resting blood pressure remain undisclosed, but are likely to include vascular remodeling (Thijssen et al., 2012); changes in the renin-angiotensin system (Hsueh and Wyne, 2011); reduced sympathetic nervous activity; and enhanced function of the nitric oxide and the prostanoid systems (Nyberg et al., 2012a; Nyberg et al., 2012b; Gunnarsson et al., 2020a; Gunnarsson et al., 2020b). The effect of training on muscle sympathetic nervous system output could be related to alternations in both central command and the pressor response originating from the contracting skeletal muscle (Mitchell et al., 1983). The vasoconstrictive effect of sympathetic activity can also be reduced in the trained muscle with a period of exercise training, via reduced alpha-adrenergic receptor sensitivity to noradrenalin (Mortensen et al., 2014). Both endurance and strength training can lower resting and exercise-induced blood pressure (Saco-Ledo et al., 2022; Correia et al., 2023). Exercise training leads to a reduction in blood pressure and sympathetic drive during acute submaximal exercise performed at the same absolute intensity when muscles of the lower limb are recruited (Cousineau et al., 1977; Winder et al., 1978; Fisher and White, 1999; Ray, 1999; Rush and Aultman, 2008; Mortensen et al., 2013). Correct antihypertensive medication of ambulatory hypertension is well-established, but it is still debated which medication best relieves exercise hypertension (Chant et al., 2018).
Conduit and resistance arteries allow for transport of blood from the heart to the peripheral organs and the diameter and elastic properties of these arteries is dimensioned so as not to limit the blood flowing from the heart, and the proximal conduit arteries exert an important pulse smoothening role (Windkessel function, please see below). The functional consequence of an increase in arterial diameter is reduced resistance during high levels of perfusion, as changes in wall thickness and wall composition have implications for vascular compliance. It is the changes in hemodynamic forces, including shear stress and transmural pressure, that lead to remodeling of arteries and arterioles, even in the heart (Aalkjaer et al., 1987; Brown, 2003). While static exercise training increases the wall thickness of conductance arteries, probably secondary to the often-significant increments in blood pressure (Scharhag et al., 2002), endurance-trained individuals have more compliant arteries (Green et al., 2017) with reduced arterial wall thickness and increased lumen diameter (Mulvany et al., 1978; Gunduz et al., 2011). Whereas heavy resistance training may decrease arterial compliance and hence the Windkessel function, the functional consequences of endurance training are accordingly the opposite (Mohiaddin et al., 1989; Cameron and Dart, 1994; Kingwell et al., 1997).
In pts. with metabolic syndrome and notably longer standing T2DM, conductance arteries including the aorta stiffen partly due to atherosclerosis, partly to media sclerosis. DM is associated not only with more narrow coronary arteries, but also with stiffening of the peripheral conductance arteries with notably smaller diameters (Frangogiannis, 2021). A more chronic increase in blood pressure likely has a greater impact on arterial wall thickness than the transient increases that occur during exercise (Gunduz et al., 2011). In media sclerosis, collagen elasticity is lost with a consequent reduction in arterial compliance. In advanced stages, large calcifications may induce secondary changes in the intima such as subendothelial hyperplasia characterized by an increase in cellularity (e.g., myofibroblasts, fibroblasts, fibrocytes) and ulcerations characterized by infiltrations of the intima or even protrusions into the lumen (Lanzer et al., 2021); (Figure 5). Such decreases in conductance including coronary arteries are associated with media sclerosis and periarterial (and periarteriolar) fibrosis with upregulation of mRNA related to not only fibrotic but also osteochondrotic pathways (Thisted et al., 2021). Media vascular wall myocytes can be differentiated into fibroblasts and thereby increase fibrosis formation and calcification (Lanzer et al., 2021). In DM, deposit of advanced glycemic end-products may also add to stiffening of conductance arteries (Fishman et al., 2018). While specific treatment modalities including statins are well-proven to improve atheromatosis, little is known about how to improve media sclerosis (Lanzer et al., 2021). Calcium-phosphate overload is important in chronic kidney disease, but in disorders with preserved systemic calcium-phosphate homeostasis, as is the case in DM, no significant specific therapy targets have been established (Lanzer et al., 2021).
Figure 5. Hematoxylin and eosin stain of tibial arteries from a patient with intimal (A) and medial (B) calcification. L denotes lumen, I denotes intima, and M denotes media (from Kim and Guzman, 2023). Patients with diabesity often suffer from intimal atheromatosis, but notably patients with T2DM also often suffer from medial calcification stiffening conductance arteries and increasing afterload to the heart.
The proximal conductance arterial tree (the ascending aorta, the aortic arch, and the proximal descending aorta) is important not only for unhindered forward-delivery of blood, but also for smoothing of the forward pressure wave (i.e., turning the pulsatile circulation into a somewhat lesser pulsatile system; “windkessel function”). Age and arterial blood pressure accounts for ∼50% of pulse-wave velocity, as the pulse-wave of the blood increases from normal values of 4–5 m/s to perhaps >9 m/s (Kyhl et al., 2021; Figure 6). Further stiffening of the proximal conductance arteries will be seen with abundant media calcification (McEniery et al., 2009) or aortic wall deposition of advanced glycation end-products (Semba et al., 2009), both abundant in diabesity. High pulse-waves will back-reflect from divisions of larger conductance arteries and eventually provide for further increments of left ventricular afterload/stress than imposed upon the heart from the mean arterial pressure itself. This results in not only ventriculo-arterial but also atrio-ventricular dysfunction (Kyhl et al., 2021; Figure 6). An increased aortic pulse-wave velocity is not simply due to a large burden of atheromas as demonstrated in a cohort of autopsy cases (Sawabe et al., 2005). From animal studies and prospective studies with multiple regression analysis, it is already well-known that arterial stiffness increases left ventricular afterload (Urschel et al., 1968; Milnor, 1975), myocardial work (Kelly et al., 1992), impairs ventricular relaxation (Hori et al., 1985; Kohno et al., 1996), and causes myocardial ischemia (Buckberg et al., 1972; Watanabe et al., 1993; Kaess et al., 2012). A non-distensible arterial tree results in left ventricular remodeling and ultimately dysfunction (Girerd et al., 1991; Saba et al., 1993; London et al., 2004; Zieman et al., 2005; Dai et al., 2015; Chirinos et al., 2019) and is associated with an increased risk of HF and overall cardiovascular-related mortality (Levy et al., 1990; Madhavan et al., 1994; Franklin et al., 1997; Domanski et al., 1999; Haider et al., 2003). Pts. with DM show stiff conductance arteries at an early age (Christoforidis et al., 2022), and is seen in pts. with metabolic syndrome before presenting with T2DM (Donley et al., 2014).
Figure 6. (A) left ventricular (LV) myocardial mass (circles, left axis) and mean arterial pressure (MAP; solid circles, right axis) to total aortic pulse wave velocity (circle) as determined from magnetic resonance imaging determined time-volume curves in 36 healthy young and 16 healthy middle-aged subjects. (B) left atrium (LA) active emptying volume to total aortic pulse wave velocity. (C) LA passive emptying volume to total aortic pulse wave velocity. (D) indexed LV peak filling rate to total aortic pulse wave velocity. Total aortic pulse wave velocity is correlated to LV peak filling rate (r = − 0.47) and both LA passive (r = − 0.66) and active emptying volumes (r = 0.47) (all p < 0.05) (from Kyhl et al., 2021). In short, it seems that in normal subjects stiff conductance arteries are associated with not only impaired ventriculo-arterial coupling but also impaired atrio-ventricular coupling.
Aerobic exercise training reduces arterial stiffness (Donley et al., 2014), and in a controlled trial of moderate vs. high-intensity exercise, pts. with T2DM both training schemes improved carotid artery media thickness, but only the high intensity training scheme was effective in improving conductance artery stiffness (Magalhaes et al., 2019). Exercise training also reduces arterial stiffness in adults with hypertension (Lopes et al., 2021). Even in elderly pts. with DM and at risk of leg ulcers, 3 months of three weekly resistance exercise training bouts may improve signs of peripheral artery stiffness (Gholami et al., 2021). SGLT2i were early shown to lower not only blood sugars but also advanced glycation end-product formation in vessels (Shen et al., 2012) and indeed lower indices of arterial stiffness (Chilton et al., 2015; Davies et al., 2017; Pfeifer et al., 2017).
To accommodate an increased demand for O2 during exercise by contracting muscle, trained individuals have a higher maximal perfusion capacity than untrained individuals, as blood flow to skeletal muscle in elite athletes can increase almost 100-fold from rest (Richardson et al., 1993; Blomstrand et al., 1997). This elevation in blood flow is achieved by an increased CO in combination with an enhancement in vascular conductance in the active muscle. Exercise hyperemia is a complex and well-regulated process that involves sympathetic vasoconstrictor signaling in combination with an integration of a large number of vasodilator systems as well as several modes of activation of these systems. To “defend blood pressure” during significant vasodilatation in working muscles, the increased sympathetic activity maintaining the increased CO also causes arteriolar constriction in relatively less active tissues (Joyner and Casey, 2015). Hence, during exercise the increased sympathetic activity causes arteriolar constriction in tissues with low metabolic demand and in the active muscle effective amounts of vasodilators have to be formed to overcome and exceed this constriction to enable sufficient oxygen delivery. In the active muscle, the increase in sympathetic vasoconstriction is overcome by local formation of a number of different vasodilators and by “functional sympatholysis”, a modulation of the vasoconstrictive effect of the sympathetic activity. Our understanding of precisely how skeletal muscle blood flow is regulated in response to changes in metabolic demand is not complete. However, fundamentally it is highly probable that there are signals that are closely coupled to a, yet to be identified, mechanism of oxygen metabolic sensing within the muscle tissue. One such potential mechanism is erythrocyte mediated vasodilation where erythrocytes release the vasodilators ATP (Ellsworth and Sprague, 2012) and nitric oxide (Singel and Stamler, 2005) in response to oxygen desaturation of the hemoglobin molecule, thus connecting the utilization of oxygen by the muscle to the magnitude of blood flow. A number of other mechanisms and compounds, not directly coupled to oxygen sensing or metabolism, have been identified but their relative importance and roles remain unclear. Out of the vasodilatory mechanisms and compounds identified, several induce their effect via sensors or receptors located on the endothelium resulting in the formation of vasodilators. Such endothelial-dependent mechanisms include flow (shear-stress)-induced vasodilatation and vasodilatation balanced by chemicals such as nitric oxide, adenosine triphoshate, adenosine, reactive oxygen species, prostaglandins, endothelial-derived hyperpolarizing factors, and Endothelin-1. Shear stress acutely increases nitric oxide formation (Rubanyi et al., 1986) and upregulates endothelial nitric oxide synthase (Woodman et al., 1999; Tuttle et al., 2001; Baum et al., 2004). Apart from endothelial cells, cellular sources of vasodilator compounds during exercise include skeletal muscle cells, and notably adenosin, ATP, prostacyclin and NO have been suggested to induce vasodilation by direct vasorelaxation of smooth muscle cells (Hellsten et al., 1998; Hellsten et al., 2012b). Of interest, exercise improves flow-mediated vasodilation not only in the exercised limbs but also in non-active limbs (Birk et al., 2012). Another mechanism involved in the control of blood flow to working muscles and which particularly serves to coordinate flow is conducted, or retrograde, vasodilation. In this process a vasoactive signal initiated either at the capillary level (Murrant and Sarelius, 2000) or at the arteriolar level (Segal and Duling, 1989; Bagher and Segal, 2011) is conveyed up and downstream via gap junctions either in the endothelium or in the smooth muscle cells. Some vasodilation may be through the myogenic response by which vessel wall myocytes respond to acute changes in transmural pressure (de Witt et al., 1998). So far it is known that all of these can mechanisms induce vasodilation of importance during exercise (Casey et al., 2013), but none of these seem to be obligatory since a great degree of redundancy exist in that other vasodilator systems take over if one system is pharmacologically inhibited.
