- 1Neuromuscular Research Laboratory, Warrior Human Performance Research Center, University of Pittsburgh, Pittsburgh, PA, United States
- 2Military Performance Division, United States Army Research Institute of Environmental Medicine, Natick, MA, United States
- 3Department of Epidemiology, School of Public Health, University of Pittsburgh, Pittsburgh, PA, United States
- 4Army Health and Performance Research, UK Army, Andover, United Kingdom
Healthy bone adjusts its traits in an exceptionally coordinated, compensatory process. Recent advancements in skeletal imaging via High-Resolution Peripheral Quantitative Computed Tomography (HR-pQCT) allows for the in vivo 3-dimensional and longitudinal quantification of bone density, microarchitecture, geometry, and parameters of mechanical strength in response to varying strain stimuli including those resulting from exercise or military training. Further, the voxel size of 61 microns has the potential to capture subtle changes in human bone in as little as 8 weeks. Given the typical time course of bone remodeling, short-term detection of skeletal changes in bone microstructure and morphology is indicative of adaptive bone formation, the deposition of new bone formation, uncoupled from prior resorption, that can occur at mechanistically advantageous regions. This review aims to synthesize existing training-induced HR-pQCT data in three distinct populations of healthy adults excluding disease states, pharmacological intervention and nutritional supplementation. Those included are: 1) military basic or officer training 2) general population and 3) non-osteoporotic aging. This review aims to further identify similarities and contrasts with prior modalities and cumulatively interpret results within the scope of bone functional adaptation.
1 Introduction
Julius Wolff’s “Law of Bone Transformation” in 1892 and Harold Frost’s “Mechanostat Theory” nearly a century later, laid the foundational framework in which bone—once thought of as an inert, unchanging tissue—functionally adapts to its environment simultaneously meeting the contradictory needs of stiffness and flexibility during impact loading, muscle contraction and joint movement (Wolff, 1986; Frost, 1987; Seeman, 2002; Frost, 2003; Jepsen, 2009). Mechanical loading as a consequence of exercise training causes varying degrees of tissue-level deformation of bone’s extracellular matrix dependent on whole bone stiffness. This deformation results in a strain stimulus which if greater than customary, and heavily influenced by osteocyte viability, may elicit one of two adaptive processes: formation modeling or targeted remodeling (Hughes and Bouxsein, 2020). Targeted remodeling, whereby osteoblast and osteoclast activity are temporally coupled with the objective of resorbing and replacing small packets of damaged tissue is important for modulating microdamage—that is, tissue deterioration at the micro- and nanostructure level (Morgan, Unnikrisnan, and Hussein 2018)—with the resultant porous space creating a transient negative bone balance that can reduce the stiffness, strength, and fatigue resistance of bone until the site is fully replenished by secondary mineralization, an extensive process that can take upwards of 30 months (Boivin et al., 2009; Plotkin, 2014; Hughes et al., 2017; Mancuso et al., 2022). Conversely, adaptive bone formation, a process of formation modeling, is the independent action of osteoblasts uncoupled from prior osteoclastic bone resorption remodeling that ultimately deposits bone at mechanically advantageous places intended to improve the morphology of the structure (Hughes et al., 2017; Hughes and Bouxsein, 2020). Further, adaptive bone formation, bypassing the resorption period with a median duration of 30–40 days (Eriksen, 2010), is temporally more expedient than is remodeling, foregoing the transient porosity and subsequent increased risk of bone stress injury.
Reports from in vivo loading models dating back to the 1960s have established the ability to capture these complex physiologic pathways in animal species (Frost, 2003). Such studies have shown improvement in bone mineral density, trabecular microstructure, and bone geometry in as little as 4 weeks (Mustafy et al., 2019). In another, after 8 weeks of 10, 45 cm freefall drops, bone volume fraction, trabecular and cortical thickness improved in rat ulnas, with greater adaptation resulting from impact than from calcium supplementation (Welch et al., 2008). A study performed over 16 weeks subjected rats to 360 load cycles per day, resulting in significant increases in biomechanical resilience, specifically ultimate force and energy to failure, occurring despite only modest gains in areal bone mineral density (aBMD) and bone mineral content (BMC) (Robling and Turner, 2009). These results have provided the scope in which exercise interventions in human research commonly range in duration from just 4–12 weeks (Linke, Gallo, and Norman, 2011; Hecksteden et al., 2018; Ashton et al., 2020). However, due to rodent cortical bone lacking well-developed haversian remodeling activity (Bentolila et al., 1998)—and the majority of animal models designed for compact bone—resorption data from animal models is limited (Robling and Turner, 2009). Capturing modeling and remodeling in response to exercise in humans is even more challenging, with recent improvements as higher resolution technologies become available.
