- 1Department of Surgery, SUNY Upstate Medical Center, Syracuse, NY, United States
- 2Roy J. Carver Department of Biomedical Engineering, University of Iowa, Iowa City, IA, United States
- 3Department of Medicine, University of Maryland, Baltimore, MD, United States
- 4Neonatal Research, Murdoch Children’s Research Institute, Royal Children’s Hospital, Parkville, VIC, Australia
- 5Department of Biomedical Engineering, Tulane University, New Orleans, LA, United States
- 6Department of Medicine, University of Vermont, Burlington, VT, United States
- 7Departments of Anesthesia and Radiology, University of Iowa, Iowa City, IA, United States
Patients with acute respiratory distress syndrome (ARDS) have few treatment options other than supportive mechanical ventilation. The mortality associated with ARDS remains unacceptably high, and mechanical ventilation itself has the potential to increase mortality further by unintended ventilator-induced lung injury (VILI). Thus, there is motivation to improve management of ventilation in patients with ARDS. The immediate goal of mechanical ventilation in ARDS should be to prevent atelectrauma resulting from repetitive alveolar collapse and reopening. However, a long-term goal should be to re-open collapsed and edematous regions of the lung and reduce regions of high mechanical stress that lead to regional volutrauma. In this paper, we consider the proposed strategy used by the full-term newborn to open the fluid-filled lung during the initial breaths of life, by ratcheting tissues opened over a series of initial breaths with brief expirations. The newborn’s cry after birth shares key similarities with the Airway Pressure Release Ventilation (APRV) modality, in which the expiratory duration is sufficiently short to minimize end-expiratory derecruitment. Using a simple computational model of the injured lung, we demonstrate that APRV can slowly open even the most recalcitrant alveoli with extended periods of high inspiratory pressure, while reducing alveolar re-collapse with brief expirations. These processes together comprise a ratchet mechanism by which the lung is progressively recruited, similar to the manner in which the newborn lung is aerated during a series of cries, albeit over longer time scales.
Introduction
Although acute respiratory distress syndrome (ARDS) was identified as a distinct pathologic entity in 1967 (Ashbaugh et al., 1967), there are still no treatments for it other than supportive care involving mechanical ventilation. Unfortunately, mechanical ventilation itself has the potential to increase mortality by causing unintended ventilator-induced lung injury (VILI) (Slutsky and Ranieri, 2013). The current standard of care for ARDS is low tidal volume ventilation (Acute Respiratory Distress Syndrome Network et al., 2000). Nevertheless, mortality associated with ARDS remains unacceptably high (Bellani et al., 2016), which motivates the search for improved approaches to mechanical ventilation.
ARDS typically presents as a heterogeneous pattern of injury on chest imaging, including patches of normal, collapsible, and fully consolidated tissue throughout the lung (Cereda et al., 2016; Cereda et al., 2019). This makes the lung particularly susceptible to further VILI by a mechanism that has been termed the Permeability-Originated Obstruction Response (POOR), manifesting at interfaces between normal and collapsed lung tissue regions (Gaver et al., 2020). The normal parenchyma at such interfaces is distorted by retraction forces exerted by collapsed regions, resulting in elevated tissue stresses that can potentially cause volutrauma. In addition, chronic atelectasis increases the risk of pulmonary infection and fibrosis. However, atelectrauma at these interfaces is also caused by repetitive opening and closing of alveoli with abnormal mechanical properties due to surfactant dysfunction, although such alveoli may not yet fully consolidated (Nieman et al., 2017). While both volutrauma and atelectrauma are key mechanisms of VILI, atelectrauma appears to be the primary driver of its progression (Ramcharran et al., 2022). Both mechanisms together thus cause VILI to spatially propagate outward from consolidated regions in a “POOR-get-POORer” manner, wherein edema-induced surfactant deactivation results in regional areas of instability, with focalized stress multipliers leading to a perpetuating cycle of VILI. This can result in a cascading, irrecoverable injury, which has been termed the “VILI Vortex” (Marini and Gattinoni, 2020; Nieman et al., 2022).