In pts. with hypertension and DM, the blood flow increment during submaximal exercise is often reduced (Kivelä et al., 2006; Thaning et al., 2010; 2011; Sacre et al., 2015; Olver and Laughlin, 2016). Lower arm hyperemia induced by submaximal and maximal hand-grip exercise is reduced in pts. with DM, who also have microvascular complications (Womack et al., 2009). One frequently observed outcome of endurance training, that likely affects the magnitude of blood flow to the muscle during submaximal exercise, is an increased arterio-venous oxygen extraction, which in part occurs as a result of an increased capillarization (Mortensen et al., 2017) and thereby improved oxygen diffusion capacity but which also is dependent on mitochondrial capacity. In a study by Nyberg et al. (2012b), it was found that blood flow during submaximal exercise performed at the same absolute workload was reduced after training in normotensive subjects, indicating an improved oxygen extraction, whereas blood flow was unaltered in hypertensive subjects after 8 weeks of endurance training. The leg vasodilator response to exercise has been reported to be lower in T2DM (Thaning et al., 2010; Groen et al., 2019) although a preserved response has also been demonstrated (Thaning et al., 2011).
Flow-mediated vasodilation is significantly impaired in pts. with DM, and while it is well-proven that exercise training improves this physiological response, it seems that the improvements seen with exercise training in pts. with T2DM are smaller than in non-diabetic controls (Qiu et al., 2018). In pts. with T2DM, training improves arterial flow-mediated (endothelial dependent) and non-endothelial dependent dilatation (Schreuder et al., 2015), and training lowers the skeletal muscle amount of endothelial nitric oxide uncoupling, suggesting enhanced capacity for nitric oxide formation, both in normal subjects and in pts. with hypertension (Nyberg et al., 2012b). Exercise training, especially in the elderly, may enhance nitric oxide availability by reducing scavenging of nitric oxide by reactive oxygen species (Taddei et al., 2000; Franzoni et al., 2005; Kirby et al., 2009; Crecelius et al., 2010; Nyberg et al., 2012b; Nyberg et al., 2014). Thus, training will improve nitric oxide availability partly by increased formation of nitric oxide itself, partly by reduced scavenging of nitric oxide, and it seems training is more important in subjects where it is already impaired. Prostaglandins may contribute to exercise-related vasodilation, and training in pts. with hypertension enhances the increase in muscle interstitial prostacyclin (Hellsten et al., 2012a). Other important vasodilator systems include ATP and adenosine as individuals with T2DM have been reported to have lower plasma ATP concentrations during exercise that occurs in parallel with a lower blood flow and the inhibition of adenosine receptors reduces exercising muscle blood flow by 15%–20% at least in healthy individuals (Radegran and Calbet, 2001; Mortensen et al., 2009). Lifelong physical activity opposes an age-related increase in skeletal muscle and plasma levels of the potent vasoconstrictor endothelin-1, and 8 weeks of training normalizes plasma endothelin-1 levels in individuals with essential hypertension (Nyberg et al., 2013). Furthermore, endothelin-1, is lowered by 30% by training in pts. with hypertension, where training improves not only the levels of endothelin-1 but concomitantly also carotid artery compliance (Maeda et al., 2009). Among vasoconstrictors, angiotensin II is well-known to be associated with vascular dysfunction and has been shown to decrease in response to exercise training (Zucker et al., 2015).
Capillary density in muscle is important because a small microvascular network of the skeletal muscles limit the ability of an enhanced cardiac output during exercise to supply skeletal muscles with a high blood flow without a reduction in the time for gas exchange at the capillary site. A high vasodilatory capacity combined with a high skeletal muscle capillary volume are thus advantageous adaptations to training. Thus, training increases the vasodilator capacity as evidenced in athletes where a greater peak vasodilator response is seen in the dominant arm of tennis players (Sinoway et al., 1986) and a maximal vasodilator response has been described after a period of forearm training in previously untrained (Sinoway et al., 1987). Moreover, as little as 4 weeks of training can increase capillary density (Jensen et al., 2004) and when previously sedentary healthy individuals are endurance-trained for 4–8 weeks an increase in capillarization of up to 40% is observed (Hoier and Hellsten, 2014; Ingjer, 1979a; Ingjer, 1979b; Klausen et al., 1981). The physiological signals governing capillary growth are still not completely understood but generally involves mechanical stimuli (Hellsten et al., 2008) with both shear stress (Langille and O´Donnell, 1986; Pipp et al., 2004; Tuttle et al., 2001) and passive stretch of the muscle tipping the weight from anti-angiogenic compounds towards pro-angiogenic compounds (Hudlicka and Egginton, 1992). Shear stress is known to be a particularly important physical factor for both arterial and capillary growth where it induces its effect by activating and enhancing the expression of several angiogenic factors, including the central growth factor vascular endothelial growth factor (VEGF), (Hudlicka and Egginton, 1992; Lloyd et al., 2005). Hypoxia, via hypoxia inducible factor 1-alpha is also known to promote capillary growth, in part via transcription of VEGF (Gustafsson et al., 2007). There is a clear correlation between training status and expression of many pro-angiogenic compounds, and studies show increments of these with training in sedentary individual (Gavin et al., 2007; Hoier et al., 2012; Gliemann et al., 2014) including in pts. with primary hypertension (Hansen et al., 2010). Although specifically VEGF protein levels in muscle are not commonly altered by exercise training in young subjects, individuals.
Training increases the capillary/fibre ratio also in T2D (Lithell et al., 1985), but an attenuation in the exercise-induced increase in capillarization has also been reported in type 1 DM mice in part likely due to reduced levels of proangiogenic factors (VEGF-A, VEGF-B, neuropilin-1, VEGFR-1, and VEGFR-2) and increased levels of antiangiogenic factors (thrombospondin-1 and retinoblastoma like-2) in comparison with normal mice (Kivelä et al., 2006). Thus, exercise training in pts. with DM alleviated some of these changes, but may not completely restore them. T2DM alters capillary hemodynamics, causes capillary rarefaction in skeletal muscle as is seen in the myocardium, and alters endothelial and vascular smooth muscle cell phenotype, resulting in impaired vasodilatory responses (Olver and Laughlin, 2016). Training will improve the endothelial-dependent as well as the non-endothelial-dependent vasodilation in pts. with T2DM (Maiorana et al., 2001). Individuals with T2DM have lower plasma ATP concentrations during exercise and hypoxia compared with control individuals, and this occurs in parallel with lower blood flow. Moreover, individuals with T2DM have a reduced vasodilatory response to infused ATP (Groen et al., 2019). In patients with DM the skeletal muscle vasodilatory effect of purines is only 50% of what is seen in normal subjects, probably as a result of receptor insensitivity (Thaning et al., 2010).
The basement membrane surrounding capillaries in skeletal muscles varies physiologically in thickness according to age, physical fitness, and anatomical site in humans and prematurely thickens with DM (Baum and Bigler, 2016). A thickened capillary basement membrane likely poses a greater barrier for diffusion, lowers the microvascular elasticity, and impedes transcytosis of inflammatory cells of possible importance for among others wound healing in DM (Baum and Bigler, 2016). Interestingly, the basement membrane thickness of capillaries in skeletal muscle has been shown to be reduced by exercise training both in pts. with T2DM (Mortensen et al., 2019) and essential hypertension (Gliemann et al., 2015). As is seen in the myocardium, related to this, T2DM is characterized by a fibrotic extracellular matrix with increased hyaluron and integrins: this may contributs to impaired glucose uptake by skeletal muscle cells and presumably also to oxygen (Hulett et al., 2022), and fibrosis is also seen in skeletal muscles of pts. with DM (Farup et al., 2021). Probably related to the same mechanisms, with long-standing DM even infarctions probably secondary to vascular rarefaction may develop (Trujillo-Santos, 2003).
Preclinical studies and clinical trials involving the use of GLP-1 receptor agonists have shown salutary cardiovascular effects and improved cardiovascular outcomes in T2DM (Love et al., 2020) but the precise impact of GLP-1 receptor agonists and SGLT2i on skeletal and myocardial capillary density and function remain unclear. GLP-1, in addition to its well-characterized glycemic actions, however, improves endothelial function, increases muscle microvascular perfusion, and stimulates angiogenesis (Love et al., 2020). Importantly, these actions are preserved in the insulin resistant states (Love et al., 2020). Thus, treatment of insulin resistant pts. with GLP-1 receptor agonists may improve skeletal and cardiac muscle microvascular perfusion and increase muscle capillarization, leading to improved delivery of oxygen, nutrients, and hormones such as insulin to the myocytes (Love et al., 2020).
While the pathophysiology driving the emerging “HFpEF epidemic” in pts. with T2DM is heavily influenced by the documented cardiac changes of myocardial hypoperfusion and fibrosis, the peripheral vascular changes are equally important and should be considered when evaluating poor exercise capacity. Thus, T2DM impacts all major cardiovascular mechanisms well-documented to be of importance in exercise and hence for the individual´s ability to lead an active healthy life. In short, documented cardiovascular changes in pts. with T2DM collectively point to a “low-CO-with-exercise-syndrome” that with respect to cardiac and peripheral changes are the antithesis to what is seen with an active healthy lifestyle. Initially, an impaired muscle perfusion with exercise may be (partly) reversed with weight-loss and training since a number of the vasodilatory mechanisms affected seem resilient, but with long-standing T2DM more profound changes such as myocardial and skeletal muscle vascular rarefaction with perivascular fibrosis will limit exercise capacity. In addition to weight-loss by gastric by-pass or treatment with GLP-1 receptor agonists, it has long been well-documented that exercise will reverse most aspects of T2DM and should be added to the weight-loss intervention. Most important aspects of exercise tolerance can be improved by exercise and indeed by weight-loss, but once more substantial cardiac and peripheral changes, such as vascular rarefaction and fibrosis, have occurred, it will be much more difficult to reverse the exercise intolerance. GLP1 receptor agonists and SGLT2 inhibitors may not only to some degree relieve symptoms of frank HF but instituted early may even halt and possibly reverse findings of fibrosis and vascular rarefaction. To combat the epidemic of HFpEF in diabesity, screening with early identification of the underlying pathophysiological changes should be instituted early in order to enable personalized treatment of deeper phenotypical traits all documented to be of importance for difficult-to-reverse cardiovascular changes. Recent epidemiological studies show that the prevalence of T2DM continues to increase worldwide and now also increases substantially in the young (GBD Diabetes Collaborator, 2023), and hence the epidemic of HFpEF will not be confined to the elderly but will probably increase substantially also in the young with potentially a large number of active patient years to protect.
