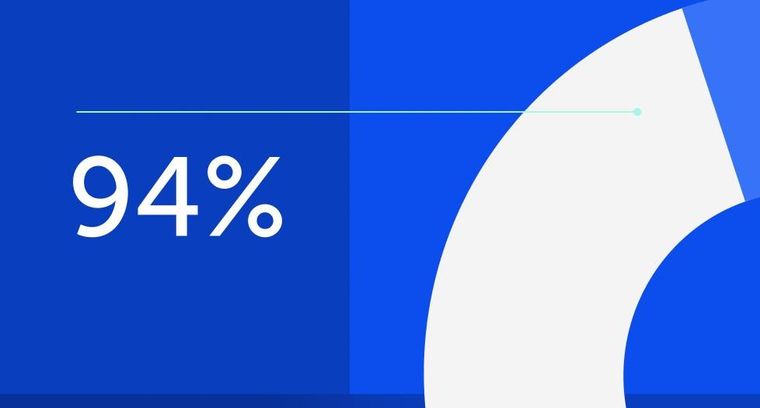
94% of researchers rate our articles as excellent or good
Learn more about the work of our research integrity team to safeguard the quality of each article we publish.
Find out more
MINI REVIEW article
Front. Physiol., 09 January 2024
Sec. Clinical and Translational Physiology
Volume 14 - 2023 | https://doi.org/10.3389/fphys.2023.1344885
This article is part of the Research TopicFrontiers in The Midlands Society of Physiological Sciences (2023-2024)View all 10 articles
Stem/progenitor cells have been widely evaluated as a promising therapeutic option for heart failure (HF). Numerous clinical trials with stem/progenitor cell-based therapy (SCT) for HF have demonstrated encouraging results, but not without limitations or discrepancies. Recent technological advancements in multiomics, bioinformatics, precision medicine, artificial intelligence (AI), and machine learning (ML) provide new approaches and insights for stem cell research and therapeutic development. Integration of these new technologies into stem/progenitor cell therapy for HF may help address: 1) the technical challenges to obtain reliable and high-quality therapeutic precursor cells, 2) the discrepancies between preclinical and clinical studies, and 3) the personalized selection of optimal therapeutic cell types/populations for individual patients in the context of precision medicine. This review summarizes the current status of SCT for HF in clinics and provides new perspectives on the development of computation-aided SCT in the era of precision medicine and AI/ML.
Heart failure (HF) typically arises from prolonged cardiomyopathy, a chronic and progressive pathological condition characterized by weakening, loss, and/or stiffening of the heart muscle (i.e., myocardium) (Dassanayaka and Jones, 2015). Without proper intervention, cumulative reductions in the cardiac capacity to pump blood likely lead to HF or even death. Unfortunately, HF is irreversible and incurable because human hearts do not have sufficient innate regenerative capacity to restore severe myocardial damage (Uygur and Lee Richard, 2016). HF has become a major global healthcare burden that progressively deteriorates the physiological capability of the affected population and significantly impacts their quality of life (Savarese et al., 2022). In the United States alone, HF affects around 2.5% and 1.7% of all men and women, respectively (Tsao et al., 2022). Importantly, the overall age-adjusted mortality rate for HF has notably increased from 2.36 to 3.16 per 100,000 people over the recent two decades (1999–2019) (Jain et al., 2022).
The current medical regimen for clinical Stage C symptomatic HF includes a combination of vasodilators, beta blockers, sodium-glucose cotransporter-2 inhibitors, mineralocorticoid receptor antagonists, and diuretics (Heidenreich et al., 2022). Regardless of the recommended medical treatment, about 5% of HF patients develop Stage D HF or end-stage heart disease that requires either heart transplantation or mechanical support with a left ventricular (LV) assist device (LVAD) (Costanzo et al., 2008). However, these advanced therapies for end-stage HF have their individual limitations. For example, there is a constant shortage of matching hearts for transplantation as well as a higher incidence of sudden cardiac death in heart transplant recipients compared to the general population (Colvin et al., 2022; Bonnet et al., 2023). Patients with LVAD are at an increased risk for thromboembolic complications, bleeding, driveline infection, and right ventricular failure (Chaudhry et al., 2022). Thus, there is an unmet need for alternative medical approaches that fundamentally stop or revert the progression of HF pathologies as well as biologically enable the preservation and/or regrowth of functional myocardium.
Stem cells are precursor cells that have the ability to self-renew and differentiate into functionally mature, specialized cells in various human tissues (i.e., pluripotent or multipotent) (Evans and Kaufman, 1981; Pittenger et al., 2019). Numerous efforts have been poured into stem cell research over the last two decades, resulting in abundant laboratory discoveries and translational applications of distinct human stem/progenitor cell types: embryonic stem cells (ESCs), induced pluripotent stem cells (iPSCs), lineage-restricted or tissue-specific stem/progenitor cells (e.g., hematopoietic stem cells, skeletal muscle satellite cells, and intestinal stem cells) (Martello and Smith, 2014; Pizzute et al., 2015; Moradi et al., 2019), and adult mesodermal multipotent precursor cells (e.g., mesenchymal stem/stromal cells) (Pittenger et al., 2019). Many human clinical trials using stem cell-based regenerative therapy for treating HF have thus arisen from promising basic stem cell research and demonstrated encouraging results (Table 1). (Hare et al., 2012; Perin et al., 2012; Heldman et al., 2014; Hare et al., 2017; Teerlink et al., 2017) In this review, we will summarize the current status of stem/progenitor cell therapy for HF, persistent challenges and possible solutions, as well as the future perspectives of stem cell-based cardiac regenerative medicine in the era of precision medicine and artificial intelligence (AI) (Figure 1).
TABLE 1. Summary of recent clinical trials with stem cell therapy for heart failure. This table summarizes the key parameters and findings of major human trials with stem/progenitor cell therapy for heart failure since 2015.
FIGURE 1. Next-generation stem/progenitor cell therapy for heart failure. To design next-generation personalized stem cell treatment for HF that ensures sustainable functional and structural recovery with minimal side effects, it is essential to integrate new components, including AI/ML, bioinformatics, and precision medicine, into stem cell research and therapeutic development. The emerging streamlined high-throughput testing platforms, such as organoid and organ-on-a-chip disease models, may greatly shorten the preclinical development phase and accelerate the progress of human trials. The Figure was partly generated using Servier Medical Art, provided by Servier, licensed under a Creative Commons Attribution 3.0 unported license.
In 2001, bone marrow-derived stem cells (BMSCs) were first transplanted into animal models of ischemic cardiac injury where the donor cells were shown to produce de novo myocardial and vascular structures in the peri-infarcted regions of the myocardium (Jackson et al., 2001; Orlic et al., 2001). The observed benefits were largely attributed to the paracrine release of tissue trophic factors by the donor cells, for example, VEGF and HGF promoting angiogenesis and cardiomyocyte (CM) survival, respectively (Gnecchi et al., 2005; Mabotuwana et al., 2022).
Human phase 2 clinical trials, such as FOCUS CCTRN and TAC-HFT, were conducted in patients with ischemic cardiomyopathy (ICM) who received multiple transendocardial injections of bone marrow mononuclear cells (BMMCs) in the infarcted territory. However, the results failed to demonstrate any significant improvement in LV chamber size, ejection fraction (EF), or quality of life (Perin et al., 2012; Heldman et al., 2014). Similarly, the REGENERATE AMI trial studied the impact of intracoronary infusion of autologous BMMCs in patients with ICM (Choudry et al., 2016). Despite the encouraging results at the 1-year follow-up that showed significant decreases in the infarct size and improved myocardial salvage indices in the intervention group, the 5-year follow-up did not exhibit improved clinical outcomes, suggesting short-term benefits of intracoronary BMMC infusion (Mathur et al., 2022). Interestingly, analysis of pre-transplant bone marrow (BM) samples of patients who responded to autologous BMMC therapy in the FOCUS CCTRN trial showed a higher frequency of CXCR4+ and B cells and fewer monocytes/macrophages and endothelial colony-forming cells in their BM compared to non-responders (Taylor et al., 2016). Therefore, the presence of certain subset(s) of BM progenitor and/or immune cell populations may indicate the potency of donor cells for autologous BMMC therapy (Taylor et al., 2016). The CardiAMP trial utilized the abovementioned concept and screened their subject’s BM cell potency by flow cytometry prior to the enrollment (Johnston et al., 2018; Raval et al., 2021). Subjects with ICM and favorable BM cellular composition were selected for the trial and underwent BM aspiration, followed by an enrichment process to separate the nucleated cell fraction from the plasma phase using a density-tuned dual buoy column; the enriched BM aspirate was then injected into the infarcted myocardium (Raval et al., 2021). The 12-month follow-up data on 10 patients reported significant improvement in 6-min walk distances and trends towards improved NYHA class, LVEF, and quality of life (Raval et al., 2021).