The above considerations indicate that the immediate goal of mechanical ventilation in ARDS should be to prevent unstable alveoli from contributing to atelectrauma. A more long-term goal should be to re-open collapsed and edematous regions and to eliminate the concentrations of high mechanical stress in adjacent aerated tissue that lead to regional volutrauma (Knudsen et al., 2017). Such are the goals of the Open Lung Approach (OLA), which applies positive end-expiratory pressure (PEEP) to prevent alveoli from collapsing during expiration, and periodically administers large, sustained inflations to recruit collapsed tissue (Writing Group for the Alveolar Recruitment for Acute Respiratory Distress Syndrome Trial et al., 2017). While scientific and clinical evidence suggests that the lung would be protected from VILI if these goals could be achieved (Cinnella et al., 2015; Cereda et al., 2016; Gaver et al., 2020), the OLA has failed to reduce the mortality associated with ARDS in randomized clinical trials (Writing Group for the Alveolar Recruitment for Acute Respiratory Distress Syndrome Trial et al., 2017). Moreover, physiologic, and radiographic indices (Writing Group for the Alveolar Recruitment for Acute Respiratory Distress Syndrome Trial et al., 2017) suggest that the OLA, as currently practiced, does not maintain durable recruitment of the unstable regions of the injured lung. Indeed, transient recruitment of the lung may increase the number of alveoli subject to atelectrauma, if subsequent derecruitment is not prevented (Halter et al., 2003).
The question remains: is there an approach to mechanical ventilation that safely reduces repetitive atelectrauma, while simultaneously reopening collapsed and edematous lung tissue? In this paper, we consider the strategy used by the full-term newborn to open the fluid-filled lung during the initial breaths of life. The newborn’s cry after birth shares key similarities with the Airway Pressure Release Ventilation (APRV) modality, in which the expiration duration is sufficiently short to minimize end-expiratory derecruitment. Using a simple computational model of the injured lung that incorporates mechanisms for fast and slow recruitment, we demonstrate that APRV, when administered in an appropriate manner, may be an answer to the above question.
Opening the fluid-filled lung of the newborn
At birth, the healthy, full-term infant faces the same problem as the clinician managing a patient with ARDS—that is, how to open a fluid-filled lung. The ability to maintain aeration in previously obstructed, flooded or collapsed lung tissue is critical to the survival of both the newborn and the patient with ARDS. In a recent paper, Tingay et al. (2021) explored a strategy by which nature opens and maintains recruitment in the newborn lung.
The lungs of an early to full-term newborn (i.e., ≥36 weeks gestation) with a functioning surfactant system are filled with amniotic fluid at birth. Upon delivery, the newborn inhales vigorously, and often emits a cry with its first exhalation. This initial inspiration forces air into the small airways and acini, displacing amniotic fluid into more distal lung regions, where it may be cleared through the interstitium (Jain and Eaton, 2006). Following initial inhalation, the physiological problem shifts to preventing newly aerated tissue from collapsing during the subsequent exhalation. To do so, the neonate is believed to impede expiratory flow by partially closing the glottis to reduce expiratory flow, which transiently raises pressure in the lung and helps to move gas distally into partially flooded alveoli. A subsequent series of cries continues to ratchet the lung progressively open, until it is fully recruited and surfactant function is established. At this point, the alveoli remain open and stable, such that normal tidal ventilation can be maintained without further derecruitment. Thus, the first ratcheting breaths of the crying newborn adds gas volume to the portion of open lung with each inspiration, without losing ground during each expiration.
Airway pressure release ventilation for patients with ARDS
Edematous and collapsed regions of the lung in ARDS can be difficult to recruit because of proteinaceous fluid entering alveoli through the disrupted alveolar-capillary barrier, which deactivates pulmonary surfactant. Even small amounts of recruitment achieved through the application of high airway pressure may be lost quickly once such pressure is released (Lu et al., 2006). This is due to both time and pressure dependencies of alveolar recruitment and derecruitment. In other words, high airway pressures do not necessarily open collapsed alveoli immediately. Recruitment may require a long time to manifest when surfactant deactivation is severe—possibly even longer than the duration of even the most protracted single recruitment maneuver.