High-Resolution Peripheral Quantitative Computed Tomography (HR-pQCT) has the potential to capture small and subtle changes in human bone (Mancuso and Troy, 2020). HR-pQCT allows for the assessment of bone compartments by assessing three-dimensional volumetric bone mineral density (vBMD) and structural measurements of total, trabecular and cortical bone due to its isotropic voxel size of 61 μm (XtremeCT II) or 82 μm (XtremeCT) (Whittier et al., 2020). In addition to differentiating between skeletal compartments, advanced HR-pQCT software allows for microfinite element analysis (FEA), capable of estimating the mechanical competence of bone (Boutroy et al., 2007). The ability to discern small changes in bone microarchitecture over a short time has the unequivocal potential to determine intervention efficacy within healthy populations and preemptively identify those at unsuspecting risk of injury or fracture. Additionally, early detection of even modest changes in bone microstructure and morphology contribute to large changes in bone strength, even in lieu of changes to bone density or mass (Turner and Robling, 2003; Bass, Eser, and Daly, 2005). Therefore, the purpose of this review is to synthesize existing training-induced HR-pQCT data in three distinct, healthy, adult populations, identify similarities and contrasts with prior modalities and cumulatively interpret results within the scope of bone functional adaptation.
2 Selection of studies
Eligibility was confirmed by full-text screening of papers written in English that utilized the HR-pQCT within a healthy, non-diseased, adult (>18 years) population. Reviews, case-reports, conference proceedings and editorials were excluded. Only studies that included an exercise or training intervention (i.e., non-observational) without the use of pharmacological drugs or nutritional supplementation were included. Consequently, 10 studies were included and were further stratified into 3 groups: 1) Military interventions without supplementation (4 studies), 2) Non-osteoporotic, healthy older adults interventions (3 studies), and 2) General population interventions (3 studies). All studies featured here are also presented in Tables 1, 2.
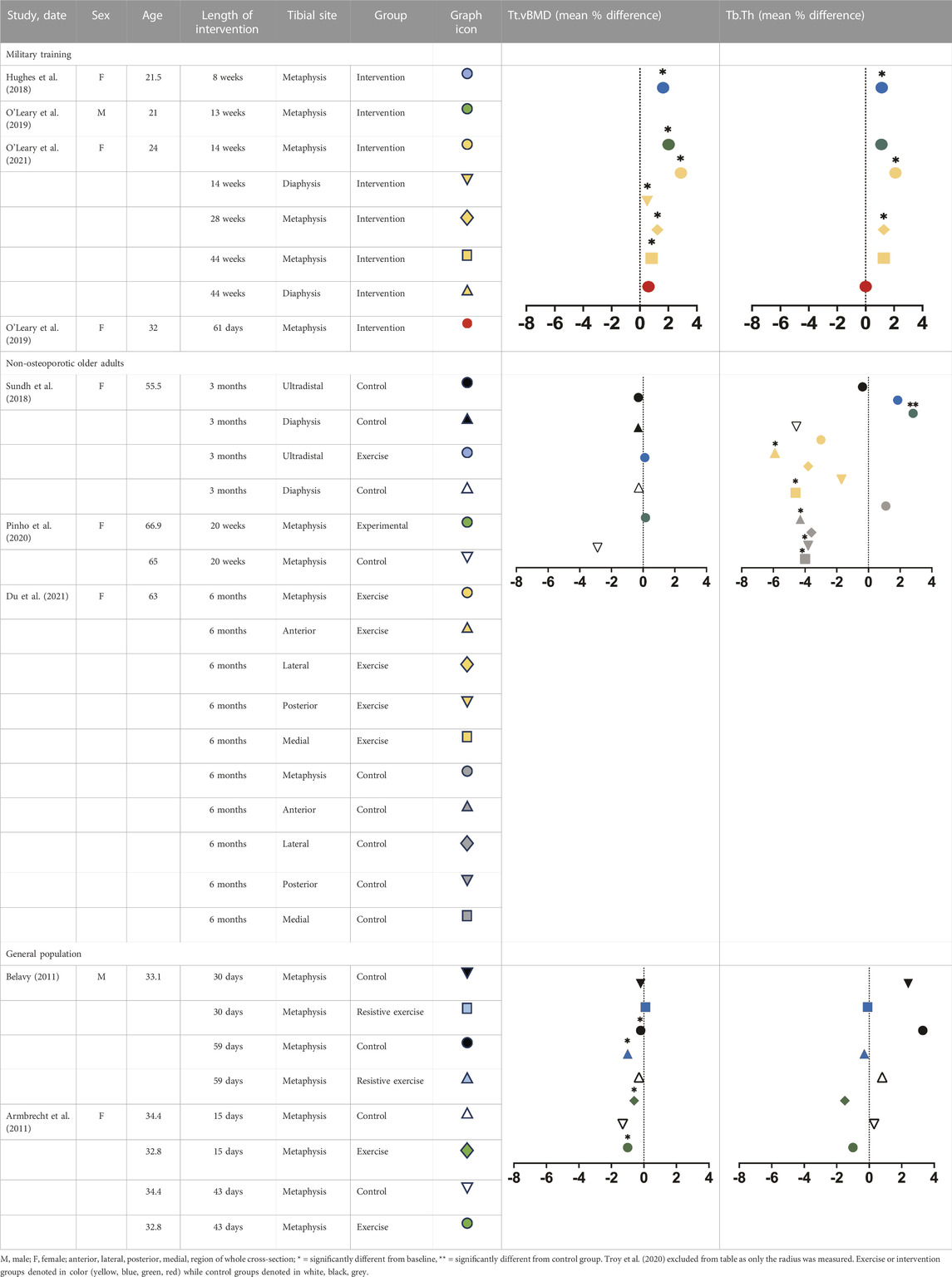
TABLE 1. Mean percent differences in total volumetric bone mineral density and trabecular thickness by group.
3 Military interventions
Perhaps the best evidence we have to support the ability of the HR-pQCT to detect short-term yet meaningful changes in skeletal microarchitecture lies in studies of basic military training (BCT), as these 8–44 weeks programs represents a brief period of often unaccustomed physical activity. Prior to the advent of the HR-pQCT, Peripheral Quantitative Computed Tomography (pQCT) studies demonstrated modest anabolic changes in bone density, geometry