PL: Conceptualization, Data curation, Methodology, Writing–original draft. CS: Visualization, Writing–review and editing. MN: Writing–review and editing. MS: Writing–review and editing. YH: Writing–review and editing. PG: Writing–review and editing. AB: Writing–review and editing.
The author(s) declare that no financial support was received for the research, authorship, and/or publication of this article.
MN is employed at Novo Nordisk A/S. YH, PL, and AB have received funding from Novo Nordisk A/S for an entirely different study from the current.
The remaining authors declare that the research was conducted in the absence of any commercial or financial relationships that could be construed as a potential conflict of interest.
The author(s) declared that they were an editorial board member of Frontiers, at the time of submission. This had no impact on the peer review process and the final decision.
All claims expressed in this article are solely those of the authors and do not necessarily represent those of their affiliated organizations, or those of the publisher, the editors and the reviewers. Any product that may be evaluated in this article, or claim that may be made by its manufacturer, is not guaranteed or endorsed by the publisher.
Aalkjaer C., Heagerty A. M., Petersen K. K., Swales J. D., Mulvany M. J. (1987). Evidence for increased media thickness, increased neuronal amine uptake, and depressed excitation–contraction coupling in isolated resistance vessels from essential hypertensives. Circ. Res. 61, 181–186. doi:10.1161/01.res.61.2.181
Abudiab M. M., Redfied M. M., Melenovsky V., Olson T. P., Kass D. A., Johnson B. D., et al. (2013). Cardiac output response to exercise in relation to metabolic demand in heart failure with preserved ejection fraction. Eur. J. Heart. Fail 15, 776–785. doi:10.1093/eurjhf/hft026
Bada A. A., Svendsen J. H., Secher N. H., Saltin B., Mortensen S. P. (2012). Peripheral vasodilatation determines cardiac output in exercising humans: insight from atrial pacing. J. Physiol. 590, 2051–2060. doi:10.1113/jphysiol.2011.225334
Baggish A. L., Wood M. J. (2011). Athlete’s heart and cardiovascular care of the athlete: scientific and clinical update. Circ 123, 2723–2735. doi:10.1161/CIRCULATIONAHA.110.981571
Bagher P., Segal S. S. (2011). Regulation of blood flow in the microcirculation: role of conducted vasodilation. Acta Physiol. (Oxf). 202, 271–284. doi:10.1111/j.1748-1716.2010.02244.x
Baum O., Bigler M. (2016). Pericapillary basement membrane thickening in human skeletal muscles. Am. J. Physiol. Heart. Circ. Physiol. 311, 654–666. doi:10.1152/ajpheart.00048.2016
Baum O., Da Silva-Azevedo L., Willerding G., Wockel A., Planitzer G., Gossrau R., et al. (2004). Endothelial NOS is main mediator for shear stress-dependent angiogenesis in skeletal muscle after prazosin administration. Am. J. Physiol. Heart. Circ. Physiol. 287, 2300–2308. doi:10.1152/ajpheart.00065.2004
Birk G. K., Dawson E. A., Atkinson C., Haynes A., Cable N. T., Thijssen D. H., et al. (2012). Brachial artery adaptation to lower limb exercise training: role of shear stress. J. Appl. Physiol. 112, 1653–1658. doi:10.1152/japplphysiol.01489.2011
Blomstrand E., Radegran G., Saltin B. (1997). Maximum rate of oxygen uptake by human skeletal muscle in relation to maximal activities of enzymes in the Krebs cycle. J. Physiol. 501, 455–460. doi:10.1111/j.1469-7793.1997.455bn.x
Bojer A. S., Sørensen M. H., Bjerre J., Gaede P., Vejlstrup N., Madsen P. L. (2021b). Metabolic improvement with short-term, glucagon-like peptide-1 receptor agonist treatment does not improve cardiac diastolic dysfunction in patients with type 2 diabetes: a randomized, double-blind, placebo-controlled trial. Diabetes Obes. Metab. 23, 2374–2384. doi:10.1111/dom.14480
Bojer A. S., Sørensen M. H., Gæde P., Madsen P. L. (2022). Myocardial extracellular volume expansion in type 2 diabetes is associated with ischemic heart disease, autonomic neuropathy, and active smoking. Diabetes Care 45, 3032–3039. doi:10.2337/dc22-0942
Bojer A. S., Sørensen M. H., Gaede P., Myerson S., Madsen P. L. (2021a). Left ventricular diastolic function studied with magnetic resonance imaging: a systematic review of techniques and relation to established measures of diastolic function. Diagn. (Basel) 11, 1282. doi:10.3390/diagnostics11071282
Bojer A. S., Sørensen M. H., Madsen S. H., Broadbent D. A., Plein S., Gæde P., et al. (2023). The independent association of myocardial extracellular volume and myocardial blood flow with cardiac diastolic function in patients with type 2 diabetes: a prospective cross-sectional cohort study. Cardiovasc. Diabetol. 22, 78. doi:10.1186/s12933-023-01804-9
Bojer A. S., Sørensen M. H., Vejlstrup N., Goetze J. P., Gæde P., Madsen P. L. (2020). Distinct non-ischemic myocardial late gadolinium enhancement lesions in patients with type 2 diabetes. Cardiovasc. Diabetol. 19, 184. doi:10.1186/s12933-020-01160-y
Bojer A. S., Sørensen M. H., Madsen S. H., Broadbent D. A., Plein S., Gaede P., et al. (2024). Early signs of myocardial systolic dysfunction in patients with type 2 diabetes are strongly associated with myocardial microvascular dysfunction independent of myocardial fibrosis: a prospective cohort study. Diabetol. Metab. Sydr. 16 (1), 41. doi:10.1186/s13098-024-01285-0
Bonne T. C., Doucende G., Flück D., Jacobs R. A., Nordsborg N. B., Robach P., et al. (2014). Phlebotomy eliminates the maximal cardiac output response to six weeks of exercise training. Am. J. Physiol. Regul. Integr. Comp. Physiol. 306 (10), R752–R760. Epub 2014 Mar 12.PMID: 24622974. doi:10.1152/ajpregu.00028.2014
Börjesson M., Onerup A., Lundqvist S., Dahlöf B. (2016). Physical activity and exercise lower blood pressure in individuals with hypertension: narrative review of 27 RCTs. Br. J. Sports. Med. 50, 356–361. doi:10.1136/bjsports-2015-095786
Borlaug B. A., Kitzman D. W., Davies M. J., Rasmussen S., Barros E., Butler J., et al. (2023b). Semaglutide in HFpEF across obesity class and by body weight reduction: a prespecified analysis of the STEP-HFpEF trial. Nat. Med. 29, 2358–2365. doi:10.1038/s41591-023-02526-x
Borlaug B. A., Sharma K., Shah S. J., Ho J. E. (2023a). Heart failure with preserved ejection fraction: JACC scientific statement. J. Am. Coll. Cardiol. 81, 1810–1834. doi:10.1016/j.jacc.2023.01.049
Brown M. D. (2003). Exercise and coronary vascular remodelling in the healthy heart. Exp. Physiol. 88, 645–658. doi:10.1113/eph8802618
Buckberg G. D., Fixler D. E., Archie J. P., Hoffman J. I. E. (1972). Experimental subendocardial ischemia in dogs with normal coronary arteries. Circ. Res. 30, 67–81. doi:10.1161/01.res.30.1.67
Cameron J. D., Dart A. M. (1994). Exercise training increases total systemic arterial compliance in humans. Am. J. Physiol. 266, 693–701. doi:10.1152/ajpheart.1994.266.2.H693
Casey D. P., Walker B. G., Ranadive S. M., Taylor J. L., Joyner M. J. (2013). Contribution of nitric oxide in the contraction-induced rapid vasodilation in young and older adults. J. Appl. Physiol. 115, 446–455. doi:10.1152/japplphysiol.00446.2013
Chant B., Bakali M., Hinton T., Burchell A. E., Nightingale A. K., Paton J. F. R., et al. (2018). Antihypertensive treatment fails to control blood pressure during exercise. Hypertension 72, 102–109. doi:10.1161/HYPERTENSIONAHA.118.11076
Chen S., Evans T., Mukherjee K., Karmazyn M., Chakrabarti S. (2000). Diabetes-induced myocardial structural changes: role of endothelin-1 and its receptors. J. Mol. Cell. Cardiol. 32, 1621–1629. doi:10.1006/jmcc.2000.1197
Chilton R., Tikkanen I., Cannon C. P., Crowe S., Woerle H. J., Broedl U. C., et al. (2015). Effects of empagliflozin on blood pressure and markers of arterial stiffness and vascular resistance in patients with type 2 diabetes. Diabetes. Obes. Metab. 17, 1180–1193. doi:10.1111/dom.12572
Chirinos J. A., Segers P., Hughes T., Townsend R. (2019). Large-artery stiffness in health and disease: JACC State-of-the-Art Review. J. Am. Coll. Cardiol. 74, 1237–1263. doi:10.1016/j.jacc.2019.07.012
Christoforidis A., Georeli I., Dimitriadou M., Galli-Tsinopoulou A., Stabouli S. (2022). Arterial stiffness indices in children and adolescents with type 1 diabetes mellitus: a meta-analysis. Diabetes. Metab. Res. Rev. 38, e3555. doi:10.1002/dmrr.3555
Convertino V. A. (1991). Blood volume: its adaptation to endurance training. Med. Sci. Sports. Exerc. 23, 1338–1348. doi:10.1249/00005768-199112000-00004
Convertino V. A., Brock P. J., Keil L. C., Bernauer E. M., Greenleaf J. E. (1980). Exercise training-induced hypervolemia: role of plasma albumin, renin, and vasopressin. J. Appl. Physiol. Respir. Environ. Exerc. Physiol. 48, 665–669. doi:10.1152/jappl.1980.48.4.665
Convertino V. A., Keil L. C., Bernauer E. M., Greenleaf J. E. (1981). Plasma volume, osmolality, vasopressin, and renin activity during graded exercise in man. J. Appl. Physiol. Respir. Environ. Exerc. Physiol. 50, 123–128. doi:10.1152/jappl.1981.50.1.123
Cornelissen V. A., Fagard R. H. (2005). Effects of endurance training on blood pressure, blood pressure-regulating mechanisms, and cardiovascular risk factors. Hypertension 46, 667–675. doi:10.1161/01.HYP.0000184225.05629.51
Correia R. R., Veras S. V., Tebar W. R., Rufino J. C., Batista V. R. G., Teixeira G. R. (2023). Strength training for arterial hypertension treatment: a systematic review and meta-analysis of randomized clinical trials. Sci. Rep. 13, 201. doi:10.1038/s41598-022-26583-3
Cousineau D., Ferguson R. J., de Champlain J., Gauthier P., Cote P., Bourassa M. (1977). Catecholamines in coronary sinus during exercise in man before and after training. J. Appl. Physiol. Respir. Environ. Exerc. Physiol. 43, 801–806. doi:10.1152/jappl.1977.43.5.801