The APRV modality may allow for such gradual recruitment, provided it is administered with sufficiently short expiratory durations. With APRV, expiratory durations are short enough such that recruited alveoli are not given sufficient time to close. This strategy, which reopens edematous lungs (Veneroni et al., 2020; Tingay et al., 2021), also gradually ratchets open the injured lung open over an extended period of hours or days, during which adequate ventilation may be achieved via rapid and periodic removal of alveolar gas This is similar to the application of an “adaptive” PEEP to the lungs (Lam et al., 2020; Herrmann et al., 2021). A more personalized approach to APRV, termed the Time-Controlled Adaptive Ventilation (TCAV) (Nieman et al., 2020), has been shown to be an effective means of choosing expiratory durations that balance alveolar CO2 elimination vs. alveolar re-collapse. TCAV adjusts the expiratory duration according to the slope of the expiratory flow curve, which is governed by the emptying time-constant of the respiratory system (Herrmann et al., 2021). With TCAV, expiration is terminated when the magnitude of expiratory flow has fallen to 75% of its peak value at the start of expiration. TCAV has been shown to be highly protective against VILI in clinically relevant animal models of ARDS (Roy et al., 2013; Kollisch-Singule et al., 2015).
The newborn cry compared to APRV: a computational modeling analysis
Figure 1 compares the volume vs. time and flow vs. time relationships for a newborn cry and TCAV that demonstrate some common features. They both employ a rapid inspiratory phase to aerate lung tissue. The newborn follows this inspiration with a rapid exhalation, that is, then impeded through mechanisms such as glottal restriction, which leads to a prolongation exhalation. For TCAV, the breath-hold maneuver precedes exhalation but, similar to that of the newborn, this exhalation is quickly interrupted to prevent collapse of the newly recruited tissue. Such a breath profile leads to the question: “Can the collapsed and edematous lung with ARDS also be ratcheted opened, similar to the neonatal lung?” A secondary question is: “Can such ratcheting occur simultaneously with the maintenance of adequate ventilation of alveoli that are already open?”
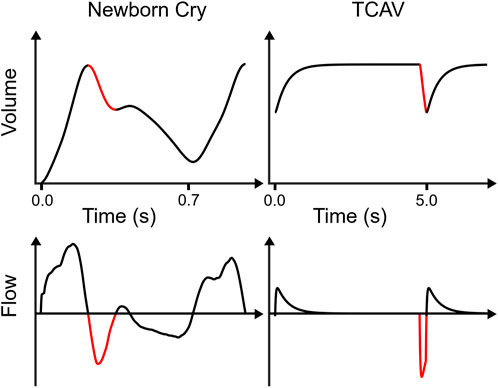
FIGURE 1. Comparisons between newborn cry and TCAV lung volume vs. time and respiratory flow vs. time. The newborn lung volume waveform shows data from a surrogate measurement (i.e., relative volume change since birth assessed by electrical impedance tomography) published by Tingay et al. (2021), then smoothed and time-differentiated to obtain flow. The TCAV waveforms were simulated in a single-compartment resistance-compliance-inertance model of respiratory mechanics. TCAV applies a prolonged high airway pressure, maintaining high lung volume. Both waveforms exhibit a rapid initial exhalation (red) with large negative flow rates for a brief time before an expiratory “brake” abruptly halts volume change. However, the newborn waveform continues with a second, slower exhalation phase, whereas TCAV immediately reinflates. Note the increase in the newborn lung volume between the beginning and end of the first breath. Note that the newborn breath duration is approximately 0.7 s, whereas each simulated TCAV cycle is 5 s.