and strength in response to short-term (<10 weeks) exercise interventions in male cadets (Izard et al., 2016) and in density, content and cortical microarchitecture in female cadets (Gaffney-Stomberg et al., 2014), though others, demonstrated no changes (Baker et al., 2022). However, pQCT studies have also consistently reported that certain geometric phenotypes (smaller/narrower bone, lower cross-sectional area) predispose military cadets to bone stress injury (Jepsen et al., 2011; Wentz et al., 2011; Davey et al., 2015; O’Leary et al., 2021), though some results have been inconsistent in women(Moran et al., 2008; Cosman et al., 2013). pQCT holds some advantages over HR-pQCT, specifically capturing midshaft and proximal sites and the ability to measure muscle cross sectional area. However, pQCT suffers from partial volume artifacts and lacks the necessary resolution (170 μm) to examine bone microarchitecture (Nishiyama and Shane, 2013). While limited, current HR-pQCT evidence from field training environments demonstrates that short periods of military training (<13 weeks) can elicit favorable adaptations in the tibia, including increased density, size and strength in both women (Hughes et al., 2018) and men (O’Leary et al., 2019a).
In just 8 weeks of Army BCT, 91 female cadets (21.5 ± 3.3 years) experienced a significant increase of approximately 2% in total and trabecular vBMD and trabecular BV/TV at the tibial metaphysis, as well as 1.13%–1.21% increases in trabecular number and thickness (Hughes et al., 2018). Changes however were less robust at the cortical compartment as cortical thickness increased by almost 1% while cortical vBMD and TMD decreased in the tibial metaphysis and diaphysis (Hughes et al., 2018). Stiffness and failure load increased at both sites, conferring much larger magnitude changes at the metaphysis (2.41%–2.45% versus 0.21%–0.77% at diaphysis), though both changes were significant. In 13 weeks of British Army BCT, 43 young men (21 ± 3 years) experienced a significant increase in total (1.7%–2.02%), trabecular (1.3%–1.9%) and cortical density (0.56%–0.9%) as well as Tb BV/TV (1.05%–2.1%), cortical area (2.7%) and cortical thickness (3.1%–3.8%) at the distal tibia. Trabecular area (−0.4%) significantly declined while trabecular number, thickness, spacing, cortical perimeter and porosity did not significantly change (O’Leary et al., 2019a). Longer training (44 weeks) in female Officer cadets demonstrated greater changes in trabecular and cortical microarchitecture at the metaphysis than did shorter training, demonstrating a magnitude of change 1.5–3 fold higher than any previous military study, with the largest changes occurring at the metaphysis in trabecular vBMD (3%), trabecular number (3.5%), cortical thickness (4%) and cortical area (4.8%) (O’Leary et al., 2021).