Mesenchymal stem cells (MSCs) are allogeneic STRO-1/STRO-3+ cells, a subpopulation of stromal cells that express CD73, CD90, and CD105 and can be extracted from BM, adipose, and other tissues (Simmons and Torok-Storb, 1991; Haynesworth et al., 1992; Zuk et al., 2001; Karantalis and Hare, 2015). MSCs are adult multipotent precursor cells with great potential for cardiac repair since they can be easily isolated from autologous sources and rapidly expanded ex vivo (Pittenger et al., 1999; Halvorsen et al., 2000; Miura et al., 2003; Dominici et al., 2006; Chen et al., 2015; Melo et al., 2017). MSCs have been shown to improve cardiac function in multiple preclinical animal models of cardiac injury (Amado et al., 2005; Alfaro et al., 2008; Qi et al., 2008; Chen et al., 2013). Their primary mechanism of action for cardiac repair is paracrine secretion of multiplex tissue trophic factors that stimulate cellular repair and regeneration via angiogenesis, endothelization, anti-inflammation, and anti-fibrosis (Kocher et al., 2001; Chen et al., 2009; Abdalmula et al., 2017). The direct differentiation of MSCs into desired cardiac cell types, if any, did not appear to contribute significantly to the functional recovery observed in prior studies (van der Spoel TI. et al., 2011a; Guo et al., 2020).
The MSC-HF trial reported that ICM patients treated with multiple intramyocardial injections of autologous BM-derived MSCs exhibit progressive improvement in LV end-systolic volume (LVESV), EF, and myocardial mass 12 months after their initial treatment, even reducing hospitalization for angina in the MSC-treated group after 4 years (Mathiasen et al., 2020). On 1-year follow-up, the DREAM-HF trial demonstrated that single-dose transendocardial injection of allogenic BM-derived MSCs improved LVEF, LVESV, LV end-diastolic volume (LVEDV) of treated HF patients, with 12% reduction in MI or stroke risk in patients with elevated high-sensitivity CRP (≥2 mg/L) (Perin et al., 2023). These results suggest that MSC treatment can improve clinical outcomes in HF patients for up to several years, especially for those with systemic inflammation.
In contrast, human clinical trials using adipose-derived MSCs for HF treatment failed to show any significant beneficial outcomes (Qayyum et al., 2023a; Qayyum et al., 2023b). Yau et al. reported that intramyocardially injecting allogenic BM-derived MSCs during LVAD implantation did not improve the successful weaning from LVAD, 1-year mortality, or the rate of serious adverse events (Yau et al., 2019). Currently, the STEMVAD trial (NCT03925324) is evaluating the safety and efficacy of three serial doses of allogenic MSCs by intravenous infusions in patients with end-stage HF requiring LVAD. The results of this study will help clarify the utility of MSC therapy in patients with end-stage HF.
Cardiosphere-derived cells (CDCs) are characterized by their ability to separate from cardiac tissues and form spheroids in suspension cultures (Messina et al., 2004). They can function as adult stem/progenitor cells and have been shown to differentiate into myocytes and vascular cells in SCID beige mice (Messina et al., 2004). CDCs mainly contribute to cardiac repair by releasing paracrine factors and exosomes which inhibit cellular apoptosis and promote angiogenesis and CM proliferation (Chimenti et al., 2010; Ibrahim et al., 2014). The ALLSTAR trial evaluated the safety and efficacy of intracoronary delivery of allogenic CDCs in ICM patients with >15% scar burden (Makkar et al., 2020). At the 6-month follow-up, the intervention group showed significant reductions in LVESV, LVEDV, N-terminal pro-B-type natriuretic peptide (NT-proBNP) levels, and decreased segmental circumferential strain with MRI, but no improvement in their LV scar size, suggesting that CDCs could functionally benefit such patients but are not anti-fibrotic (Ostovaneh et al., 2021).
In 2006, Yamanaka and colleagues first described a cocktail of four transcription factors (Oct3/4, Sox2, c-Myc, and Klf4) capable of artificially reprogramming mouse embryonic cells and adult fibroblasts into iPSCs that exhibit the self-renewability and pluripotency similar to ESCs (Takahashi and Yamanaka, 2006). iPSCs possess multiple translational advantages over ESCs: 1) no ethical concerns regarding the cellular origin; (Zheng, 2016); 2) autologous immunocompatible cell sources (if applicable), such as patient’s own fibroblasts, obviating the need for immunosuppression; (Mandai et al., 2017; Schweitzer et al., 2020); 3) direct reprogramming approaches available for differentiating into desired tissue-specific cell types without going through the pluripotent stage, for example, direct reprogramming of human fibroblasts into CMs. (Qian et al., 2012). Cardiomyogenesis from iPSCs has been attempted previously (Yang et al., 2017; Wu et al., 2023); however, iPSC-derived CMs (iPSC-CMs) largely expressed fetal phenotypes and failed to efficiently function as adult CMs (Liao et al., 2021), limiting their clinical applicability. Recently, progress has been made to enhance the maturity of iPSC-CMs (Li et al., 2022; Hsueh et al., 2023). Besides, viral vectors used to reprogram fibroblasts to iPSCs may have the potential to cause cancer; (Okita et al., 2007) alternative non-viral delivery systems to induce iPSCs are under investigation, for example, the targeted nanoparticles (Anokye-Danso et al., 2011; Ye et al., 2016; Wang et al., 2020). iPSCs have the potential for clinical cell therapy in HF patients, and currently, two phase 1 trials are ongoing to assess the safety and efficacy of human allogenic iPSC-CMs in patients with ICM (ClinicalTrials.gov Identifier: NCT04945018 and NCT04696328).
Exosomes are extracellular vesicles that carry various proteins, lipids, and/or RNAs and play a major role in intercellular communications (Rezaie et al., 2022). Since the paracrine effect is an essential mechanism for stem/progenitor cell-mediated cardiac repair, exosomes derived from those cells that contain secretory trophic factors (e.g., pro-angiogenic and pro-survival cytokines) may constitute an alternative therapeutic approach to direct cell transplantation (Bolli et al., 2021). For instance, exosomes derived from human iPSCs had proliferative and protective effects on cardiac mesenchymal stromal cells, impacting their transcriptomic and proteomic profiles. (Bobis-Wozowicz et al., 2015). CDC-derived exosomes delivered via intramyocardial injections were shown to improve cardiac function and decrease scar size in porcine MI models (Gallet et al., 2017). A meta-analysis of ten studies using preclinical animal models of MI revealed that exosomal therapy had the potential to reduce cellular apoptosis and autophagy as well as improve cardiac function, fibrogenesis, and inflammatory response (Zheng et al., 2022).
Potential mechanisms of action of exosomal therapy in ischemic hearts include: 1) protection against myocardial reperfusion injury by reducing oxidative stress through inhibition of caspase 3/7 activation and delivery of cardioprotective microRNAs (miRs) such as miR-21 and miR-210; (Wang et al., 2015); 2) enhancement of intracellular calcium homeostasis and cardiomyocyte contraction by rescuing the expression and function of reticulum Ca2+ ATPase 2a (SERCA-2a) and ryanodine receptor 2 (RyR-2); (Li et al., 2023); and 3) improvement of cellular energy metabolism and myocardial bioenergetics without increasing the risk of arrhythmia (Gao et al., 2020). Intriguingly, besides modulating immune responses and inflammation, immune cell-derived exosomes facilitate crosstalk between immune cells and myocardial cells, which sustains ventricular function and promotes cardiac repair post-MI (Wen et al., 2021).
The use of exosomal therapy in HF patients is still under investigation, (Marbán, 2018; Duong et al., 2023), and current challenges for clinical applications include exosomal delivery, tissue targeting, and immunogenicity (Balbi and Vassalli, 2020). Moreover, exosomes may possibly carry inherent limitations or defects of their cellular origins that could impact their therapeutic efficacy (Riva et al., 2019; Andreeva et al., 2021). Thus, selecting appropriate, healthy stem/progenitor cell sources from which beneficial exosomes can be efficiently extracted is key to improving exosome-based HF therapy.
Human trials involving stem cell therapy often fail to replicate the remarkable successes in animal models of cardiac injury (Table 1). (Rheault-Henry et al., 2021; Bolli et al., 2022; Bolli and Tang, 2022) This could be attributed to multiple reasons: 1) rodent hearts may not accurately mimic the pathophysiology of human HF because they differ from human hearts in terms of size, intrinsic heart rate, (Wessels and Sedmera, 2003), and epigenomic and transcriptomic profiles; (Lin et al., 2014); 2) a number of confounding factors that can be controlled in a laboratory experiment may not be adequately controlled in a human clinical trial, leading to differences in observed outcomes (e.g., diet and genetic background); (Hasenfuss, 1998; van der Spoel et al., 2011a); 3) inconsistent results in clinical trials may be due to the variability in study protocols between different research groups/institutions in terms of donor cell types and sources, treatment dose and duration, routes of administration, and timing of stem cell therapy. (Golpanian et al., 2015).