To answer these questions, it is important to understand how quickly the expiration should be terminated. This is a critical issue because derecruitment in the injured lung can be rapid, so significant alveolar closure can occur even when expiration is substantially shorter than in conventional mechanical ventilation. If derecruitment occurs, alveoli that close must be forced open during the next inspiration, resulting in atelectrauma. This may be a highly injurious process that quickly leads to severe VILI if allowed to occur repeatedly (Kollisch-Singule et al., 2014).
In a previous study (Bates et al., 2020), we represented the lung as a single alveolar compartment that can expand in two orthogonal directions, as illustrated in Figure 2A. Airway pressure and flow are denoted as P and
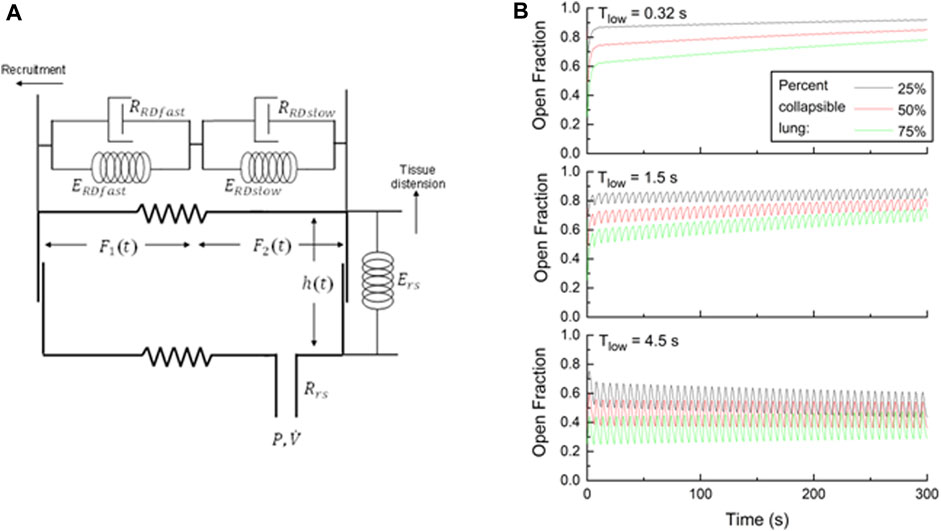
FIGURE 2. (A) Single-compartment model of the respiratory system in which the alveolar compartment expands vertically to represent tissue distension, but horizontally to represent recruitment. Variables for the model parameters are defined in the text. (B) Open fractions of the lung
Figure 2B shows how the fraction of open lung
Repeating this simulation with Thigh = 4.5 s and Tlow = 1.5 s illustrates the problem associated with using typical APRV settings without the TCAV strategy. The long-term recruitment trends remain, albeit delayed relative to the simulations with Tlow = 0.32 s, but there are now noticeable oscillations in
Conclusion
In summary, we have reviewed the key similarities and differences between the newborn cry and the mechanical breath of the APRV/TCAV modality. Nature has devised a unique mechanical strategy for opening the newborn lung, allowing it to transition rapidly from its fluid-filled state in utero to the air-filled state necessary for survival outside the womb. The newborn lung achieves this vital function by ratcheting lung tissue open over a series of initial breaths that incorporate brief expirations, to avoid undoing the gains made with each previous inspiration. A similar challenge faces the clinician who must ventilation the collapsed and edematous lung with ARDS, but with an added complication. Recruitment in an injured lung typically takes longer to manifest than in the neonatal lung, with ongoing ventilation of those alveoli already opened. APRV administered using TCAV achieves these goals, with rapid lung inflation to move fluid from the airways into the interstitial spaces, thus opening collapsed airways and alveoli. Extended periods of high inspiratory pressure slowly recruits even the most recalcitrant alveoli, while brief expirations avoid re-collapse of those alveoli already opened. These processes together comprise a ratchet mechanism by which the lung is progressively recruited, much in the same way that the newborn lung is aerated during a series of cries, albeit over a longer time scale.
Data availability statement
The raw data supporting the conclusion of this article will be made available by the authors, without undue reservation.