Crecelius A. R., Kirby B. S., Voyles W. F., Dinenno F. A. (2010). Nitric oxide, but not vasodilating prostaglandins, contributes to the improvement of exercise hyperemia via ascorbic acid in healthy older adults. Am. J. Physiol. Heart. Circ. Physiol. 299, 1633–1641. doi:10.1152/ajpheart.00614.2010
Dai X., Hummel S. L., Salazar J. B., Taffet G. E., Zieman S., Schwartz J. B. (2015). Cardiovascular physiology in the older adults. J. Geriatr. Cardiol. 12, 196–201. doi:10.11909/j.issn.1671-5411.2015.03.015
Dai Z., Aoki T., Fukumoto Y., Shimokawa H. (2012). Coronary perivascular fibrosis is associated with impairment of coronary blood flow in patients with non-ischemic heart failure. J. Cardiol. 60, 416–421. doi:10.1016/j.jjcc.2012.06.009
Davies M. J., Merton K., Vijapurkar U., Yee J., Qiu R. (2017). Efficacy and safety of canagliflozin in patients with type 2 diabetes based on history of cardiovascular disease or cardiovascular risk factors: a post hoc analysis of pooled data. Cardiovasc. Diabetol. 16, 40. doi:10.1186/s12933-017-0517-7
Dawson A., Morris A. D., Struthers A. D. (2005). The epidemiology of left ventricular hypertrophy in type 2 diabetes mellitus. Diabetologia 48, 1971–1979. doi:10.1007/s00125-005-1896-y
de Simone G., Devereux R. B., Izzo R., Girfoglio D., Lee E. T., Howard B. V., et al. (2013). Lack of reduction of left ventricular mass in treated hypertension: the Strong Heart Study. J. Am. Heart. Assoc. 2, e000144. doi:10.1161/JAHA.113.000144
de Simone G., Okin P. M., Gerdts E., Olsen M. H., Wachtell K., Hille D. A., et al. (2009). Clustered metabolic abnormalities blunt regression of hypertensive left ventricular hypertrophy: the LIFE study. Nutr. Metab. Cardiovasc. Dis. 19, 634–640. doi:10.1016/j.numecd.2008.12.012
de Witt C., Jahrbeck B., Schafer C., Bolz S. S., Pohl U. (1998). Nitric oxide opposes myogenic pressure responses predominantly in large arterioles in vivo. Hypertension 31, 787–794. doi:10.1161/01.hyp.31.3.787
Domanski M. J., Davis B. R., Pfeffer M. A., Kastantin M., Mitchell G. F. (1999). Isolated systolic hypertension: prognostic information provided by pulse pressure. Hypertension 34, 375–380. doi:10.1161/01.hyp.34.3.375
Donley D. A., Fournier S. B., Reger B. L., DeVallance E., Bonner D. A., Olfert I. M., et al. (2014). Aerobic exercise training reduces arterial stiffness in metabolic syndrome. J. Appl. Physiol. 116, 1396–1404. doi:10.1152/japplphysiol.00151.2014
Dutra-Marques A. C., Rodrigues S., Cepeda F. X., Toschi-Dias E., Rondon E., Carvalho J. C., et al. (2021). Exaggerated exercise blood pressure as a marker of baroreflex dysfunction in normotensive metabolic syndrome patients. Front. Neurosci. 9, 680195. doi:10.3389/fnins.2021.680195
Ekblom B., Hermansen L. (1968). Cardiac output in athletes. J. Appl. Physiol. 25, 619–625. doi:10.1152/jappl.1968.25.5.619
Ellsworth M. L., Sprague R. S. (2012). Regulation of blood flow distribution in skeletal muscle: role of erythrocyte-released ATP. J. Physiol. 590, 4985–4991. doi:10.1113/jphysiol.2012.233106
El-Sayed M. S., Ali N., El-Sayed A. Z. (2005). Haemorheology in exercise and training. Sports Med. 35, 649–670. doi:10.2165/00007256-200535080-00001
Engelberger R. P., Indermühle A., Baumann F., Fahrni J., Diehm N., Kucher N., et al. (2014). Diurnal changes of lower leg volume in obese and non-obese subjects. Int. J. Obes. (Lond). 38, 801–805. doi:10.1038/ijo.2013.178
Farup J., Just J., de Paoli F., Lin L., Jensen J. B., Billeskov T., et al. (2021). Human skeletal muscle CD90+ fibro-adipogenic progenitors are associated with muscle degeneration in type 2 diabetic patients. Cell. Metab. 33, 2201–2214.e11. doi:10.1016/j.cmet.2021.10.001
Fisher W. J., White M. J. (1999). Training-induced adaptations in the central command and peripheral reflex components of the pressor response to isometric exercise of the human triceps surae. J. Physiol. 520, 621–628. doi:10.1111/j.1469-7793.1999.00621.x
Fishman S. L., Sonmez H., Basman C., Singh V., Poretsky L. (2018). The role of advanced glycation end-products in the development of coronary artery disease in patients with and without diabetes mellitus: a review. Mol. Med. 24, 59. doi:10.1186/s10020-018-0060-3
Frangogiannis N. G. (2021). Cardiac fibrosis. Cardiovasc. Res. 117, 1450–1488. doi:10.1093/cvr/cvaa324
Frank O. (1898). Die Grundform des arteriellen pulses: erste abhandlung: mathematische analyse. Z. Biol. 37, 483–526.
Franklin S. S., Sutton-Tyrrell K., Belle S. H., Weber M. A., Kuller L. H. (1997). The importance of pulsatile components of hypertension in predicting carotid stenosis in older adults. J. Hypertens. 15, 1143–1150. doi:10.1097/00004872-199715100-00012
Franzoni F., Ghiadoni L., Galetta F., Plantinga Y., Lubrano V., Huang Y., et al. (2005). Physical activity, plasma antioxidant capacity, and endothelium-dependent vasodilation in young and older men. Am. J. Hypertens. 18, 510–516. doi:10.1016/j.amjhyper.2004.11.006
Fujimoto N., Hastings J. L., Carrick-Ranson G., Shafer K. M., Shibata S., Bhella P. S., et al. (2013). Cardiovascular effects of 1 year of alagebrium and endurance exercise training in healthy older individuals. Circ. Heart. Fail. 6, 1155–1164. doi:10.1161/CIRCHEARTFAILURE.113.000440
Fujimoto N., Prasad A., Hastings J. L., Bhella P. S., Shibata S., Palmer D., et al. (2012). Cardiovascular effects of 1 year of progressive endurance exercise training in patients with heart failure with preserved ejection fraction. Am. Heart. J. 164, 869–877. doi:10.1016/j.ahj.2012.06.028
Gaede P., Pedersen O. (2004). Intensive integrated therapy of type 2 diabetes: implications for long-term prognosis. Diabetes 53, 39–47. doi:10.2337/diabetes.53.suppl_3.s39
Gavin T. P., Ruster R. S., Carrithers J. A., Zwetsloot K. A., Kraus R. M., Evans C. A., et al. (2007). No difference in the skeletal muscle angiogenic response to aerobic exercise training between young and aged men. J. Physiol. 585, 231–239. doi:10.1113/jphysiol.2007.143198
GBD Diabetes Collaborators (2023). Global, regional, and national burden of diabetes from 1990 to 2021, with projections of prevalence to 2050: a systematic analysis for the Global Burden of Disease Study 2021. Lancet 402, 203–234. doi:10.1016/S0140-6736(23)01301-6
Gerdts E., Okin P. M., de Simone G., Cramariuc D., Wachtell K., Boman K., et al. (2008). Gender differences in left ventricular structure and function during antihypertensive treatment: the Losartan Intervention for Endpoint Reduction in Hypertension Study. Hypertension 51, 1109–1114. doi:10.1161/HYPERTENSIONAHA.107.107474
Gholami F., Khaki R., Mirzaei B., Howatson G. (2021). Resistance training improves nerve conduction and arterial stiffness in older adults with diabetic distal symmetrical polyneuropathy: a randomized controlled trial. Exp. Gerontol. 153, 111481. doi:10.1016/j.exger.2021.111481
Girerd X., Laurent S., Pannier B., Asmar R., Safar M. (1991). Arterial distensibility and left ventricular hypertrophy in patients with sustained essential hypertension. Am. Heart. J. 122, 1210–1214. doi:10.1016/0002-8703(91)90941-a
Gliemann L., Buess R., Nyberg M., Hoppeler H., Odriozola A., Thaning P., et al. (2015). Capillary growth, ultrastructure remodelling and exercise training in skeletal muscle of essential hypertensive patients. Acta. Physiol. (Oxf). 214, 210–220. doi:10.1111/apha.12501
Gliemann L., Olesen J., Bienso R. S., Schmidt J., Akerstrom T., Nyberg M., et al. (2014). Resveratrol modulates the angiogenic response to exercise training in skeletal muscles of aged men. Am. J. Physiol. Heart. Circ. Physiol. 307, 1111–1119. doi:10.1152/ajpheart.00168.2014
Gonzalez A., Schelbert E. B., Diez J., Butler J. (2018). Myocardial interstitial fibrosis in heart failure: biological and translational perspectives. J. Am. Coll. Cardiol. 71, 1696–1706. doi:10.1016/j.jacc.2018.02.021
Gori M., Lam C. S. P., D`Elia E., Iorio A. M., Calabrese A., Canova P., et al. (2021). Integrating natriuretic peptides and diastolic dysfunction to predict adverse events in high-risk asymptomatic subjects. Eur. J. Prev. Cardiol. 28, 937–945. doi:10.1177/2047487319899618
Gosse P. S. (2005). Left ventricular hypertrophy: the problem and possible solutions. J. Int. Med. Res. 33, 3A–11A. doi:10.1177/14732300050330S102
Green D. J., Hopman M. T. E., Padilla J., Laughlin M. H., Thijssen D. H. J. (2017). Vascular adaptation to exercise in humans: role of hemodynamic stimuli. Physiol. Rev. 97, 495–528. doi:10.1152/physrev.00014.2016
Groen M. B., Knudsen T. A., Finsen S. H., Pedersen B. K., Hellsten Y., Mortensen S. P. (2019). Reduced skeletal-muscle perfusion and impaired ATP release during hypoxia and exercise in individuals with type 2 diabetes. Diabetologia 62, 485–493. doi:10.1007/s00125-018-4790-0
Gunduz F., Kocer G., Ulker S., Meiselman H. J., Baskurt O. K., Senturk U. K. (2011). Exercise training enhances flow-mediated dilation in spontaneously hypertensive rats. Physiol. Res. 60, 589–597. doi:10.33549/physiolres.932166
Gunnarsson T. P., Ehlers T. S., Baasch-Skytte T., Lund A. P., Tamariz-Ellemann A., Gliemann L., et al. (2020a). Hypertension is associated with blunted NO-mediated leg vasodilator responsiveness that is reversed by high-intensity training in postmenopausal women. Am. J. Physiol. Regul. Integr. Comp. Physiol. 319, R712–R723. doi:10.1152/ajpregu.00170.2020
Gunnarsson T. P., Ehlers T. S., Fiorenza M., Nyberg M., Bangsbo J. (2020b). Essential hypertension is associated with blunted smooth muscle cell vasodilator responsiveness and is reversed by 10-20-30 training in men. Am. J. Physiol. Cell. Physiol. 318, C1252–C1263. doi:10.1152/ajpcell.00047.2020