Besides, isolating specific stem/progenitor cells out of their native niche environment could disrupt important cell-to-cell and/or microenvironmental signaling, which may lead to suboptimal therapeutic potency including reduced cell proliferation, survival, differentiation, and/or paracrine function. (Kuchina et al., 2011). Furthermore, the cardiac disease cascade in humans is complex and consists of a dynamic process of progressive tissue ischemia, hypoxia, inflammation, and/or myocardial fibrosis, making the host environment harsh for transplanted cells to survive. Another issue is inadequate cell retention and reduced cell survival after administration because only ∼11% of the delivered cells are retained in the myocardium, decreasing the overall efficiency of cell therapy (Hou et al., 2005). Currently, the process of stem/progenitor cell homing to areas of myocardial damage is not fully understood, and strategies to improve targeted cardiac delivery are under investigation (Liesveld et al., 2020).
Also, there are variabilities among patients in terms of comorbidities, risk factors, lifestyle, and genomic differences; presently, it is not clear which type of individuals will benefit most from stem cell therapy (Patel et al., 2010; Gambini et al., 2012). Additionally, the majority of human trials used LVEF, LVESV, and LVEDV as surrogates for cardiac recovery; alternative endpoints may be needed to assess the efficacy of stem/progenitor cells since multiple clinical studies have reported improvement in subjects’ quality of life and exercise tolerance without any significant increases in LVEF (Bolli et al., 2022). Thus, it may be essential to investigate alternative endpoint surrogates rather than solely relying on notable improvement in LVEF as the marker for therapeutic success (Borow et al., 2019).
There remain other challenges to solve, including 1) designing optimal cell banking strategies to maintain the therapeutic quality of donor stem/progenitor cells; 2) establishing appropriate high-throughput experimental protocols or computation algorithms to select or predict stem/progenitor cells ideal for treating a particular HF stage or pathology, respectively; and 3) building more non-invasive tools to measure how transplanted precursor cells function within the human subjects. Addressing these challenges may help improve the efficacy of stem cell therapy in human trials.
Precision medicine is the anticipated future of medicine where therapy will be tailored according to the patient’s genetic composition, environment, lifestyle, and risk factors (Ashley, 2016; Delpierre and Lefèvre, 2023). Stem cells can be used for a number of applications in precision medicine: 1) stem cell-derived CMs can be used to understand or simulate the pathophysiology of patient-specific cardiac conditions; (Musunuru et al., 2018) 2) cardiac diseases caused by genetic mutations can be replicated in patient-derived or engineered cell models with the assistance of the iPSC technology or genome editing tools, respectively; (Musunuru et al., 2018) 3) stem cell-derived cardiac cellular models can be used to test the efficacy and safety of personalized medication for individual patients; (Chen et al., 2016) and 4) autologous stem cell-derived cardiac cells may be used as a personalized therapeutic tool (Musunuru et al., 2018; Lightner and Chan, 2021).
Alternatively, cardiac organoid models derived from stem cells have been used to substitute animal and human subjects for the initial testing of the safety and/or efficacy in drug development, reducing animal and human morbidity and mortality (Azar et al., 2021). Thus, utilizing stem cells in precision medicine will not only improve our understanding of acquired cardiac disease (e.g., ICM and HF), (Bolli et al., 2022) inherent conditions (e.g., familial cardiomyopathies), (Jiang et al., 2021) and congenital heart defects (e.g., hypoplastic left heart syndrome, Ebstein anomaly, Fontan circulation with right ventricular dysfunction), (Tsilimigras et al., 2017) but also has the potential to be used as adjuvant treatment to current medical or surgical therapies.
Furthermore, large clinical datasets that comprise patient histories and characteristics, body fluid compositions, diagnostic results, tissue pathologies, imaging studies, and/or treatment effects may be used to identify, classify, or even predict the distinct signatures or behaviors of genome, epigenome, transcriptome, proteome, and/or phenome associated with a particular cardiac pathology in individual patients, and vice versa (Attia et al., 2019; Qiu et al., 2020; Segar et al., 2020; Liu et al., 2022). Also, many biological samples of patient-specific stem cells used in clinical trials are currently stored in biobanks (Musunuru et al., 2018; Annaratone et al., 2021). Combining the big data of multiomics with the cellular background and clinical information may facilitate a personalized multi-level analysis (Shi and Xu, 2019; Hu et al., 2020). Such comprehensive personalized analysis may improve our understanding of how stem cells behave and/or interact with other cell types under specific pathological conditions or disease stages (e.g., terminal-stage HF), ultimately aiding in the design of precision stem cell therapy for personalized medicine (Figure 1).
The fields of AI and machine learning (ML) are rapidly expanding and contributing to various medical applications, including medical imaging, personalized medicine, and robotic-assisted surgeries (Krajcer, 2022; Haug and Drazen, 2023). AI-driven decision-making is exemplified in scenarios where algorithms can process environmental and biological inputs, such as changes in the culture media, intercellular signals, or cellular behaviors, and respond accordingly based on predefined parameters (Adlung et al., 2021). For instance, AI may autonomously detect and sustain predetermined cellular phenotypes by adjusting the conditions in human stem/progenitor cell cultures (e.g., infusing specific cytokines to stimulate cell growth or adding bicarbonate to maintain consistent pH levels), keeping the culture quality and streamlining routine wet-lab tasks (Capponi and Daniels, 2023).
AI’s capability to analyze large preclinical and clinical datasets from biobanks, research data depositories, public health databases, and healthcare systems has immense implications for stem cell therapeutics in the context of precision medicine. AI/ML can be leveraged to identify common genomic traits, individual genetic polymorphism, disease-associated mutations, morphological patterns, and/or cellular functions in a personalized manner (Capponi and Daniels, 2023). This transdisciplinary knowledge helps: 1) determine the developmental stage and maturation of stem cells, (Guan et al., 2021; Kim et al., 2022) 2) assess their regenerative potentials and/or limitations, (Fischbacher et al., 2021), and 3) predict their therapeutic efficacy and/or side-effects in individual subjects. (Mota et al., 2021). For example, ML algorithms were used to identify biomarkers for predicting positive patient responses to BMSC therapy, (Steinhoff et al., 2017), characterize CMs non-invasively using video microscopy and image analysis, (Maddah and Loewke, 2014), analyze the effects of drugs on the calcium signals of iPSC-CMs, (Juhola et al., 2021), identify cell lines with/without genetic defects using cellular images, (Kim et al., 2023), and identify neural stem cell differentiation. (Zhu et al., 2021).
Moreover, by analyzing the unique DNA methylation profiles, investigators devised a linear classification learning model to discern iPSCs, ESCs, somatic cells, and embryonal carcinoma cells, achieving 94.23% accuracy. (Nishino et al., 2021). Another group utilized convolutional neural networks (CNNs) to effectively differentiate pluripotent cells from initial differentiating cells. (Waisman et al., 2019). The training of the CNN model involved the use of light microscopic images of PSCs captured at different intervals after the induction process, including mouse-embryonic cells being induced to epiblast-like cells. (Waisman et al., 2019). Notably, the results demonstrated CNN’s remarkable capability to distinguish between differentiated and undifferentiated cells with 99% accuracy.
Importantly, AI can leverage information from separate studies, extensive datasets, and stem cell biobanks to create models that predict the outcomes of stem cell therapy for specific disease states. These models can potentially be applied to enhance stem cell proliferation, optimize their functions in the host environment, and/or predict the most effective population(s) for individuals with specific phenotypes of cardiomyopathy (Capponi and Daniels, 2023). Thus, integrating AI/ML into stem cell research holds great promise for advancing precursor cell-based therapy for HF by: 1) facilitating our understanding of stem cell biology within specific cardiac disease contexts at a systems level; 2) improving the good manufacturing practice for clinical-grade cellular products; and 3) establishing personalized therapeutic prediction models for individual patients (Figure 1).
A considerable number of recent clinical trials in stem cell therapy for HF have demonstrated its promise and substantially increased our understanding of the behaviors and working mechanisms of stem/progenitor cells in patients (Table 1). (Hamshere et al., 2015; Martino et al., 2015; Perin et al., 2015; Choudry et al., 2016; Noiseux et al., 2016; Patel et al., 2016; Bartolucci et al., 2017; Butler et al., 2017; Choudhury et al., 2017; Florea et al., 2017; Gwizdala et al., 2017; Hare et al., 2017; Steinhoff et al., 2017; Teerlink et al., 2017; Xiao et al., 2017; Bassetti et al., 2018; Vrtovec et al., 2018; Yau et al., 2019; Bolli et al., 2020; He et al., 2020; Makkar et al., 2020; Mathiasen et al., 2020; Ulus et al., 2020; Bolli et al., 2021; Qayyum et al., 2023a; Qayyum et al., 2023b; Perin et al., 2023) In the next phase of clinical stem cell research, it is critical to address the outcome discrepancy between preclinical and clinical studies and expand the scope of stem cell-based therapy to other forms of cardiomyopathy, such as chemotherapy- or arrhythmia-induced cardiomyopathy. Exploiting the power of AI/ML and computational tools will facilitate our understanding of the benefits and limitations of stem cell therapy and provide a systems perspective for properly applying stem cell therapeutics in the context of precision and personalized medicine.