Author contributions
GN: Conceptualization, Funding acquisition, Methodology, Supervision, Writing–original draft, Writing–review and editing, Formal Analysis. JH: Conceptualization, Writing–original draft, Writing–review and editing. JS: Conceptualization, Writing–review and editing. MK-S: Writing–original draft, Writing–review and editing. PA: Writing–review and editing, Conceptualization. NH: Conceptualization, Writing–review and editing. DT: Conceptualization, Writing–review and editing, Funding acquisition. DG: Conceptualization, Funding acquisition, Writing–original draft, Writing–review and editing. JB: Conceptualization, Funding acquisition, Writing–original draft, Writing–review and editing, Methodology. DK: Conceptualization, Funding acquisition, Methodology, Writing–original draft, Writing–review and editing, Supervision.
Funding
The author(s) declare financial support was received for the research, authorship, and/or publication of this article. This work was supported by the Office of the Assistant Secretary of Defense for Health Affairs, through the Peer Reviewed Medical Research Program under Award Nos. W81XWH-20-1-0696 and W81XWH-21-1-0507. Opinions, interpretations, conclusions, and recommendations are those of the authors and are not necessarily endorsed by the Department of Defense. This work was also supported by the National Institutes of Health award R01 HL142702. Other support was given by National Health and Medical Research Council of Australia (ID2008212 and ID1192676) and Victorian State Government (Operational Infrastructure Support Program).
Conflict of interest
MK-S has received a Research Grant and has received equipment loans and NH has received an Unrestricted Educational Grant from Drager Medical Systems, Inc. MK-S and NH have presented and received honoraria and/or travel reimbursement at event(s) sponsored by Drager Medical Systems, Inc., outside of the published work. GN and MK-S have lectured for Intensive Care Online Network, Inc. (ICON). NH holds patents on a method of initiating, managing and/or weaning airway pressure release ventilation, as well as controlling a ventilator in accordance with the same, but these patents are not commercialized, licensed, or royalty-producing. DK and JH are co-founders and shareholders of OscillaVent, Inc., and are co-inventors on a patent involving multi-frequency oscillatory ventilation. DK and JH also receive research support from ZOLL Medical Corporation. JB is a consultant to and shareholder of OscillaVent, Inc., and has two patents pending in the field of mechanical ventilation.
The remaining authors declare that the research was conducted in the absence of any commercial or financial relationships that could be construed as a potential conflict of interest.
The author(s) declared that they were an editorial board member of Frontiers, at the time of submission. This had no impact on the peer review process and the final decision.
Publisher’s note
All claims expressed in this article are solely those of the authors and do not necessarily represent those of their affiliated organizations, or those of the publisher, the editors and the reviewers. Any product that may be evaluated in this article, or claim that may be made by its manufacturer, is not guaranteed or endorsed by the publisher.
Supplementary material
The Supplementary Material for this article can be found online at: https://www.frontiersin.org/articles/10.3389/fphys.2023.1287416/full#supplementary-material
References
Acute RespiratoryDistress Syndrome Network Brower R. G., Matthay M. A., Morris A., Schoenfeld D., Thompson B. T., Wheeler A. (2000). Ventilation with lower tidal volumes as compared with traditional tidal volumes for acute lung injury and the acute respiratory distress syndrome. N. Engl. J. Med. 342, 1301–1308. doi:10.1056/NEJM200005043421801