Gustafsson T., Rundqvist H., Norrbom J., Rullman E., Jansson E., Sundberg C. J. (2007). The influence of physical training on the angiopoietin and VEGF-A systems in human skeletal muscle. J. Appl. Physiol. 103, 1012–1020. doi:10.1152/japplphysiol.01103.2006
Guyton A. C., Lindsey A. W., Abernathy B., Richardson T. (1957). Venous return at various right atrial pressures and the normal venous return curve. Am. J. Physiol. 189, 609–615. doi:10.1152/ajplegacy.1957.189.3.609
Haider A. W., Larson M. G., Franklin S. S., Levy D.Framingham Heart Study (2003). Systolic blood pressure, diastolic blood pressure, and pulse pressure as predictors of risk for congestive heart failure in the Framingham heart study. Ann. Intern. Med. 138, 10–16. doi:10.7326/0003-4819-138-1-200301070-00006
Hamilton M. T., Hamilton D. G., Zderic T. W. (2007). Role of low energy expenditure and sitting in obesity, metabolic syndrome, type 2 diabetes, and cardiovascular disease. Diabetes 56, 2655–2667. doi:10.2337/db07-0882
Hansen A. H., Nielsen J. J., Saltin B., Hellsten Y. (2010). Exercise training normalizes skeletal muscle vascular endothelial growth factor levels in patients with essential hypertension. J. Hypertens. 28, 1176–1185. doi:10.1097/HJH.0b013e3283379120
Hellsten Y., Jensen L., Thaning P., Nyberg M., Mortensen S. (2012a). Impaired formation of vasodilators in peripheral tissue in essential hypertension is normalized by exercise training: role of adenosine and prostacyclin. J. Hypertens. 30, 2007–2014. doi:10.1097/HJH.0b013e328356dd57
Hellsten Y., Maclean D., Radegran G., Saltin B., Bangsbo J. (1998). Adenosine concentrations in the interstitium of resting and contracting human skeletal muscle. Circulation 98, 6–8. doi:10.1161/01.cir.98.1.6
Hellsten Y., Nyberg M., Jensen L. G., Mortensen S. P. (2012b). Vasodilator interactions in skeletal muscle blood flow regulation. J. Physiol. 590, 6297–6305. doi:10.1113/jphysiol.2012.240762
Hellsten Y., Rufener N., Nielsen J. J., Hoier B., Krustrup P., Bangsbo J. (2008). Passive leg movement enhances interstitial VEGF protein, endothelial cell proliferation, and eNOS mRNA content in human skeletal muscle. Am. J. Physiol. Regul. Integr. Comp. Physiol. 294, 975–982. doi:10.1152/ajpregu.00677.2007
Higginbotham M. B., Morris K. G., Williams R. S., McHale P. A., Coleman R. E., Cobb F. R. (1986). Regulation of stroke volume during submaximal and maximal upright exercise in normal man. Circ. Res. 58, 281–291. doi:10.1161/01.res.58.2.281
Ho C. W., Beard J. L., Farrell P. A., Minson C. T., Kenney W. L. (1997). Age, fitness, and regional blood flow during exercise in the heat. J. Appl. Physiol. 82, 1126–1135. doi:10.1152/jappl.1997.82.4.1126
Hoier B., Hellsten Y. (2014). Exercise-induced capillary growth in human skeletal muscle and the dynamics of VEGF. Microcirculation 21, 301–314. doi:10.1111/micc.12117
Hoier B., Nordsborg N., Andersen S., Jensen L., Nybo L., Bangsbo J., et al. (2012). Pro- and anti-angiogenic factors in human skeletal muscle in response to acute exercise and training. J. Physiol. 590, 595–606. doi:10.1113/jphysiol.2011.216135
Hoiland R. L., Tremblay J. C., Stacey B. S., Coombs G. B., Nowak-Flück D., Tymko M. M., et al. (2020). Acute reductions in haematocrit increase flow-mediated dilatation independent of resting nitric oxide bioavailability in humans. J. Physiol. 598, 4225–4236. doi:10.1113/JP280141
Hori M., Inoue M., Kitakaze M., Tsujioka K., Ishida Y., Fukunami M., et al. (1985). Loading sequence is a major determinant of afterload-dependent relaxation in intact canine heart. Am. J. Physiol. 249, 747–754. doi:10.1152/ajpheart.1985.249.4.H747
Hsueh W. A., Wyne K. (2011). Renin-Angiotensin-aldosterone system in diabetes and hypertension. J. Clin. Hypertens. (Greenwich). 13, 224–237. doi:10.1111/j.1751-7176.2011.00449.x
Hudlicka O., Egginton M. B., Egginton S. (1992). Angiogenesis in skeletal and cardiac muscle. Physiol. Rev. 72, 369–417. doi:10.1152/physrev.1992.72.2.369
Hulett N. A., Scalzo R. L., Reusch J. E. B. (2022). Glucose uptake by skeletal muscle within the contexts of type 2 diabetes and exercise: an integrated approach. Nutrients 14, 647. doi:10.3390/nu14030647
Ingjer F. (1979a). Effects of endurance training on muscle fibre ATP-ase activity, capillary supply and mitochondrial content in man. J. Physiol. 294, 419–432. doi:10.1113/jphysiol.1979.sp012938
Ingjer F. (1979b). Capillary supply and mitochondrial content of different skeletal muscle fiber types in untrained and endurance-trained men: a histochemical and ultrastructural study. Eur. J. Appl. Physiol. Occup. Physiol. 40, 197–209. doi:10.1007/BF00426942
Jellis C., Wright J., Kennedy D., Sacre J., Jenkins C., Haluska B., et al. (2011). Association of imaging markers of myocardial fibrosis with metabolic and functional disturbances in early diabetic cardiomyopathy. Circ. Cardiovasc. Imag. 4, 693–702. doi:10.1161/CIRCIMAGING.111.963587
Jensen L., Bangsbo J., Hellsten Y. (2004). Effect of high intensity training on capillarization and presence of angiogenic factors in human skeletal muscle. J. Physiol. 557, 571–582. doi:10.1113/jphysiol.2003.057711
Jørgensen P. G., Biering-Sørensen T., Mogelvang R., Fritz-Hansen T., Vilsbøl T., Rossing P., et al. (2019). Predictive value of echocardiography in Type 2 diabetes. Eur. Heart. J. Cardiovasc. Imag. 20, 687–693. doi:10.1093/ehjci/jey164
Joyner M. J., Casey D. P. (2015). Regulation of increased blood flow (hyperemia) to muscles during exercise: a hierarchy of competing physiological needs. Physiol. Rev. 95, 549–601. doi:10.1152/physrev.00035.2013
Juguilon C., Wang Z., Wang Y., Enrick M., Jamiyar A., Xu Y., et al. (2022). Mechanism of the switch from NO to H2O2 in endothelium-dependent vasodilation in diabetes. Basic. Res. Cardiol. 117, 2. doi:10.1007/s00395-022-00910-1
Kaess B. M., Rong J., Larson M. G., Hamburg N. M., Vita J. A., Levy D., et al. (2012). Aortic stiffness, blood pressure progression, and incident hypertension. JAMA 308, 875–881. doi:10.1001/2012.jama.10503
Kelly R. P., Tunin R., Kass D. A. (1992). Effect of reduced aortic compliance on cardiac efficiency and contractile function of in situ canine left ventricle. Circ. Res. 71, 490–502. doi:10.1161/01.res.71.3.490
Keytsman C., Dendale P., Hansen D. (2015). Chronotropic incompetence during exercise in type 2 diabetes: aetiology, assessment methodology, prognostic impact and therapy. Sports Med. 45, 985–995. doi:10.1007/s40279-015-0328-5
Kim T. I., Guzman R. J. (2023). Medial artery calcification in peripheral artery disease. Front. Cardiovasc. Med. 10, 1093355. doi:10.3389/fcvm.2023.1093355
Kim Y. S., Seifert T., Brassard P., Rasmussen P., Vaag A., Nielsen H. B., et al. (2015). Impaired cerebral blood flow and oxygenation during exercise in type 2 diabetic patients. Physiol. Rep. 3, e12430. doi:10.14814/phy2.12430
Kingwell B. A., Berry K. L., Cameron J. D., Jennings G. L., Dart A. M. (1997). Arterial compliance increases after moderate-intensity cycling. Am. J. Physiol. 273, 2186–2191. doi:10.1152/ajpheart.1997.273.5.H2186
Kirby B. S., Voyles W. F., Simpson C. B., Carlson R. E., Schrage W. G., Dinenno F. A. (2009). Endothelium-dependent vasodilatation and exercise hyperaemia in ageing humans: impact of acute ascorbic acid administration. J. Physiol. 587, 1989–2003. doi:10.1113/jphysiol.2008.167320
Kivelä R., Sivennoinen M., Touvra A.-M., Lehti T. M., Kainulainen H., Vihko V. (2006). Effects of experimental type 1 diabetes and exercise training on angiogenic gene expression and capillarization in skeletal muscle. Faseb. J. 20, 1570–1572. doi:10.1096/fj.05-4780fje
Klausen K., Andersen L. B., Pelle I. (1981). Adaptive changes in work capacity, skeletal muscle capillarization and enzyme levels during training and detraining. Acta Physiol. Scand. 113, 9–16. doi:10.1111/j.1748-1716.1981.tb06854.x
Kohno F., Kumada T., Kamabayashi M., Hayashida W., Ishikawa N., Sasayama S. (1996). Change in aortic end systolic pressure by alterations in loading sequence and its relation to left ventricular isovolumic relaxation. Circulation 93, 2080–2087. doi:10.1161/01.cir.93.11.2080
Kosiborod M. N., Abildstrøm S. Z., Borlaug B. A., Butler J., Rasmussen S., Davies M., et al. (2023). Semaglutide in patients with heart failure with preserved ejection fraction and obesity. N. Engl. J. Med. 389, 1069–1084. doi:10.1056/NEJMoa2306963
Krip B., Gledhill N., Jamnik V., Warburton D. (1997). Effect of alterations in blood volume on cardiac function during maximal exercise. Med. Sci. Sports. Exerc. 29, 1469–1476. doi:10.1097/00005768-199711000-00013
Kyhl K., von Huth S., Bojer A., Thomsen C., Engstrøm T., Vejlstrup N., et al. (2021). Conductance artery stiffness impairs atrio-ventriculo-arterial coupling before manifestation of arterial hypertension or left ventricular hypertrophic remodelling. Sci. Reps. 11, 14467. doi:10.1038/s41598-021-93614-w
Langille B. L., O’Donnell F. (1986). Reductions in arterial diameter produced by chronic decreases in blood flow are endothelium-dependent. Science 231, 405–407. doi:10.1126/science.3941904
Lanzer P., Hannan F. M., Lanzer J. D., Janzen J., Raggi P., Furniss D., et al. (2021). Medial arterial calcification: JACC state-of-the-art review. J. Am. Coll. Cardiol. 78, 1145–1165. doi:10.1016/j.jacc.2021.06.049
Lav Madsen P. (2022). “Biomarkers of myocardial fibrosis in diabetes, echocardiography, and magnetic resonance imaging,” in Preedy VR. red. Biomarkers in Diabetes. Biomarkers in disease: methods, discoveries and applications. Editor V. B. Patel (Springer).