MC: Investigation, Resources, Software, Visualization, Writing–original draft, Writing–review and editing. JZ: Investigation, Writing–original draft, Writing–review and editing. RR: Conceptualization, Investigation, Writing–original draft, Writing–review and editing. WC: Conceptualization, Funding acquisition, Investigation, Project administration, Resources, Supervision, Visualization, Writing–original draft, Writing–review and editing.
The author(s) declare financial support was received for the research, authorship, and/or publication of this article. This work was supported by the U.S. Department of Defense (W81XWH2110089 to WC), the American Heart Association Career Development Award (855260 to WC), and the University of South Dakota Sanford School of Medicine (Startup Fund to WC).
The authors declare that the research was conducted in the absence of any commercial or financial relationships that could be construed as a potential conflict of interest.
All claims expressed in this article are solely those of the authors and do not necessarily represent those of their affiliated organizations, or those of the publisher, the editors and the reviewers. Any product that may be evaluated in this article, or claim that may be made by its manufacturer, is not guaranteed or endorsed by the publisher.
Abdalmula A., Dooley L. M., Kaufman C., Washington E. A., House J. V., Blacklaws B. A., et al. (2017). Immunoselected STRO-3+ mesenchymal precursor cells reduce inflammation and improve clinical outcomes in a large animal model of monoarthritis. Stem Cell. Res. Ther. 8 (1), 22. doi:10.1186/s13287-016-0460-7
Adlung L., Cohen Y., Mor U., Elinav E. (2021). Machine learning in clinical decision making. Med 2 (6), 642–665. doi:10.1016/j.medj.2021.04.006
Alfaro M. P., Pagni M., Vincent A., Atkinson J., Hill M. F., Cates J., et al. (2008). The Wnt modulator sFRP2 enhances mesenchymal stem cell engraftment, granulation tissue formation and myocardial repair. Proc. Natl. Acad. Sci. U. S. A. 105 (47), 18366–18371. doi:10.1073/pnas.0803437105
Amado L. C., Saliaris A. P., Schuleri K. H., St John M., Xie J. S., Cattaneo S., et al. (2005). Cardiac repair with intramyocardial injection of allogeneic mesenchymal stem cells after myocardial infarction. Proc. Natl. Acad. Sci. U. S. A. 102 (32), 11474–11479. doi:10.1073/pnas.0504388102
Andreeva O. E., Shchegolev Y. Y., Scherbakov A. M., Mikhaevich E. I., Sorokin D. V., Gudkova M. V., et al. (2021). Secretion of mutant DNA and mRNA by the exosomes of breast cancer cells. Molecules 26 (9), 2499. doi:10.3390/molecules26092499
Annaratone L., De Palma G., Bonizzi G., Sapino A., Botti G., Berrino E., et al. (2021). Basic principles of biobanking: from biological samples to precision medicine for patients. Virchows Arch. 479 (2), 233–246. doi:10.1007/s00428-021-03151-0
Anokye-Danso F., Trivedi C. M., Juhr D., Gupta M., Cui Z., Tian Y., et al. (2011). Highly efficient miRNA-mediated reprogramming of mouse and human somatic cells to pluripotency. Cell. Stem Cell. 8 (4), 376–388. doi:10.1016/j.stem.2011.03.001
Ashley E. A. (2016). Towards precision medicine. Nat. Rev. Genet. 17 (9), 507–522. doi:10.1038/nrg.2016.86
Attia Z. I., Noseworthy P. A., Lopez-Jimenez F., Asirvatham S. J., Deshmukh A. J., Gersh B. J., et al. (2019). An artificial intelligence-enabled ECG algorithm for the identification of patients with atrial fibrillation during sinus rhythm: a retrospective analysis of outcome prediction. Lancet 394 (10201), 861–867. doi:10.1016/S0140-6736(19)31721-0
Azar J., Bahmad H. F., Daher D., Moubarak M. M., Hadadeh O., Monzer A., et al. (2021). The use of stem cell-derived organoids in disease modeling: an update. Int. J. Mol. Sci. 22 (14), 7667. doi:10.3390/ijms22147667
Balbi C., Vassalli G. (2020). Exosomes: beyond stem cells for cardiac protection and repair. STEM CELLS 38 (11), 1387–1399. doi:10.1002/stem.3261
Bartolucci J., Verdugo F. J., González P. L., Larrea R. E., Abarzua E., Goset C., et al. (2017). Safety and efficacy of the intravenous infusion of umbilical cord mesenchymal stem cells in patients with heart failure: a phase 1/2 randomized controlled trial (rimecard trial [randomized clinical trial of intravenous infusion umbilical cord mesenchymal stem cells on cardiopathy]). Circ. Res. 121 (10), 1192–1204. doi:10.1161/CIRCRESAHA.117.310712
Bassetti B., Carbucicchio C., Catto V., Gambini E., Rurali E., Bestetti A., et al. (2018). Linking cell function with perfusion: insights from the transcatheter delivery of bone marrow-derived CD133+ cells in ischemic refractory cardiomyopathy trial (RECARDIO). Stem Cell. Res. Ther. 9 (1), 235. doi:10.1186/s13287-018-0969-z
Bobis-Wozowicz S., Kmiotek K., Sekula M., Kedracka-Krok S., Kamycka E., Adamiak M., et al. (2015). Human induced pluripotent stem cell-derived microvesicles transmit RNAs and proteins to recipient mature heart cells modulating cell fate and behavior. Stem Cells 33 (9), 2748–2761. doi:10.1002/stem.2078
Bolli R., Mitrani R. D., Hare J. M., Pepine C. J., Perin E. C., Willerson J. T., et al. (2021). A Phase II study of autologous mesenchymal stromal cells and c-kit positive cardiac cells, alone or in combination, in patients with ischaemic heart failure: the CCTRN CONCERT-HF trial. Eur. J. Heart Fail 23 (4), 661–674. doi:10.1002/ejhf.2178
Bolli R., Perin E. C., Willerson J. T., Yang P. C., Traverse J. H., Henry T. D., et al. (2020). Allogeneic mesenchymal cell therapy in anthracycline-induced cardiomyopathy heart failure patients: the CCTRN SENECA trial. JACC CardioOncol 2 (4), 581–595. doi:10.1016/j.jaccao.2020.09.001
Bolli R., Solankhi M., Tang X. L., Kahlon A. (2022). Cell therapy in patients with heart failure: a comprehensive review and emerging concepts. Cardiovasc Res. 118 (4), 951–976. doi:10.1093/cvr/cvab135
Bolli R., Tang X. L. (2022). The sad plight of cell therapy for heart failure: causes and consequences. J. Cardiovasc Aging 2, 16. doi:10.20517/jca.2022.02
Bonnet G., Coutance G., Aubert O., Waldmann V., Raynaud M., Asselin A., et al. (2023). Sudden cardiac death after heart transplantation: a population-based study. EP Eur. 25 (5), euad126. doi:10.1093/europace/euad126
Borow K. M., Yaroshinsky A., Greenberg B., Perin E. C. (2019). Phase 3 DREAM-HF trial of mesenchymal precursor cells in chronic heart failure. Circ. Res. 125 (3), 265–281. doi:10.1161/CIRCRESAHA.119.314951
Butler J., Epstein S. E., Greene S. J., Quyyumi A. A., Sikora S., Kim R. J., et al. (2017). Intravenous allogeneic mesenchymal stem cells for nonischemic cardiomyopathy: safety and efficacy results of a phase II-A randomized trial. Circ. Res. 120 (2), 332–340. doi:10.1161/CIRCRESAHA.116.309717
Capponi S., Daniels K. G. (2023). Harnessing the power of artificial intelligence to advance cell therapy. Immunol. Rev. 320, 147–165. n/a(n/a). doi:10.1111/imr.13236
Chaudhry S. P., DeVore A. D., Vidula H., Nassif M., Mudy K., Birati E. Y., et al. (2022). Left ventricular assist devices: a primer for the general cardiologist. J. Am. Heart Assoc. 11 (24), e027251. doi:10.1161/JAHA.122.027251
Chen C.-W., Montelatici E., Crisan M., Corselli M., Huard J., Lazzari L., et al. (2009). Perivascular multi-lineage progenitor cells in human organs: regenerative units, cytokine sources or both? Cytokine and Growth Factor Rev. 20 (5-6), 429–434. doi:10.1016/j.cytogfr.2009.10.014
Chen C.-W., Okada M., Proto J. D., Gao X., Sekiya N., Beckman S. A., et al. (2013). Human pericytes for ischemic heart repair. STEM CELLS 31 (2), 305–316. doi:10.1002/stem.1285
Chen I. Y., Matsa E., Wu J. C. (2016). Induced pluripotent stem cells: at the heart of cardiovascular precision medicine. Nat. Rev. Cardiol. 13 (6), 333–349. doi:10.1038/nrcardio.2016.36