Ashbaugh D. G., Bigelow D. B., Petty T. L., Levine B. E. (1967). Acute respiratory distress in adults. Lancet 2, 319–323. doi:10.1016/s0140-6736(67)90168-7
Bates J. H. T., Gaver D. P., Habashi N. M., Nieman G. F. (2020). Atelectrauma versus volutrauma: a tale of two time-constants. Crit. Care Explor 2, e0299. doi:10.1097/CCE.0000000000000299
Bellani G., Laffey J. G., Pham T., Fan E., Brochard L., Esteban A., et al. (2016). Epidemiology, patterns of care, and mortality for patients with acute respiratory distress syndrome in intensive care units in 50 countries. JAMA 315, 788–800. doi:10.1001/jama.2016.0291
Cereda M., Xin Y., Goffi A., Herrmann J., Kaczka D. W., Kavanagh B. P., et al. (2019). Imaging the injured lung: mechanisms of action and clinical use. Anesthesiology 131, 716–749. doi:10.1097/ALN.0000000000002583
Cereda M., Xin Y., Meeder N., Zeng J., Jiang Y., Hamedani H., et al. (2016). Visualizing the propagation of acute lung injury. Anesthesiology 124, 121–131. doi:10.1097/ALN.0000000000000916
Cinnella G., Grasso S., Raimondo P., D'antini D., Mirabella L., Rauseo M., et al. (2015). Physiological effects of the open lung approach in patients with early, mild, diffuse acute respiratory distress syndrome: an electrical Impedance tomography study. Anesthesiology 123, 1113–1121. doi:10.1097/ALN.0000000000000862
Gaver D. P., Nieman G. F., Gatto L. A., Cereda M., Habashi N. M., Bates J. H. T. (2020). The POOR get POORer: a hypothesis for the pathogenesis of ventilator-induced lung injury. Am. J. Respir. Crit. Care Med. 202, 1081–1087. doi:10.1164/rccm.202002-0453CP
Halter J. M., Steinberg J. M., Schiller H. J., Dasilva M., Gatto L. A., Landas S., et al. (2003). Positive end-expiratory pressure after a recruitment maneuver prevents both alveolar collapse and recruitment/derecruitment. Am. J. Respir. Crit. Care Med. 167, 1620–1626. doi:10.1164/rccm.200205-435OC
Herrmann J., Gerard S. E., Shao W., Xin Y., Cereda M., Reinhardt J. M., et al. (2021). Effects of lung injury on regional aeration and expiratory time constants: insights from four-dimensional computed tomography image registration. Front. Physiol. 12, 707119. doi:10.3389/fphys.2021.707119
Jain L., Eaton D. C. (2006). Physiology of fetal lung fluid clearance and the effect of labor. Semin. Perinatol. 30, 34–43. doi:10.1053/j.semperi.2006.01.006
Knudsen L., Ruppert C., Ochs M. (2017). Tissue remodelling in pulmonary fibrosis. Cell Tissue Res. 367, 607–626. doi:10.1007/s00441-016-2543-2
Kollisch-Singule M., Emr B., Jain S. V., Andrews P., Satalin J., Liu J., et al. (2015). The effects of airway pressure release ventilation on respiratory mechanics in extrapulmonary lung injury. Intensive Care Med. Exp. 3, 35. doi:10.1186/s40635-015-0071-0
Kollisch-Singule M., Emr B., Smith B., Ruiz C., Roy S., Meng Q., et al. (2014). Airway pressure release ventilation reduces conducting airway micro-strain in lung injury. J. Am. Coll. Surg. 219, 968–976. doi:10.1016/j.jamcollsurg.2014.09.011
Lam R., Schilling D., Scottoline B., Platteau A., Niederhausen M., Lund K. C., et al. (2020). The effect of extended continuous positive airway pressure on changes in lung volumes in stable premature infants: a randomized controlled trial. J. Pediatr. 217, 66–72. doi:10.1016/j.jpeds.2019.07.074
Lu Q., Constantin J. M., Nieszkowska A., Elman M., Vieira S., Rouby J. J. (2006). Measurement of alveolar derecruitment in patients with acute lung injury: computerized tomography versus pressure-volume curve. Crit. Care 10, R95. doi:10.1186/cc4956
Marini J. J., Gattinoni L. (2020). Time course of evolving ventilator-induced lung injury: the "Shrinking Baby Lung. Crit. Care Med. 48, 1203–1209. doi:10.1097/CCM.0000000000004416