Levine B. D. (2008). VO2max: what do we know, and what do we still need to know? J. Physiol. 586 (1), 25–34. doi:10.1113/jphysiol.2007.147629
Levy D., Garrison R. J., Savage D. D., Kannel W. B., Castelli W. P. (1990). Prognostic implications of echocardiographically determined left ventricular mass in the Framingham Heart Study. N. Engl. J. Med. 322, 1561–1566. doi:10.1056/NEJM199005313222203
Li C., Zhang J., Xue M., Li X., Han F., Liu X., et al. (2019). SGLT2 inhibition with empagliflozin attenuates myocardial oxidative stress and fibrosis in diabetic mice heart. Cardiovasc. Diabetol. 18, 15. doi:10.1186/s12933-019-0816-2
Lithell H., Krotkiewski M., Kiens B., Wroblewski Z., Holm G., Strömblad G., et al. (1985). Non-response of muscle capillary density and lipoprotein-lipase activity to regular training in diabetic patients. Diabetes Res. 2, 17–21.
Lloyd P. G., Prior B. M., Li H., Yang H. T., Terjung R. L. (2005). VEGF receptor antagonism blocks arteriogenesis, but only partially inhibits angiogenesis, in skeletal muscle of exercise-trained rats. Am. J. Physiol. Heart. Circ. Physiol. 288, 759–768. doi:10.1152/ajpheart.00786.2004
London G. M., Marchais S. J., Guerin A. P., Pannier B. (2004). Arterial stiffness: pathophysiology and clinical impact. Clin. Exp. Hypertens. 26, 689–699. doi:10.1081/ceh-200031982
Lønnebakken M. T., Izzo R., Mancusi C., Gerdts E., Losi M. A., Canciello G., et al. (2017). Left ventricular hypertrophy regression during antihypertensive treatment in an outpatient clinic (the Campania Salute Network). J. Am. Heart. Assoc. 6, e004152. doi:10.1161/JAHA.116.004152
Lopes S., Afreixo V., Teixeira M., Garcia C., Leitão C., Gouveia M., et al. (2021). Exercise training reduces arterial stiffness in adults with hypertension: a systematic review and meta-analysis. J. Hypertens. 39, 214–222. doi:10.1097/HJH.0000000000002619
Love K. M., Liu J., Regensteiner J. G., Reusch J. E. B., Liu Z. (2020). GLP-1 and insulin regulation of skeletal and cardiac muscle microvascular perfusion in type 2 diabetes. J. Diabetes. 12, 488–498. doi:10.1111/1753-0407.13045
Lundbaek K. (1954). Diabetic angiopathy: a specific vascular disease. Lancet 266, 377–379. doi:10.1016/s0140-6736(54)90924-1
Lundbaek K. (1969). “Is there a diabetic cardiopathy?,” in Pathogenetische faktoren des myokardinfarkts. Editor G. R. Schettler (Stuttgart: Schattauer Verlag), 63–71.
Madhavan S., Ooi W. L., Cohen H., Alderman M. H. (1994). Relation of pulse pressure and blood pressure reduction to the incidence of myocardial infarction. Hypertension 23, 395–401. doi:10.1161/01.hyp.23.3.395
Madsen P. L., Scheuermann Freestone M., Neubauer S., Channon K., Clarke K. (2006). Haemoglobin and flow-mediated vasodilation. Clin. Sci. 110, 467–473. doi:10.1042/CS20050291
Maeda S., Sugawara J., Yoshizawa M., Otsuki T., Shimojo N., Jesmin S., et al. (2009). Involvement of endothelin-1 in habitual exercise-induced increase in arterial compliance. Acta. Physiol. (Oxf). 196, 223–229. doi:10.1111/j.1748-1716.2008.01909.x
Magalhaes J. P., Melo X., Correia I. R., Ribeiro R. T., Raposo J., Dores H., et al. (2019). Effects of combined training with different intensities on vascular health in patients with type 2 diabetes: a 1-year randomized controlled trial. Cardiovasc. Diabetol. 18, 34. doi:10.1186/s12933-019-0840-2
Maiorana A., O´Driscoll G., Cheetham C., Dembo L., Stanton K., Goodman C., et al. (2001). The effect of combined aerobic and resistance exercise training on vascular function in type 2 diabetes. J. Am. Coll. Cardiol. 38, 860–866. doi:10.1016/s0735-1097(01)01439-5
Mason T., Coehlo-Filho O. R., Verma S., Chowdhury B., Zuo F., Quan A., et al. (2021). Empagliflozin reduces myocardial extracellular volume in patients with type 2 diabetes and coronary artery disease. J. Am. Coll. Cardiol. Imaging. 14, 1164–1173. doi:10.1016/j.jcmg.2020.10.017
McEniery C. M., McDonnell B. J., So A., Aitken S., Bolten C. E., Munnery M., et al. (2009). Aortic calcification is associated with aortic stiffness and isolated systolic hypertension in healthy individuals. Hypertension 53, 524–531. doi:10.1161/HYPERTENSIONAHA.108.126615
Milnor W. R. (1975). Arterial impedance as ventricular afterload. Circ. Res. 36, 565–570. doi:10.1161/01.res.36.5.565
Mitchell J. H., Kaufman M. P., Iwamoto G. A. (1983). The exercise pressor reflex: its cardiovascular effects, afferent mechanisms, and central pathways. Annu. Rev. Physiol. 45, 229–242. doi:10.1146/annurev.ph.45.030183.001305
Mohan M., McSwiggan S., Baig F., Rutherford L., Lang C. C. (2015). Metformin and its effects on myocardial dimension and left ventricular hypertrophy in normotensive patients with coronary heart disease (the MET-REMODEL study): rationale and design of the MET-REMODEL study. Cardiovasc. Ther. 33, 1–8. doi:10.1111/1755-5922.12101
Mohiaddin R. H., Underwood S. R., Bogren H. G., Firmin D. N., Klipstein R. H., Rees R. S., et al. (1989). Regional aortic compliance studied by magnetic resonance imaging: the effects of age, training, and coronary artery disease. Br. Heart. J. 62, 90–96. doi:10.1136/hrt.62.2.90
Montero D., Diaz-Canestro C., Oberholzer L., Lundby C. (2019). The role of blood volume in cardiac dysfunction and reduced exercise tolerance in patients with diabetes. Lancet Diabetes Endocrinol. 7, 807–816. doi:10.1016/S2213-8587(19)30119-6
Mortensen S. P., Damsgaard R., Dawson E. A., Secher N. H., Gonzalez-Alonso J. (2008). Restrictions in systemic and locomotor skeletal muscle perfusion, oxygen supply and VO2 during high-intensity whole-body exercise in humans. J. Physiol. 586, 2621–2635. doi:10.1113/jphysiol.2007.149401
Mortensen S. P., Dawson E. A., Yoshiga C. C., Dalsgaard M. K., Damsgaard R., Secher N. H., et al. (2005). Limitations to systemic and locomotor limb muscle oxygen delivery and uptake during maximal exercise in humans. J. Physiol. 566, 273–285. doi:10.1113/jphysiol.2005.086025
Mortensen S. P., Egginton S., Madsen M., Hansen J. B., Munch G. D. W., Iepsen U. W., et al. (2017). Alpha adrenergic receptor blockade increases capillarization and fractional O2 extraction and lowers blood flow in contracting human skeletal muscle. Acta Physiol. (Oxf). 22, 32–43. doi:10.1111/apha.12857
Mortensen S. P., Nyberg M., Gliemann L., Thaning P., Saltin B., Hellsten Y. (2014). Exercise training modulates functional sympatholysis and α-adrenergic vasoconstrictor responsiveness in hypertensive and normotensive individuals. J. Physiol. 592, 3063–3073. doi:10.1113/jphysiol.2014.273722
Mortensen S. P., Nyberg M., Thaning P., Saltin B., Hellsten Y. (2009). Adenosine contributes to blood flow regulation in the exercising human leg by increasing prostaglandin and nitric oxide formation. Hypertension 53, 993–999. doi:10.1161/HYPERTENSIONAHA.109.130880
Mortensen S. P., Svendsen J. H., Ersboll M., Hellsten Y., Secher N. H., Saltin B. (2013). Skeletal muscle signaling and the heart rate and blood pressure response to exercise: insight from heart rate pacing during exercise with a trained and a deconditioned muscle group. Hypertension 61, 1126–1133. doi:10.1161/HYPERTENSIONAHA.111.00328
Mortensen S. P., Winding K. M., Jepsen U. W., Munch G. W., Marcussen N., Hellsten Y., et al. (2019). The effect of two exercise modalities on skeletal muscle capillary ultrastructure in individuals with type 2 diabetes. Scand. J. Med. Sci. Sports. 29, 360–368. doi:10.1111/sms.13348
Mulvany M. J., Hansen O. K., Aalkjaer C. (1978). Direct evidence that the greater contractility of resistance vessels in spontaneously hypertensive rats is associated with a narrowed lumen, a thickened media, and an increased number of smooth muscle cell layers. Circ. Res. 43, 854–864. doi:10.1161/01.res.43.6.854
Munch G. D. W., Svendsen J. H., Damsgaard R., Secher N. H., Gonzáles-Alonso J., Mortensen S. P. (2014). Maximal heart rate does not limit cardiovascular capacity in healthy humans: insight from right atrial pacing during maximal exercise. J. Physiol. 592, 377–390. doi:10.1113/jphysiol.2013.262246
Murrant C. L., Sarelius I. H. (2000). Coupling of muscle metabolism and muscle blood flow in capillary units during contraction. Acta. Physiol. Scand. 168, 531–541. doi:10.1046/j.1365-201x.2000.00706.x
Nesti L., Pugliese N. R., Sciuto P., Natali A. (2020). Type 2 diabetes and reduced exercise tolerance: a review of the literature through an integrated physiology approach. Cardiovasc. Diabetol. 19, 134. doi:10.1186/s12933-020-01109-1