Chen W. C. W., Baily J. E., Corselli M., Díaz M. E., Sun B., Xiang G., et al. (2015). Human myocardial pericytes: multipotent mesodermal precursors exhibiting cardiac specificity. STEM CELLS 33 (2), 557–573. doi:10.1002/stem.1868
Chimenti I., Smith R. R., Li T. S., Gerstenblith G., Messina E., Giacomello A., et al. (2010). Relative roles of direct regeneration versus paracrine effects of human cardiosphere-derived cells transplanted into infarcted mice. Circ. Res. 106 (5), 971–980. doi:10.1161/CIRCRESAHA.109.210682
Choudhury T., Mozid A., Hamshere S., Yeo C., Pellaton C., Arnous S., et al. (2017). An exploratory randomized control study of combination cytokine and adult autologous bone marrow progenitor cell administration in patients with ischaemic cardiomyopathy: the REGENERATE-IHD clinical trial. Eur. J. Heart Fail 19 (1), 138–147. doi:10.1002/ejhf.676
Choudry F., Hamshere S., Saunders N., Veerapen J., Bavnbek K., Knight C., et al. (2016). A randomized double-blind control study of early intra-coronary autologous bone marrow cell infusion in acute myocardial infarction: the REGENERATE-AMI clinical trial. Eur. Heart J. 37 (3), 256–263. doi:10.1093/eurheartj/ehv493
Colvin M., Smith J. M., Ahn Y., Skeans M. A., Messick E., Bradbrook K., et al. (2022). OPTN/SRTR 2020 annual data report: heart. Am. J. Transpl. 22 (Suppl. 2), 350–437. doi:10.1111/ajt.16977
Costanzo M. R., Mills R. M., Wynne J. (2008). Characteristics of "stage D" heart failure: insights from the acute decompensated heart failure national registry longitudinal module (ADHERE LM). Am. Heart J. 155 (2), 339–347. doi:10.1016/j.ahj.2007.10.020
Dassanayaka S., Jones S. P. (2015). Recent developments in heart failure. Circulation Res. 117 (7), e58–e63. doi:10.1161/CIRCRESAHA.115.305765
Delpierre C., Lefèvre T. (2023). Precision and personalized medicine: what their current definition says and silences about the model of health they promote. Implication for the development of personalized health. Front. Sociol. 8, 1112159. doi:10.3389/fsoc.2023.1112159
Dominici M., Le Blanc K., Mueller I., Slaper-Cortenbach I., Marini F., Krause D., et al. (2006). Minimal criteria for defining multipotent mesenchymal stromal cells. The International Society for Cellular Therapy position statement. Cytotherapy 8 (4), 315–317. doi:10.1080/14653240600855905
Duong A., Parmar G., Kirkham A. M., Burger D., Allan D. S. (2023). Registered clinical trials investigating treatment with cell-derived extracellular vesicles: a scoping review. Cytotherapy 25 (9), 939–945. doi:10.1016/j.jcyt.2023.04.007
Evans M. J., Kaufman M. H. (1981). Establishment in culture of pluripotential cells from mouse embryos. Nature 292 (5819), 154–156. doi:10.1038/292154a0
Fischbacher B., Hedaya S., Hartley B. J., Wang Z., Lallos G., Hutson D., et al. (2021). Modular deep learning enables automated identification of monoclonal cell lines. Nat. Mach. Intell. 3 (7), 632–640. doi:10.1038/s42256-021-00354-7
Florea V., Rieger A. C., DiFede D. L., El-Khorazaty J., Natsumeda M., Banerjee M. N., et al. (2017). Dose comparison study of allogeneic mesenchymal stem cells in patients with ischemic cardiomyopathy (the TRIDENT study). Circ. Res. 121 (11), 1279–1290. doi:10.1161/CIRCRESAHA.117.311827
Gallet R., Dawkins J., Valle J., Simsolo E., de Couto G., Middleton R., et al. (2017). Exosomes secreted by cardiosphere-derived cells reduce scarring, attenuate adverse remodelling, and improve function in acute and chronic porcine myocardial infarction. Eur. Heart J. 38 (3), 201–211. doi:10.1093/eurheartj/ehw240
Gambini E., Pesce M., Persico L., Bassetti B., Gambini A., Alamanni F., et al. (2012). Patient profile modulates cardiac c-kit(+) progenitor cell availability and amplification potential. Transl. Res. 160 (5), 363–373. doi:10.1016/j.trsl.2012.05.009
Gao L., Wang L., Wei Y., Krishnamurthy P., Walcott G. P., Menasché P., et al. (2020). Exosomes secreted by hiPSC-derived cardiac cells improve recovery from myocardial infarction in swine. Sci. Transl. Med. 12 (561), eaay1318. doi:10.1126/scitranslmed.aay1318
Gnecchi M., He H., Liang O. D., Melo L. G., Morello F., Mu H., et al. (2005). Paracrine action accounts for marked protection of ischemic heart by Akt-modified mesenchymal stem cells. Nat. Med. 11 (4), 367–368. doi:10.1038/nm0405-367
Golpanian S., Schulman I. H., Ebert R. F., Heldman A. W., DiFede D. L., Yang P. C., et al. (2015). Concise review: review and perspective of cell dosage and routes of administration from preclinical and clinical studies of stem cell therapy for heart disease. Stem Cells Transl. Med. 5 (2), 186–191. doi:10.5966/sctm.2015-0101
Guan B. X., Bhanu B., Theagarajan R., Liu H., Talbot P., Weng N. (2021). Human embryonic stem cell classification: random network with autoencoded feature extractor. J. Biomed. Opt. 26 (5), 052913. doi:10.1117/1.JBO.26.5.052913
Guo Y., Yu Y., Hu S., Chen Y., Shen Z. (2020). The therapeutic potential of mesenchymal stem cells for cardiovascular diseases. Cell. Death Dis. 11 (5), 349. doi:10.1038/s41419-020-2542-9
Gwizdala A., Rozwadowska N., Kolanowski T. J., Malcher A., Cieplucha A., Perek B., et al. (2017). Safety, feasibility and effectiveness of first in-human administration of muscle-derived stem/progenitor cells modified with connexin-43 gene for treatment of advanced chronic heart failure. Eur. J. Heart Fail 19 (1), 148–157. doi:10.1002/ejhf.700
Halvorsen Y. C., Wilkison W. O., Gimble J. M. (2000). Adipose-derived stromal cells--their utility and potential in bone formation. Int. J. Obes. Relat. Metab. Disord. 24 (Suppl. 4), S41–S44. doi:10.1038/sj.ijo.0801503
Hamshere S., Arnous S., Choudhury T., Choudry F., Mozid A., Yeo C., et al. (2015). Randomized trial of combination cytokine and adult autologous bone marrow progenitor cell administration in patients with non-ischaemic dilated cardiomyopathy: the REGENERATE-DCM clinical trial. Eur. Heart J. 36 (44), 3061–3069. doi:10.1093/eurheartj/ehv390
Hare J. M., DiFede D. L., Rieger A. C., Florea V., Landin A. M., El-Khorazaty J., et al. (2017). Randomized comparison of allogeneic versus autologous mesenchymal stem cells for nonischemic dilated cardiomyopathy: POSEIDON-DCM trial. J. Am. Coll. Cardiol. 69 (5), 526–537. doi:10.1016/j.jacc.2016.11.009
Hare J. M., Fishman J. E., Gerstenblith G., DiFede Velazquez D. L., Zambrano J. P., Suncion V. Y., et al. (2012). Comparison of allogeneic vs autologous bone marrow–derived mesenchymal stem cells delivered by transendocardial injection in patients with ischemic cardiomyopathy: the POSEIDON randomized trial. Jama 308 (22), 2369–2379. doi:10.1001/jama.2012.25321
Hasenfuss G. (1998). Animal models of human cardiovascular disease, heart failure and hypertrophy. Cardiovasc. Res. 39 (1), 60–76. doi:10.1016/s0008-6363(98)00110-2
Haug C. J., Drazen J. M. (2023). Artificial intelligence and machine learning in clinical medicine, 2023. N. Engl. J. Med. 388 (13), 1201–1208. doi:10.1056/NEJMra2302038
Haynesworth S. E., Goshima J., Goldberg V. M., Caplan A. I. (1992). Characterization of cells with osteogenic potential from human marrow. Bone 13 (1), 81–88. doi:10.1016/8756-3282(92)90364-3
He X., Wang Q., Zhao Y., Zhang H., Wang B., Pan J., et al. (2020). Effect of intramyocardial grafting collagen scaffold with mesenchymal stromal cells in patients with chronic ischemic heart disease: a randomized clinical trial. JAMA Netw. Open 3 (9), e2016236. doi:10.1001/jamanetworkopen.2020.16236
Heidenreich P. A., Bozkurt B., Aguilar D., Allen L. A., Byun J. J., Colvin M. M., et al. (2022). 2022 AHA/ACC/HFSA guideline for the management of heart failure: a report of the American college of cardiology/American heart association joint committee on clinical practice guidelines. Circulation 145 (18), e895–e1032. doi:10.1161/CIR.0000000000001063