BCT environments elicit mild energy deficits (Adler et al., 2015; O’Leary et al., 2021). Alternatively, longitudinal assessment of bone microarchitecture under conditions of severe physiological stress are limited. One such example included six healthy British servicewomen (32 ± 3 years) traversing the Antarctic, scanned via HR-pQCT approximately 1 month before the expedition and 2 weeks afterwards. As a result, there were no changes at the tibial metaphysis or diaphysis. The authors reported significant declines in axial aBMD ranging from −2.6% to −5% (O’Leary et al., 2019b) with concurrent preservation of the appendicular skeleton and bone biomarkers indicative of remodeling (O’Leary et al., 2019). Bone resorption, specifically, has been shown to increase with military training (Evans et al., 2008; Lutz et al., 2012; Hughes et al., 2014). HR-pQCT, by neither capturing discernable gains in vBMD nor improvements in microarchitecture, indicated that intense physical activity conducted under multiple stressors (i.e., prolonged severe exercise stress and reduced energy availability) does not favor anabolic formation modeling in women. Aforementioned changes in vBMD and microarchitecture over a short period of time are highly indicative of bone formation modeling, or de novo bone formation (Hughes et al., 2017; Hughes and Bouxsein, 2020) on existing surfaces, without prior resorption. Training-induced decreases in cortical vBMD at both sites also occurred, suggestive of potentially concurrent intracortical targeted remodeling. This suggests that bones can adapt in structure and strength to even brief periods of heightened physical activity in healthy, young adult populations. As many of the military trainees are beyond the age of peak height velocity and residual bone deposition due to growth, these HR-pQCT in military trainees offer evidence that even the mature young adult skeleton retains mechanosensitivity.
3.1 General population interventions
Prior pQCT studies have demonstrated density-centric skeletal adaptations in response to short-term training within general population cohorts. For example, trabecular density increased by approximately 1.34% at the distal tibia in 20-year-old women following just 8 weeks of aerobic (i.e., running based) training, along with a modest improvement in aBMD (Lester et al., 2009). Similarly, trabecular density increased by approximately 0.8%–1.2% in the distal tibia following 13 weeks of combined aerobic and periodized resistance training in young women(R. K. Evans et al., 2012). However, bone properties are only partially explained by bone density as measured by pQCT, as we know that bone microarchitecture is a key determinant of bone’s mechanical competence (Jepsen et al., 2011; Manske et al., 2015) fragility (Burr et al., 1996; Carbonare and Giannini, 2004) and can discriminate those with and without fracture (Legrand et al., 2000; Davey et al., 2015). To date, few studies utilizing the HR-pQCT have been performed in general, non-specialized populations. For example, 102 healthy women (28 ± 6 years) were scanned bilaterally at the standard radius at baseline, 3, 6, 9 and 12 months while undergoing 12 months of 100 cycles of axial force, four times per week. The low stimulus group observed significant increases at 3 (0.39%) and 9 months (0.44%–2.59%) in total and trabecular BMD. The high stimulus group similarly reported increases at 3 (0.29%–2.78%) but a decline at 9 (−0.26%) months in total and trabecular density, indicating formation modeling with increases in cortical thickness at 3 months and perhaps concurrent targeted remodeling specifically with decreases in inner-trabecular vBMD at 9 months (Troy et al., 2020).