Nyberg M., Blackwell J. R., Damsgaard R., Jones A. M., Hellsten Y., Mortensen S. P. (2012a). Lifelong physical activity prevents an age-related reduction in arterial and skeletal muscle nitric oxide bioavailability in humans. J. Physiol. 590, 5361–5370. doi:10.1113/jphysiol.2012.239053
Nyberg M., Jensen L. G., Thaning P., Hellsten Y., Mortensen S. P. (2012b). Role of nitric oxide and prostanoids in the regulation of leg blood flow and blood pressure in humans with essential hypertension: effect of high-intensity aerobic training. J. Physiol. 590, 1481–1494. doi:10.1113/jphysiol.2011.225136
Nyberg M., Mortensen S. P., Cabo H., Gomez-Cabrera M.-C., Viña J., Hellsten Y. (2014). Roles of sedentary aging and lifelong physical activity in exchange of glutathione across exercising human skeletal muscle. Free Radic. Biol. Med. 73, 166–173. doi:10.1016/j.freeradbiomed.2014.05.008
Nyberg M., Mortensen S. P., Hellsten Y. (2013). Physical activity opposes the age-related increase in skeletal muscle and plasma endothelin-1 levels and normalizes plasma endothelin-1 levels in individuals with essential hypertension. Acta Physiol. (Oxf). 207, 524–535. doi:10.1111/apha.12048
Ohara K., Masuda T., Murakami T., Imai T., Yoshizawa H., Nakagawa S., et al. (2019). Effects of the sodium-glucose cotransporter 2 inhibitor dapagliflozin on fluid distribution: a comparison study with furosemide and tolvaptan. Nephrol. Carlt. 24, 904–911. doi:10.1111/nep.13552
Olver T. D., Laughlin M. H. (2016). Endurance, interval sprint, and resistance exercise training: impact on microvascular dysfunction in type 2 diabetes. Am. J. Physiol. Heart. Circ. Physiol. 310, H337–H350. doi:10.1152/ajpheart.00440.2015
Patterson S. W., Piper H., Starling E. H. (1914). The regulation of the heart beat. J. Physiol. 48, 465–513. doi:10.1113/jphysiol.1914.sp001676
Patterson S. W., Starling E. H. (1914). On the mechanical factors which determine the output of the ventricles. J. Physiol. 48, 357–379. doi:10.1113/jphysiol.1914.sp001669
Pelliccia A., Maron B. J., Culasso F., Spataro A., Caselli G. (1996). Athlete’s heart in women. Echocardiographic characterization of highly trained elite female athletes. JAMA 276, 211–215. doi:10.1001/jama.276.3.211
Pfeifer M., Townsend R. R., Davies M. J., Vijapurkar U., Ren J. (2017). Effects of canagliflozin, a sodium glucose co-transporter 2 inhibitor, on blood pressure and markers of arterial stiffness in patients with type 2 diabetes mellitus: a post hoc analysis. Cardiovasc. Diabetol. 16, 29. doi:10.1186/s12933-017-0511-0
Pinto T. E., Gusso S., Hofman P. L., Derraik J. G. B., Hornung T. S., Cutfield W. S., et al. (2014). Systolic and diastolic abnormalities reduce the cardiac response to exercise in adolescents with type 2 diabetes. Diabetes Care 37, 1439–1446. doi:10.2337/dc13-2031
Pipp F., Boehm S., Cai W. J., Adili F., Ziegler B., Karanovic G., et al. (2004). Elevated fluid shear stress enhances postocclusive collateral artery growth and gene expression in the pig hind limb. Arterioscler. Thromb. Vasc. Biol. 24, 1664–1668. doi:10.1161/01.ATV.0000138028.14390.e4
Pluim B. M., Zwinderman A. H., van der Laarse A., van der Wall E. E. (2000). The athlete's heart: a meta-analysis of cardiac structure and function. Circ 101, 336–344. doi:10.1161/01.cir.101.3.336
Qiu S., Cai X., Yin H., Sun Z., Zügel M., Steinacker J. M., et al. (2018). Exercise training and endothelial function in patients with type 2 diabetes: a meta-analysis. Cardiovasc. Diabetol. 17, 64. doi:10.1186/s12933-018-0711-2
Radegran G., Calbet J. A. (2001). Role of adenosine in exercise-induced human skeletal muscle vasodilatation. Acta Physiol. Scand. 171, 177–185. doi:10.1046/j.1365-201x.2001.00796.x
Ray C. A. (1999). Sympathetic adaptations to one-legged training. J. Appl. Physiol. 86, 1583–1587. doi:10.1152/jappl.1999.86.5.1583
Regensteiner J. G., Bauer T. A., Reusch J. E., Brandenburg S. L., Sippel J. M., Vogelsong A. M., et al. (1998). Abnormal oxygen uptake kinetic responses in women with type II diabetes mellitus. J. Appl. Physiol. 85, 310–317. doi:10.1152/jappl.1998.85.1.310
Rekhraj S., Gandy S. J., Szwejkowski B. R., Nadir M. A., Noman A., Houston J. G., et al. (2013). High-dose allopurinol reduces left ventricular mass in patients with ischemic heart disease. J. Am. Coll. Cardiol. 61, 926–932. doi:10.1016/j.jacc.2012.09.066
Richardson R. S., Poole D. C., Knight D. R., Kurdak S. S., Hogan M. C., Grassi B., et al. (1993). High muscle blood flow in man: is maximal O2 extraction compromised? J. Appl. Physiol. 75, 1911–1916. doi:10.1152/jappl.1993.75.4.1911
Roberts T. J., Burns A. T., MacIsaac R. J., MacIsaac A. I., Prior D. L., La Gerche A. (2018). Exercise capacity in diabetes mellitus is predicted by activity status and cardiac size rather than cardiac function: a case control study. Cardiovasc. Diabetol. 17, 44. doi:10.1186/s12933-018-0688-x
Rubanyi G. M., Romero J. C., Vanhoutte P. M. (1986). Flow-induced release of endothelium-derived relaxing factor. Am. J. Physiol. 250, 1145–1149. doi:10.1152/ajpheart.1986.250.6.H1145
Rubler S. J., Dlugash J., Yuceoglu Y. Z., Kumral T., Branwood A. W., Grishman A. (1972). New type of cardiomyopathy associated with diabetic glomerulosclerosis. Am. J. Cardiol. 30, 595–602. doi:10.1016/0002-9149(72)90595-4
Rush J. W., Aultman C. D. (2008). Vascular biology of angiotensin and the impact of physical activity. Appl. Physiol. Nutr. Metab. 33, 162–172. doi:10.1139/H07-147
Russo I., Frangogiannis N. G. (2016). Diabetes-associated cardiac fibrosis: cellular effectors, molecular mechanisms and therapeutic opportunities. J. Mol. Cell. Cardiol. 90, 84–93. doi:10.1016/j.yjmcc.2015.12.011
Saba P. S., Roman M. J., Pini R., Spitzer M., Ganau A., Devereux R. B. (1993). Relation of arterial pressure waveform to left ventricular and carotid anatomy in normotensive subjects. J. Am. Coll. Cardiol. 22, 1873–1880. doi:10.1016/0735-1097(93)90772-s
Saco-Ledo G., Valenzuela P. L., Ruilope L. M., Lucia A. (2022). Physical exercise in resistant hypertension: a systematic review and meta-analysis of randomized controlled trials. Front. Cardiovasc. Med. 9, 893811. doi:10.3389/fcvm.2022.893811
Sacre J. W., Jellis C. L., Haluska B. A., Jenkins C., Coombes J. S., Marwick T. H., et al. (2015). Association of Exercise Intolerance in type 2 diabetes with skeletal muscle blood flow reserve. JACC. Cardiovasc. Imaging 8, 913–921. doi:10.1016/j.jcmg.2014.12.033
Sawabe M., Takahashi R., Matsushita S., Ozawa T., Arai T., Hamamatsu A., et al. (2005). Aortic pulse wave velocity and the degree of atherosclerosis in the elderly: a pathological study based on 304 autopsy cases. Atherosclerosis 179, 345–351. doi:10.1016/j.atherosclerosis.2004.09.023
Scharhag J., Schneider G., Urhausen A., Rochette V., Kramann B., Kindermann W. (2002). Athlete’s heart: right and left ventricular mass and function in male endurance athletes and untrained individuals determined by magnetic resonance imaging. J. Am. Coll. Cardiol. 40, 1856–1863. doi:10.1016/s0735-1097(02)02478-6
Schreuder T. H. A., Green D. J., Nyakayiru J., Hopman M. T. E., Thijssen D. H. J. (2015). Time-course of vascular adaptations during 8 weeks of exercise training in subjects with type 2 diabetes and middle-aged controls. Eur. J. Appl. Physiol. 115, 187–196. doi:10.1007/s00421-014-3006-7
Schultz M. G., Otahal P., Cleland V. J., Blizzard L., Marwick T. H., Sharman J. E. (2013). Exercise-induced hypertension, cardiovascular events, and mortality in patients undergoing exercise stress testing: a systematic review and meta-analysis. Am. J. Hypertens. 26, 357–366. doi:10.1093/ajh/hps053
Schultz M. G., Otahal P., Kovacevic A.-M., Roberts-Thomson P., Stanton T., Hamilton-Craig C., et al. (2022). Type-2 diabetes and the clinical importance of exaggerated exercise blood pressure. Hypertension 79, 2346–2354. doi:10.1161/HYPERTENSIONAHA.122.19420
Segal S. S., Duling B. R. (1989). Conduction of vasomotor responses in arterioles: a role for cell-to-cell coupling? Am. J. Physiol. 256, 838–845. doi:10.1152/ajpheart.1989.256.3.H838
Semba R. D., Najjar S. S., Sun K., Lakatta E. W. G., Ferrucci L. (2009). Serum carboxymethyl-lysine, an advanced glycation end product, is associated with increased aortic pulse wave velocity in adults. Am. J. Hypertens. 22, 74–79. doi:10.1038/ajh.2008.320
Shen L., You B.-A., Gao H.-Q., Li B.-Y., Yu F., Pei F. (2012). Effects of phlorizin on vascular complications in diabetes db/db mice. Chin. Med. J. (Engl.) 125, 3692–3696.