Heldman A. W., DiFede D. L., Fishman J. E., Zambrano J. P., Trachtenberg B. H., Karantalis V., et al. (2014). Transendocardial mesenchymal stem cells and mononuclear bone marrow cells for ischemic cardiomyopathy: the TAC-HFT randomized trial. Jama 311 (1), 62–73. doi:10.1001/jama.2013.282909
Hou D., Youssef E. A., Brinton T. J., Zhang P., Rogers P., Price E. T., et al. (2005). Radiolabeled cell distribution after intramyocardial, intracoronary, and interstitial retrograde coronary venous delivery: implications for current clinical trials. Circulation 112 (9 Suppl. l), I150–I156. doi:10.1161/CIRCULATIONAHA.104.526749
Hsueh Y.-C., Pratt R. E., Dzau V. J., Hodgkinson C. P. (2023). Novel method of differentiating human induced pluripotent stem cells to mature cardiomyocytes via Sfrp2. Sci. Rep. 13 (1), 3920. doi:10.1038/s41598-023-31144-3
Hu J., Xu J., Yu M., Gao Y., Liu R., Zhou H., et al. (2020). An integrated prognosis model of pharmacogenomic gene signature and clinical information for diffuse large B-cell lymphoma patients following CHOP-like chemotherapy. J. Transl. Med. 18 (1), 144. doi:10.1186/s12967-020-02311-1
Ibrahim A. G., Cheng K., Marbán E. (2014). Exosomes as critical agents of cardiac regeneration triggered by cell therapy. Stem Cell. Rep. 2 (5), 606–619. doi:10.1016/j.stemcr.2014.04.006
Jackson K. A., Majka S. M., Wang H., Pocius J., Hartley C. J., Majesky M. W., et al. (2001). Regeneration of ischemic cardiac muscle and vascular endothelium by adult stem cells. J. Clin. Investig. 107 (11), 1395–1402. doi:10.1172/JCI12150
Jain V., Minhas A. M. K., Morris A. A., Greene S. J., Pandey A., Khan S. S., et al. (2022). Demographic and regional trends of heart failure–related mortality in young adults in the US, 1999-2019. JAMA Cardiol. 7 (9), 900–904. doi:10.1001/jamacardio.2022.2213
Jiang X., Chen Y., Liu X., Ye L., Yu M., Shen Z., et al. (2021). Uncovering inherited cardiomyopathy with human induced pluripotent stem cells. Front. Cell. Dev. Biol. 9, 672039. doi:10.3389/fcell.2021.672039
Johnston P. V., Duckers H. J., Raval A. N., Cook T. D., Pepine C. J. (2018). Not all stem cells are created equal. Circulation Res. 123 (8), 944–946. doi:10.1161/CIRCRESAHA.118.313425
Juhola M., Penttinen K., Joutsijoki H., Aalto-Setälä K. (2021). Analysis of drug effects on iPSC cardiomyocytes with machine learning. Ann. Biomed. Eng. 49 (1), 129–138. doi:10.1007/s10439-020-02521-0
Karantalis V., Hare J. M. (2015). Use of mesenchymal stem cells for therapy of cardiac disease. Circulation Res. 116 (8), 1413–1430. doi:10.1161/CIRCRESAHA.116.303614
Kim M., Namkung Y., Hyun D., Hong S. (2023). Prediction of stem cell state using cell image-based deep learning. Adv. Intell. Syst. 5 (7), 2300017. doi:10.1002/aisy.202370031
Kim M., Namkung Y., Hyun D., Hong S. (2022). Prediction of stem cell state using cell image-based deep learning. Adv. Intell. Syst. 1, 2300017. doi:10.1002/aisy.202300017
Kocher A. A., Schuster M. D., Szabolcs M. J., Takuma S., Burkhoff D., Wang J., et al. (2001). Neovascularization of ischemic myocardium by human bone-marrow-derived angioblasts prevents cardiomyocyte apoptosis, reduces remodeling and improves cardiac function. Nat. Med. 7 (4), 430–436. doi:10.1038/86498
Krajcer Z. (2022). Artificial intelligence in cardiovascular medicine: historical overview, current status, and future directions. Tex Heart Inst. J. 49 (2), e207527. doi:10.14503/THIJ-20-7527
Kuchina A., Espinar L., Garcia-Ojalvo J., Süel G. M. (2011). Reversible and noisy progression towards a commitment point enables adaptable and reliable cellular decision-making. PLoS Comput. Biol. 7 (11), e1002273. doi:10.1371/journal.pcbi.1002273
Li H., Wang L., Ma T., Liu Z., Gao L. (2023). Exosomes secreted by endothelial cells derived from human induced pluripotent stem cells improve recovery from myocardial infarction in mice. Stem Cell. Res. Ther. 14 (1), 278. doi:10.1186/s13287-023-03462-w
Li L., Wan Z., Wang R., Zhao Y., Ye Y., Yang P., et al. (2022). Generation of high-performance human cardiomyocytes and engineered heart tissues from extended pluripotent stem cells. Cell. Discov. 8 (1), 105. doi:10.1038/s41421-022-00446-7
Liao Y., Zhu L., Wang Y. (2021). Maturation of stem cell-derived cardiomyocytes: foe in translation medicine. Int. J. Stem Cells 14 (4), 366–385. doi:10.15283/ijsc21077
Liesveld J. L., Sharma N., Aljitawi O. S. (2020). Stem cell homing: from physiology to therapeutics. STEM CELLS 38 (10), 1241–1253. doi:10.1002/stem.3242
Lightner A. L., Chan T. (2021). Precision regenerative medicine. Stem Cell. Res. Ther. 12 (1), 39. doi:10.1186/s13287-020-02092-w
Lin S., Lin Y., Nery J. R., Urich M. A., Breschi A., Davis C. A., et al. (2014). Comparison of the transcriptional landscapes between human and mouse tissues. Proc. Natl. Acad. Sci. 111 (48), 17224–17229. doi:10.1073/pnas.1413624111
Liu Z., Lin G., Yan Z., Li L., Wu X., Shi J., et al. (2022). Predictive mutation signature of immunotherapy benefits in NSCLC based on machine learning algorithms. Front. Immunol. 13, 989275. doi:10.3389/fimmu.2022.989275
Mabotuwana N. S., Rech L., Lim J., Hardy S. A., Murtha L. A., Rainer P. P., et al. (2022). Paracrine factors released by stem cells of mesenchymal origin and their effects in cardiovascular disease: a systematic review of pre-clinical studies. Stem Cell. Rev. Rep. 18 (8), 2606–2628. doi:10.1007/s12015-022-10429-6
Maddah M., Loewke K. (2014). Automated, non-invasive characterization of stem cell-derived cardiomyocytes from phase-contrast microscopy. Med. Image Comput. Comput. Assist. Interv. 17 (Pt 1), 57–64. doi:10.1007/978-3-319-10404-1_8
Makkar R. R., Kereiakes D. J., Aguirre F., Kowalchuk G., Chakravarty T., Malliaras K., et al. (2020). Intracoronary ALLogeneic heart STem cells to Achieve myocardial Regeneration (ALLSTAR): a randomized, placebo-controlled, double-blinded trial. Eur. Heart J. 41 (36), 3451–3458. doi:10.1093/eurheartj/ehaa541
Mandai M., Watanabe A., Kurimoto Y., Hirami Y., Morinaga C., Daimon T., et al. (2017). Autologous induced stem-cell-derived retinal cells for macular degeneration. N. Engl. J. Med. 376 (11), 1038–1046. doi:10.1056/NEJMoa1608368
Marbán E. (2018). The secret life of exosomes: what bees can teach us about next-generation therapeutics. J. Am. Coll. Cardiol. 71 (2), 193–200. doi:10.1016/j.jacc.2017.11.013
Martello G., Smith A. (2014). The nature of embryonic stem cells. Annu. Rev. Cell. Dev. Biol. 30, 647–675. doi:10.1146/annurev-cellbio-100913-013116
Martino H., Brofman P., Greco O., Bueno R., Bodanese L., Clausell N., et al. (2015). Multicentre, randomized, double-blind trial of intracoronary autologous mononuclear bone marrow cell injection in non-ischaemic dilated cardiomyopathy (the dilated cardiomyopathy arm of the MiHeart study). Eur. Heart J. NICM2015 36 (42), 2898–2904. doi:10.1093/eurheartj/ehv477
Mathiasen A. B., Qayyum A. A., Jørgensen E., Helqvist S., Kofoed K. F., Haack-Sørensen M., et al. (2020). Bone marrow-derived mesenchymal stromal cell treatment in patients with ischaemic heart failure: final 4-year follow-up of the MSC-HF trial. Eur. J. Heart Fail 22 (5), 884–892. doi:10.1002/ejhf.1700
Mathur A., Sim D. S., Choudry F., Veerapen J., Colicchia M., Turlejski T., et al. (2022). Five-year follow-up of intracoronary autologous cell therapy in acute myocardial infarction: the REGENERATE-AMI trial. Esc. Heart Fail 9 (2), 1152–1159. doi:10.1002/ehf2.13786
Melo F. R., Bressan R. B., Forner S., Martini A. C., Rode M., Delben P. B., et al. (2017). Transplantation of human skin-derived mesenchymal stromal cells improves locomotor recovery after spinal cord injury in rats. Cell. Mol. Neurobiol. 37 (5), 941–947. doi:10.1007/s10571-016-0414-8
Messina E., De Angelis L., Frati G., Morrone S., Chimenti S., Fiordaliso F., et al. (2004). Isolation and expansion of adult cardiac stem cells from human and murine heart. Circ. Res. 95 (9), 911–921. doi:10.1161/01.RES.0000147315.71699.51