Exercise loads the skeleton, in contrast, bedrest—a frequent analog for spaceflight—unloads bone. Bone loss due to situations of disuse typically occurs in bone mass and density in weight-bearing regions, namely, the legs, hip and pelvis (LeBlanc et al., 2000). Twenty four healthy men (31.1 ± 5.1 years) and 24 women (32.8 ± 3.4 years) underwent 60 days of bed rest (60 days) with or without resistive exercise (EXE) 3x per week that targeted the lower extremity (i.e., bilateral squats, back and heel raises) (Armbrecht et al., 2011; Belavý, 2011). Women in the EXE group performed resistance training every 2–3 days and aerobic exercise on a supine treadmill every 3–4 days (Armbrecht et al., 2011). Distal tibia and radial scans were performed prior to, during, and 1–2 years post-bedrest. At the radius, men experienced a slight but significant increase in Ct.vBMD (0.8%), Ct.Ar (1.2%–2.4%) and Ct.Th (1.5%–2.4%) while Tb.Ar progressively worsened (Belavý, 2011). There was no change in trabecular microarchitecture except for Tb.1/N.SD—a measure of inhomogeneity—which decreased significantly by −3.7% 90 days into recovery. At the tibia, density parameters (−1.1% to 0.3%), geometric (−2.0% to 0.3%) and cortical microarchitecture (−2.1% to 0.3%) only modestly changed with the most pronounced changes occurring in trabecular microarchitecture (−3.7% to −3.2%) (Belavý, 2011). There was no evidence of impact from exercise on any skeletal parameter at either site in women (Armbrecht et al., 2011). In men, exercise blunted significant declines in trabecular microarchitecture compared with the control group (Belavý, 2011). However, this occurred only in the tibia, with little to no benefit seen at the radius. Conclusively, exercise during prolonged bedrest was insufficient in counteracting the consequences of disuse. As expected, HR-pQCT parameters at the distal tibia showed greater changes than the distal radius, consistent with weight-bearing sites demonstrating greater sensitivity to inactivity than non-weight-bearing sites. These studies also provided evidence of sex-specific differences in response to disuse as women demonstrated significantly greater losses than did men. A limitation however is that, in disuse-mediated remodeling, deficits preferentially occur at the endocortical region in diaphyseal bone (Hughes and Bouxsein, 2020). Therefore, as only distal sites were studied in both instances, it is plausible that greater skeletal deficits went unnoticed.
3.2 Healthy, non-osteoporotic older adults
Exercise of sufficient load and impact (Petit, Hughes, and Warpeha, 2008) can mitigate bone loss that occurs with aging. While the current diagnosis of osteoporosis is based on areal bone mineral density (aBMD; g/cm2) values, it is been shown that the aBMD in most older adults who experience a fracture is outside of the T-score denoting osteoporotic range (<−2.5) (Mikolajewicz et al., 2020). Additionally, DXA’s measurement of two-dimensional areal bone mineral density (aBMD) is commonly employed as a surrogate measure of bone strength but lacks the resolution to distinguish between cortical and trabecular bone (Harding and Beck, 2017). However, we know from DXA studies that even short-duration exercise interventions can promote bone formation in an aging skeleton. For example, 16 weeks of resistance training in 59–61 year old men resulted in a 2.8%–3.8% increase in femoral neck BMD compared with no changes seen in non-exercising controls (Menkes et al., 1993; Ryan et al., 1994). While aging women tended to need combined training and longer interventions (25 weeks–6 months) to ascertain skeletal benefit (Harding and Beck, 2017), 25 weeks of combined weight lifting and agility training in women aged 75–85 years significantly increased cortical BMD as measured by pQCT at the proximal tibia and radius by 0.5%–1.9% (Liu-Ambrose et al., 2004). With large benefits discernable in older populations with lower resolution technologies, we can anticipate important future findings related to microarchitecture in those that employ HR-pQCT. Few HR-pQCT studies consist of a healthy, non-osteoporotic aging population, none of which include men. Twenty healthy but inactive postmenopausal women (55.5 ± 2.3 years) undergoing 3-months of daily one-legged jumps observed no significant changes in densitometric or geometric parameters, trabecular or cortical microarchitecture or mechanical strength at the distal or ultradistal tibial (Sundh et al., 2018). Similarly, 20 weeks (5 months) of high-impact exercises in 38 osteopenic women aged 60–70 years, elicited only modest responses including a −0.8% reduction in Tb.vBMD and 2.8% increase in Tb.Th at the tibial metaphysis (Pinho et al., 2020). A 6-month unilateral hopping protocol significantly increased trabecular microarchitecture, specifically at the global—whole cross section—and anterior region with a 4.4% increase globally and 6.1% increase in BV/TV anteriorly, but no significant change in mechanical strength at the tibial metaphysis (Du et al., 2021). While more diverse research is still needed, HR-pQCT studies confirm that favorable anabolic changes to the distal tibia in <6 months are possible in a senescent skeleton.