Nieman G., Kollisch-Singule M., Ramcharran H., Satalin J., Blair S., Gatto L. A., et al. (2022). Unshrinking the baby lung to calm the VILI vortex. Crit. Care 26, 242. doi:10.1186/s13054-022-04105-x
Nieman G. F., Gatto L. A., Andrews P., Satalin J., Camporota L., Daxon B., et al. (2020). Prevention and treatment of acute lung injury with time-controlled adaptive ventilation: physiologically informed modification of airway pressure release ventilation. Ann. Intensive Care 10, 3. doi:10.1186/s13613-019-0619-3
Nieman G. F., Satalin J., Kollisch-Singule M., Andrews P., Aiash H., Habashi N. M., et al. (2017). Physiology in Medicine: understanding dynamic alveolar physiology to minimize ventilator-induced lung injury. J. Appl. Physiol. (1985) 122, 1516–1522. doi:10.1152/japplphysiol.00123.2017
Ramcharran H., Bates J. H. T., Satalin J., Blair S., Andrews P. L., Gaver D. P., et al. (2022). Protective ventilation in a pig model of acute lung injury: timing is as important as pressure. J. Appl. Physiol. (1985) 133, 1093–1105. doi:10.1152/japplphysiol.00312.2022
Roy S., Habashi N., Sadowitz B., Andrews P., Ge L., Wang G. R., et al. (2013). Early airway pressure release ventilation prevents ARDS-a novel preventive approach to lung injury. Shock 39, 28–38. doi:10.1097/SHK.0b013e31827b47bb
Slutsky A. S., Ranieri V. M. (2013). Ventilator-induced lung injury. N. Engl. J. Med. 369, 2126–2136. doi:10.1056/NEJMra1208707
Tingay D. G., Farrell O., Thomson J., Perkins E. J., Pereira-Fantini P. M., Waldmann A. D., et al. (2021). Imaging the respiratory transition at birth: unraveling the complexities of the first breaths of life. Am. J. Respir. Crit. Care Med. 204, 82–91. doi:10.1164/rccm.202007-2997OC
Veneroni C., Tingay D. G., Mccall K. E., Pereira-Fantini P. M., Perkins E. J., Dargaville P. A., et al. (2020). Respiratory mechanics during initial lung aeration at birth in the preterm lamb. Am. J. Physiol. Lung Cell Mol. Physiol. 318, L525–L532. doi:10.1152/ajplung.00302.2019
Writing Group For The Alveolar Recruitment For Acute Respiratory Distress Syndrome Trial Cavalcanti A. B., Suzumura E. A., Laranjeira L. N., Paisani D. M., Damiani L. P., et al. (2017). Effect of lung recruitment and titrated positive end-expiratory pressure (PEEP) vs low PEEP on mortality in patients with acute respiratory distress syndrome: a randomized clinical trial. JAMA 318, 1335–1345. doi:10.1001/jama.2017.14171
Keywords: ARDS, airway pressure release ventilation, time-controlled adaptive ventilation, ventilator-induced lung injury, lung recruitment
Citation: Nieman GF, Herrmann J, Satalin J, Kollisch-Singule M, Andrews PL, Habashi NM, Tingay DG, Gaver DP III, Bates JHT and Kaczka DW (2023) Ratchet recruitment in the acute respiratory distress syndrome: lessons from the newborn cry. Front. Physiol. 14:1287416. doi: 10.3389/fphys.2023.1287416
Received: 01 September 2023; Accepted: 19 October 2023;
Published: 01 November 2023.
Edited by:
Alysson Roncally Silva Carvalho, University of Porto, PortugalReviewed by:
Roberta Südy, University of Geneva, SwitzerlandCopyright © 2023 Nieman, Herrmann, Satalin, Kollisch-Singule, Andrews, Habashi, Tingay, Gaver, Bates and Kaczka. This is an open-access article distributed under the terms of the Creative Commons Attribution License (CC BY). The use, distribution or reproduction in other forums is permitted, provided the original author(s) and the copyright owner(s) are credited and that the original publication in this journal is cited, in accordance with accepted academic practice. No use, distribution or reproduction is permitted which does not comply with these terms.
*Correspondence: David W. Kaczka, ZGF2aWQta2FjemthQHVpb3dhLmVkdQ==