Miura M., Gronthos S., Zhao M., Lu B., Fisher L. W., Robey P. G., et al. (2003). SHED: stem cells from human exfoliated deciduous teeth. Proc. Natl. Acad. Sci. U. S. A. 100 (10), 5807–5812. doi:10.1073/pnas.0937635100
Moradi S., Mahdizadeh H., Šarić T., Kim J., Harati J., Shahsavarani H., et al. (2019). Research and therapy with induced pluripotent stem cells (iPSCs): social, legal, and ethical considerations. Stem Cell. Res. Ther. 10 (1), 341. doi:10.1186/s13287-019-1455-y
Mota S. M., Rogers R. E., Haskell A. W., McNeill E. P., Kaunas R., Gregory C. A., et al. (2021). Automated mesenchymal stem cell segmentation and machine learning-based phenotype classification using morphometric and textural analysis. J. Med. Imaging (Bellingham) 8 (1), 014503. doi:10.1117/1.JMI.8.1.014503
Musunuru K., Sheikh F., Gupta R. M., Houser S. R., Maher K. O., Milan D. J., et al. (2018). Induced pluripotent stem cells for cardiovascular disease modeling and precision medicine: a scientific statement from the American heart association. Circulation Genomic Precis. Med. 11 (1), e000043. doi:10.1161/HCG.0000000000000043
Nishino K., Takasawa K., Okamura K., Arai Y., Sekiya A., Akutsu H., et al. (2021). Identification of an epigenetic signature in human induced pluripotent stem cells using a linear machine learning model. Hum. Cell. 34 (1), 99–110. doi:10.1007/s13577-020-00446-3
Noiseux N., Mansour S., Weisel R., Stevens L. M., Der Sarkissian S., Tsang K., et al. (2016). The IMPACT-CABG trial: a multicenter, randomized clinical trial of CD133+ stem cell therapy during coronary artery bypass grafting for ischemic cardiomyopathy. J. Thorac. Cardiovasc Surg. 152 (6), 1582–1588. doi:10.1016/j.jtcvs.2016.07.067
Okita K., Ichisaka T., Yamanaka S. (2007). Generation of germline-competent induced pluripotent stem cells. Nature 448 (7151), 313–317. doi:10.1038/nature05934
Orlic D., Kajstura J., Chimenti S., Jakoniuk I., Anderson S. M., Li B., et al. (2001). Bone marrow cells regenerate infarcted myocardium. Nature 410 (6829), 701–705. doi:10.1038/35070587
Ostovaneh M. R., Makkar R. R., Ambale-Venkatesh B., Ascheim D., Chakravarty T., Henry T. D., et al. (2021). Effect of cardiosphere-derived cells on segmental myocardial function after myocardial infarction: ALLSTAR randomised clinical trial. Open Heart 8 (2), e001614. doi:10.1136/openhrt-2021-001614
Patel A. N., Henry T. D., Quyyumi A. A., Schaer G. L., Anderson R. D., Toma C., et al. (2016). Ixmyelocel-T for patients with ischaemic heart failure: a prospective randomised double-blind trial. Lancet 387 (10036), 2412–2421. doi:10.1016/S0140-6736(16)30137-4
Patel S. A., King C. C., Lim P. K., Habiba U., Dave M., Porecha R., et al. (2010). Personalizing stem cell research and therapy: the arduous road ahead or missed opportunity? Curr. Pharmacogenomics Person. Med. 8 (1), 25–36. doi:10.2174/1875692111008010025
Perin E. C., Borow K. M., Henry T. D., Mendelsohn F. O., Miller L. W., Swiggum E., et al. (2023). Randomized trial of targeted transendocardial mesenchymal precursor cell therapy in patients with heart failure. J. Am. Coll. Cardiol. 81 (9), 849–863. doi:10.1016/j.jacc.2022.11.061
Perin E. C., Borow K. M., Silva G. V., DeMaria A. N., Marroquin O. C., Huang P. P., et al. (2015). A phase II dose-escalation study of allogeneic mesenchymal precursor cells in patients with ischemic or nonischemic heart failure. Circ. Res. 117 (6), 576–584. doi:10.1161/CIRCRESAHA.115.306332
Perin E. C., Willerson J. T., Pepine C. J., Henry T. D., Ellis S. G., Zhao D. X. M., et al. (2012). Effect of transendocardial delivery of autologous bone marrow mononuclear cells on functional capacity, left ventricular function, and perfusion in chronic heart failure: the FOCUS-CCTRN trial. Jama 307 (16), 1717–1726. doi:10.1001/jama.2012.418
Pittenger M. F., Discher D. E., Péault B. M., Phinney D. G., Hare J. M., Caplan A. I. (2019). Mesenchymal stem cell perspective: cell biology to clinical progress. npj Regen. Med. 4 (1), 22. doi:10.1038/s41536-019-0083-6
Pittenger M. F., Mackay A. M., Beck S. C., Jaiswal R. K., Douglas R., Mosca J. D., et al. (1999). Multilineage potential of adult human mesenchymal stem cells. Science 284 (5411), 143–147. doi:10.1126/science.284.5411.143
Pizzute T., Lynch K., Pei M. (2015). Impact of tissue-specific stem cells on lineage-specific differentiation: a focus on the musculoskeletal system. Stem Cell. Rev. Rep. 11 (1), 119–132. doi:10.1007/s12015-014-9546-8
Qayyum A. A., Mouridsen M., Nilsson B., Gustafsson I., Schou M., Nielsen O. W., et al. (2023a). Danish phase II trial using adipose tissue derived mesenchymal stromal cells for patients with ischaemic heart failure. Esc. Heart Fail 10 (2), 1170–1183. doi:10.1002/ehf2.14281
Qayyum A. A., van Klarenbosch B., Frljak S., Cerar A., Poglajen G., Traxler-Weidenauer D., et al. (2023b). Effect of allogeneic adipose tissue-derived mesenchymal stromal cell treatment in chronic ischaemic heart failure with reduced ejection fraction - the SCIENCE trial. Eur. J. Heart Fail 25 (4), 576–587. doi:10.1002/ejhf.2772
Qi C. M., Ma G. S., Liu N. F., Shen C. x., Chen Z., Liu X. j., et al. (2008). Transplantation of magnetically labeled mesenchymal stem cells improves cardiac function in a swine myocardial infarction model. Chin. Med. J. Engl. 121 (6), 544–550. doi:10.1097/00029330-200803020-00016
Qian L., Huang Y., Spencer C. I., Foley A., Vedantham V., Liu L., et al. (2012). In vivo reprogramming of murine cardiac fibroblasts into induced cardiomyocytes. Nature 485 (7400), 593–598. doi:10.1038/nature11044
Qiu S., Joshi P. S., Miller M. I., Xue C., Zhou X., Karjadi C., et al. (2020). Development and validation of an interpretable deep learning framework for Alzheimer’s disease classification. Brain 143 (6), 1920–1933. doi:10.1093/brain/awaa137
Raval A. N., Johnston P. V., Duckers H. J., Cook T. D., Traverse J. H., Altman P. A., et al. (2021). Point of care, bone marrow mononuclear cell therapy in ischemic heart failure patients personalized for cell potency: 12-month feasibility results from CardiAMP heart failure roll-in cohort. Int. J. Cardiol. 326, 131–138. doi:10.1016/j.ijcard.2020.10.043
Rezaie J., Feghhi M., Etemadi T. (2022). A review on exosomes application in clinical trials: perspective, questions, and challenges. Cell. Commun. Signal. 20 (1), 145. doi:10.1186/s12964-022-00959-4
Rheault-Henry M., White I., Grover D., Atoui R. (2021). Stem cell therapy for heart failure: medical breakthrough, or dead end? World J. Stem Cells 13 (4), 236–259. doi:10.4252/wjsc.v13.i4.236
Riva P., Battaglia C., Venturin M. (2019). Emerging role of genetic alterations affecting exosome biology in neurodegenerative diseases. Int. J. Mol. Sci. 20 (17), 4113. doi:10.3390/ijms20174113
Savarese G., Becher P. M., Lund L. H., Seferovic P., Rosano G. M. C., Coats A. J. S. (2022). Global burden of heart failure: a comprehensive and updated review of epidemiology. Cardiovasc. Res. 118 (17), 3272–3287. doi:10.1093/cvr/cvac013
Schweitzer J. S., Song B., Herrington T. M., Park T. Y., Lee N., Ko S., et al. (2020). Personalized iPSC-derived dopamine progenitor cells for Parkinson’s disease. N. Engl. J. Med. 382 (20), 1926–1932. doi:10.1056/NEJMoa1915872
Segar M. W., Patel K. V., Ayers C., Basit M., Tang W. H. W., Willett D., et al. (2020). Phenomapping of patients with heart failure with preserved ejection fraction using machine learning-based unsupervised cluster analysis. Eur. J. Heart Fail. 22 (1), 148–158. doi:10.1002/ejhf.1621
Shi M., Xu G. (2019). Development and validation of GMI signature based random survival forest prognosis model to predict clinical outcome in acute myeloid leukemia. BMC Med. Genomics 12 (1), 90. doi:10.1186/s12920-019-0540-5