Shi C., Zhang H., Zhang N., Liu D., Fan Z., Sun Z., et al. (2022). The dynamic characteristics of myocardial contractility and extracellular volume in type 2 diabetes mellitus mice investigated by 7.0T cardiac magnetic resonance. J. Clin. Med. 11, 4262. doi:10.3390/jcm11154262
Shibata S., Hastings J. L., Prasad A., Fu Q., Bhella P. S., Pacini E., et al. (2011). Congestive heart failure with preserved ejection fraction is associated with severely impaired dynamic Starling mechanism. J. Appl. Physiol. 110, 964–971. doi:10.1152/japplphysiol.00826.2010
Singel D. J., Stamler J. S. (2005). Chemical physiology of blood flow regulation by red blood cells: the role of nitric oxide and S-nitrosohemoglobin. Annu. Rev. Physiol. 67, 99–145. doi:10.1146/annurev.physiol.67.060603.090918
Sinoway L. I., Musch T. I., Minotti J. R., Zelis R. (1986). Enhanced maximal metabolic vasodilatation in the dominant forearms of tennis players. J. Appl. Physiol. 61, 673–678. doi:10.1152/jappl.1986.61.2.673
Sinoway L. I., Shenberger J., Wilson J., McLaughlin D., Musch T., Zelis R. (1987). A 30-day forearm work protocol increases maximal forearm blood flow. J. Appl. Physiol. 62, 1063–1067. doi:10.1152/jappl.1987.62.3.1063
Sørensen M. H., Bojer A. S., Broadbent D. A., Plein S., Madsen P. L., Gæde P. (2020a). Cardiac perfusion, structure, and function in type 2 diabetes mellitus with and without diabetic complications. Eur. Heart. J. Cardiovasc. Imaging. 21, 887–895. doi:10.1093/ehjci/jez266
Sørensen M. H., Bojer A. S., Jørgensen N. R., Broadbent D. A., Plein S., Madsen P. L., et al. (2020b). Fibroblast growth factor-23 is associated with imaging markers of diabetic cardiomyopathy and anti-diabetic therapeutics. Cardiovasc. Diabetol. 19, 158. doi:10.1186/s12933-020-01135-z
Sørensen M. H., Bojer A. S., Pontoppidan J. R. N., Broadbent D. A., Plein S., Madsen P. L., et al. (2020c). Reduced myocardial perfusion reserve in type 2 diabetes is caused by increased perfusion at rest and decreased maximal perfusion during stress. Diabetes. Care. 43, 1285–1292. doi:10.2337/dc19-2172
Starling E. H., Visscher M. B. (1926). The regulation of the energy output of the heart. J. Physiol. 62, 243–261. doi:10.1113/jphysiol.1927.sp002355
Szwejkowski B. R., Gandy S. J., Rekhraj S., Houston J. G., Lang C. C., Morris A. D., et al. (2013). Allopurinol reduces left ventricular mass in patients with type 2 diabetes and left ventricular hypertrophy. J. Am. Coll. Cardiol. 62, 2284–2293. doi:10.1016/j.jacc.2013.07.074
Taddei S., Galetta F., Virdis A., Ghiadoni L., Salvetti G., Franzoni F., et al. (2000). Physical activity prevents age-related impairment in nitric oxide availability in elderly athletes. Circulation 101, 2896–2901. doi:10.1161/01.cir.101.25.2896
Thaning P., Bune L. T., Hellsten Y., Pilegaard H., Saltin B., Rosenmeier J. B. (2010). Attenuated purinergic receptor function in patients with type 2 diabetes. Diabetes 59, 182–189. doi:10.2337/db09-1068
Thaning P., Bune L. T., Zaar M., Saltin B., Rosenmeier J. B. (2011). Functional sympatholysis during exercise in patients with type 2 diabetes with intact response to acetylcholine. Diabetes Care 34, 1186–1191. doi:10.2337/dc10-2129
Thijssen D. H. J., Cable N. T., Green D. J. (2012). Impact of exercise training on arterial wall thickness in humans. Clin. Sci. (Lond.) 122 (7), 311–322. doi:10.1042/CS20110469
Thisted L., Correia C., Jennbacken K., Wagberg M., Wichern F., Madsen M., et al. (2021). Abstract P380: common and distinct signatures of interstitial and perivascular fibrosis in mouse models of hypertensive heart disease. Circ. Res. 129, AP380. (Poster). doi:10.1161/res.129.suppl_1.p380
Thomas T. P., Grisanti L. A. (2020). The dynamic interplay between cardiac inflammation and fibrosis. Front. Physiol. 11, 529075. doi:10.3389/fphys.2020.529075
Toprak C., Yigitaslan S. (2019). Alagebrium and complications of diabetes mellitus. Eurasian. J. Med. 51, 285–292. doi:10.5152/eurasianjmed.2019.18434
Trujillo-Santos A. J. (2003). Diabetic muscle infarction: an underdiagnosed complication of long-standing diabetes. Diabetes Care 26, 211–215. doi:10.2337/diacare.26.1.211
Tuttle J. L., Nachreiner R. D., Bhuller A. S., Condict K. W., Connors B. A., Herring B. P., et al. (2001). Shear level influences resistance artery remodeling: wall dimensions, cell density, and eNOS expression. Am. J. Physiol. Heart. Circ. Physiol. 281, 1380–1389. doi:10.1152/ajpheart.2001.281.3.H1380
Ubels F. L., Muntinga J. H. J., Links T. P., Hoogenberg K., Dullaart R. P. F., Reitsma W. D., et al. (2001). Redistribution of blood volume in Type I diabetes. Diabetologia 44, 429–432. doi:10.1007/s001250051639
Urschel C. W., Covel J. W., Sonnenblick E. H., Ross J., Braunwald E. (1968). Effects of decreased aortic compliance on performance of the left ventricle. Am. J. Physiol. 214, 298–304. doi:10.1152/ajplegacy.1968.214.2.298
Wang E., Solli G. S., Nyberg S. K., Hoff J., Helgerud J. (2012). Stroke volume does not plateau in female endurance athletes. Int. J. Sports. Med. 33, 734–739. doi:10.1055/s-0031-1301315
Watanabe H., Ohtsuka S., Kakihana M., Sugishita Y. (1993). Coronary circulation in dogs with an experimental decrease in aortic compliance. J. Am. Coll. Cardiol. 21, 1497–1506. doi:10.1016/0735-1097(93)90330-4
Willenberg T., Schumacher A., Amann-Vesti B., Jacomella V., Thalhammer C., Diehm N., et al. (2010). Impact of obesity on venous hemodynamics of the lower limbs. J. Vasc. Surg. 52, 664–668. doi:10.1016/j.jvs.2010.04.023
Wilson G. A., Wilkins G. T., Cotter J. D., Lamberts R. R., Lal S., Baldi J. C. (2017). Impaired ventricular filling limits cardiac reserve during submaximal exercise in people with type 2 diabetes. Cardiovasc. Diabetol. 16, 160. doi:10.1186/s12933-017-0644-1
Winder W. W., Hagberg J. M., Hickson R. C., Ehsani A. A., McLane J. A. (1978). Time course of sympathoadrenal adaptation to endurance exercise training in man. J. Appl. Physiol. Respir. Environ. Exerc. Physiol. 45, 370–374. doi:10.1152/jappl.1978.45.3.370
Womack L., Peters D., Barrett E. J., Kaul S., Price W., Lindner J. R. (2009). Abnormal skeletal muscle capillary recruitment during exercise in patients with type 2 diabetes mellitus and microvascular complications. J. Am. Coll. Cardiol. 53 (23), 2175–2183. doi:10.1016/j.jacc.2009.02.042
Woodman C. R., Muller J. M., Rush J. W., Laughlin M. H., Price E. M. (1999). Flow regulation of ecNOS and Cu/Zn SOD mRNA expression in porcine coronary arterioles. Am. J. Physiol. 276, 1058–1063. doi:10.1152/ajpheart.1999.276.3.H1058
Yang E. Y., Ghosn M. G., Khan M. A., Gramze N. L., Brunner G., Nabi F., et al. (2019). Myocardial extracellular volume fraction adds prognostic information beyond myocardial replacement fibrosis. Circ. Cardiovasc. Imaging 12, e009535. doi:10.1161/CIRCIMAGING.119.009535
Zieman S. J., Melenovsky V., Kass D. A. (2005). Mechanisms, pathophysiology, and therapy of arterial stiffness. Arterioscler. Thromb. Vasc. Biol. 25, 932–943. doi:10.1161/01.ATV.0000160548.78317.29
Keywords: type 2 diabetes mellitus, heart failure with a preserved ejection fraction, exercise intolerance, hypertension, cardiac fibrosis, myocardial blood flow and flow reserve
Citation: Lav Madsen P, Sejersen C, Nyberg M, Sørensen MH, Hellsten Y, Gaede P and Bojer AS (2024) The cardiovascular changes underlying a low cardiac output with exercise in patients with type 2 diabetes mellitus. Front. Physiol. 15:1294369. doi: 10.3389/fphys.2024.1294369
Received: 14 September 2023; Accepted: 19 February 2024;
Published: 20 March 2024.
Edited by:
Jianxin Deng, Shenzhen Second People’s Hospital, ChinaReviewed by:
Pietro Scicchitano, ASLBari—Azienda Sanitaria Localedella provincia di Bari (ASL BA), ItalyCopyright © 2024 Lav Madsen, Sejersen, Nyberg, Sørensen, Hellsten, Gaede and Bojer. This is an open-access article distributed under the terms of the Creative Commons Attribution License (CC BY). The use, distribution or reproduction in other forums is permitted, provided the original author(s) and the copyright owner(s) are credited and that the original publication in this journal is cited, in accordance with accepted academic practice. No use, distribution or reproduction is permitted which does not comply with these terms.
*Correspondence: Per Lav Madsen, bGF2Lm1hZHNlbkBnbWFpbC5jb20=; Annemie Stege Bojer, YXNib2plckBnbWFpbC5jb20=
Disclaimer: All claims expressed in this article are solely those of the authors and do not necessarily represent those of their affiliated organizations, or those of the publisher, the editors and the reviewers. Any product that may be evaluated in this article or claim that may be made by its manufacturer is not guaranteed or endorsed by the publisher.
Research integrity at Frontiers
Learn more about the work of our research integrity team to safeguard the quality of each article we publish.