Simmons P. J., Torok-Storb B. (1991). Identification of stromal cell precursors in human bone marrow by a novel monoclonal antibody, STRO-1. Blood 78 (1), 55–62. doi:10.1182/blood.v78.1.55.55
Steinhoff G., Nesteruk J., Wolfien M., Kundt G., Borgermann J., et al. (2017). Cardiac function improvement and bone marrow response -: outcome analysis of the randomized PERFECT phase III clinical trial of intramyocardial CD133(+) application after myocardial infarction. EBioMedicine 22, 208–224. doi:10.1016/j.ebiom.2017.07.022
Takahashi K., Yamanaka S. (2006). Induction of pluripotent stem cells from mouse embryonic and adult fibroblast cultures by defined factors. Cell. 126 (4), 663–676. doi:10.1016/j.cell.2006.07.024
Taylor D. A., Perin E. C., Willerson J. T., Zierold C., Resende M., Carlson M., et al. (2016). Identification of bone marrow cell subpopulations associated with improved functional outcomes in patients with chronic left ventricular dysfunction: an embedded cohort evaluation of the FOCUS-CCTRN trial. Cell. Transpl. 25 (9), 1675–1687. doi:10.3727/096368915X689901
Teerlink J. R., Metra M., Filippatos G. S., Davison B. A., Bartunek J., Terzic A., et al. (2017). Benefit of cardiopoietic mesenchymal stem cell therapy on left ventricular remodelling: results from the Congestive Heart Failure Cardiopoietic Regenerative Therapy (CHART-1) study. Eur. J. Heart Fail 19 (11), 1520–1529. doi:10.1002/ejhf.898
Tsao C. W., Aday A. W., Almarzooq Z. I., Alonso A., Beaton A. Z., Bittencourt M. S., et al. (2022). Heart disease and stroke statistics—2022 update: a report from the American heart association. Circulation 145 (8), e153–e639. doi:10.1161/CIR.0000000000001052
Tsilimigras D. I., Oikonomou E. K., Moris D., Schizas D., Economopoulos K. P., Mylonas K. S. (2017). Stem cell therapy for congenital heart disease: a systematic review. Circulation 136 (24), 2373–2385. doi:10.1161/CIRCULATIONAHA.117.029607
Ulus A. T., Mungan C., Kurtoglu M., Celikkan F. T., Akyol M., Sucu M., et al. (2020). Intramyocardial transplantation of umbilical cord mesenchymal stromal cells in chronic ischemic cardiomyopathy: a controlled, randomized clinical trial (HUC-heart trial). Int. J. Stem Cells 13 (3), 364–376. doi:10.15283/ijsc20075
Uygur A., Lee Richard T. (2016). Mechanisms of cardiac regeneration. Dev. Cell. 36 (4), 362–374. doi:10.1016/j.devcel.2016.01.018
van der Spoel T. I., Jansen of Lorkeers S. J., Agostoni P., van Belle E., Gyöngyösi M., Sluijter J. P. G., et al. (2011a). Human relevance of pre-clinical studies in stem cell therapy: systematic review and meta-analysis of large animal models of ischaemic heart disease. Cardiovasc Res. 91 (4), 649–658. doi:10.1093/cvr/cvr113
Vrtovec B., Poglajen G., Sever M., Zemljic G., Frljak S., Cerar A., et al. (2018). Effects of repetitive transendocardial CD34+ cell transplantation in patients with nonischemic dilated cardiomyopathy. Circ. Res. 123 (3), 389–396. doi:10.1161/CIRCRESAHA.117.312170
Waisman A., La Greca A., Möbbs A. M., Scarafía M. A., Santín Velazque N. L., Neiman G., et al. (2019). Deep learning neural networks highly predict very early onset of pluripotent stem cell differentiation. Stem Cell. Rep. 12 (4), 845–859. doi:10.1016/j.stemcr.2019.02.004
Wang Y., Wang Z., Xie K., Zhao X., Jiang X., Chen B., et al. (2020). High-efficiency cellular reprogramming by nanoscale puncturing. Nano Lett. 20 (7), 5473–5481. doi:10.1021/acs.nanolett.0c01979
Wang Y., Zhang L., Li Y., Chen L., Wang X., Guo W., et al. (2015). Exosomes/microvesicles from induced pluripotent stem cells deliver cardioprotective miRNAs and prevent cardiomyocyte apoptosis in the ischemic myocardium. Int. J. Cardiol. 192, 61–69. doi:10.1016/j.ijcard.2015.05.020
Wen H., Peng L., Chen Y. (2021). The effect of immune cell-derived exosomes in the cardiac tissue repair after myocardial infarction: molecular mechanisms and pre-clinical evidence. J. Cell. Mol. Med. 25 (14), 6500–6510. doi:10.1111/jcmm.16686
Wessels A., Sedmera D. (2003). Developmental anatomy of the heart: a tale of mice and man. Physiol. Genomics 15 (3), 165–176. doi:10.1152/physiolgenomics.00033.2003
Wu P., Sai X., Li Z., Ye X., Jin L., Liu G., et al. (2023). Maturation of induced pluripotent stem cell-derived cardiomyocytes and its therapeutic effect on myocardial infarction in mouse. Bioact. Mater 20, 286–305. doi:10.1016/j.bioactmat.2022.05.024
Xiao W., Guo S., Gao C., Dai G., Gao Y., Li M., et al. (2017). A randomized comparative study on the efficacy of intracoronary infusion of autologous bone marrow mononuclear cells and mesenchymal stem cells in patients with dilated cardiomyopathy. Int. Heart J. 58 (2), 238–244. doi:10.1536/ihj.16-328
Yang Y., Liu B., Xu J., Wang J., Wu J., Shi C., et al. (2017). Derivation of pluripotent stem cells with in vivo embryonic and extraembryonic potency. Cell. 169 (2), 243–257. doi:10.1016/j.cell.2017.02.005
Yau T. M., Pagani F. D., Mancini D. M., Chang H. L., Lala A., Woo Y. J., et al. (2019). Intramyocardial injection of mesenchymal precursor cells and successful temporary weaning from left ventricular assist device support in patients with advanced heart failure: a randomized clinical trial. JAMA 321 (12), 1176–1186. doi:10.1001/jama.2019.2341
Ye J., Ge J., Zhang X., Cheng L., Zhang Z., He S., et al. (2016). Pluripotent stem cells induced from mouse neural stem cells and small intestinal epithelial cells by small molecule compounds. Cell. Res. 26 (1), 34–45. doi:10.1038/cr.2015.142
Zheng Y. L. (2016). Some ethical concerns about human induced pluripotent stem cells. Sci. Eng. Ethics 22 (5), 1277–1284. doi:10.1007/s11948-015-9693-6
Zheng Y. L., Wang W. D., Cai P. Y., Zheng F., Zhou Y. F., Li M. M., et al. (2022). Stem cell-derived exosomes in the treatment of acute myocardial infarction in preclinical animal models: a meta-analysis of randomized controlled trials. Stem Cell. Res. Ther. 13 (1), 151. doi:10.1186/s13287-022-02833-z
Zhu Y., Huang R., Wu Z., Song S., Cheng L., Zhu R. (2021). Deep learning-based predictive identification of neural stem cell differentiation. Nat. Commun. 12 (1), 2614. doi:10.1038/s41467-021-22758-0
Keywords: stem cells, heart failure, cell therapy, precision medicine, artificial intelligence, machine learning, clinical trial, regenerative medicine
Citation: Chowdhury MA, Zhang JJ, Rizk R and Chen WCW (2024) Stem cell therapy for heart failure in the clinics: new perspectives in the era of precision medicine and artificial intelligence. Front. Physiol. 14:1344885. doi: 10.3389/fphys.2023.1344885
Received: 26 November 2023; Accepted: 26 December 2023;
Published: 09 January 2024.
Edited by:
Meijing Wang, Indiana University Bloomington, United StatesCopyright © 2024 Chowdhury, Zhang, Rizk and Chen. This is an open-access article distributed under the terms of the Creative Commons Attribution License (CC BY). The use, distribution or reproduction in other forums is permitted, provided the original author(s) and the copyright owner(s) are credited and that the original publication in this journal is cited, in accordance with accepted academic practice. No use, distribution or reproduction is permitted which does not comply with these terms.
*Correspondence: William C. W. Chen, V2lsbGlhbS5DaGVuQHVzZC5lZHU=
Disclaimer: All claims expressed in this article are solely those of the authors and do not necessarily represent those of their affiliated organizations, or those of the publisher, the editors and the reviewers. Any product that may be evaluated in this article or claim that may be made by its manufacturer is not guaranteed or endorsed by the publisher.
Research integrity at Frontiers
Learn more about the work of our research integrity team to safeguard the quality of each article we publish.