4 Discussion
Following short-term training (8–13 weeks) robust changes are observed during military training with sex-specific differences in bone adaptation. Military initial level training involves a drastic and sudden increase in both volume and frequency of weight bearing activities such as unaccustomed load carriage and military drill, during which the lower extremities experience intense loading (O’Leary et al., 2021). Greater sensitivity to heightened mechanical loading consistently occurred distally, despite animal studies demonstrating that long bone adaptations in response to mechanical loading tends to occur at the midshaft and more proximally (Warden et al., 2004; Wallace et al., 2007). This discrepancy could be the result of limited scan breadth on HR-pQCT lending to best capturing only the distal appendicular skeleton (Lieberman et al., 2003; Pearson and Lieberman, 2004). However, the second generation HR-pQCT allows for expansion of the diaphyseal region to include up to 66% of total tibial length. Although to date, no training-specific studies have utilized this methodology, future longitudinal capture at this site will allow for further elucidation of cortical bone, muscle and fat in response to exercise or training (Whittier et al., 2020). Longer training periods in female Officer cadets demonstrated greater changes in trabecular and cortical microarchitecture at the metaphysis than did shorter training, a magnitude of change 1.5-3 fold higher than any prior military study (O’Leary et al., 2021). Maintenance of whole body aBMD in conjunction with these high magnitude adaptations implies that unaccustomed mechanical loading was protective against periodic low energy availability(Gifford et al., 2021) despite significant changes in total body, fat and lean mass(O’Leary et al., 2021). Further research is needed to further elucidate factors promoting versus preventing adaptation, from which we’ll discover new risk factors (i.e., energy availability, psychological stress, sleep, NSAIDs) (Hughes et al., 2022) for bone stress injury, stress fracture and bone fragility.
Within the general population, healthy adult women undergoing 12 months of compressive loading of the forearm showed significant increases in BMC associated with strain ranges experienced during activities of daily living (Troy et al., 2020). This finding conflicts with the previously held belief that extremely high strain magnitudes were necessary to elicit an adaptive response, specifically in women (Martyn-St James and Carroll, 2010). In the absence of loading, men demonstrated a pattern of bone recovery after disuse most pronounced at the distal tibia with recovery of cortical bone (namely, Ct.Ar and Ct.Th) beginning as early as 3 days after bedrest (Belavý, 2011). Women, however, demonstrated the greatest loss in Ct.Th at the distal tibia, despite additional protein supplementation (Armbrecht et al., 2011). These findings demonstrate sex-specific differences in skeletal recovery following disuse, such that men require substantially less time than do women for recovery of bone structure and density (Armbrecht et al., 2011).
Drawing sex-specific conclusions in aging populations remains difficult, as few HR-pQCT studies exist with healthy, aging populations and those that do, include exclusively women. However, existing research demonstrates that bone mechanosensitivity remains viable in women well into their 6th decade of life with changes favoring the distal trabecular compartment. Cumulatively, these results demonstrate that age may not be the decisive factor in whether or not an adaptive response occurs, but instead the length of the intervention (>6 months), type of stimulus (multidirectional, high impact) and granularity of the imaging technique (regional analysis). There remains work to be done to establish the effectiveness of exercise not just in preventing bone loss but encouraging anabolism into old age. The exercise-specific characteristics and environmental considerations that will promote these processes have yet to be fully elucidated and captured with HR-pQCT technology.
Introduction of the HR-pQCT has provided researchers the ability to investigate bone microarchitecture in vivo and quantify densitometric, morphological and geometric adaptation in response to exercise and training exposure. While pharmaceutical interventions have shown efficacy, they are often contraindicated until late in life. Non-pharmacological, non-invasive training intervention studies provide insight into the optimal exercise program to enhance bone strength and reduce susceptibility to bone injury, stress and osteoporotic fracture. Ultimately, the utilization of highly sensitive bone imaging modalities like the HR-pQCT maximizes the ability to detect even modest change that may, in turn, manifest into substantial effects over longer periods of time. Promising horizons are ahead for the role of exercise to promote anabolism and bone strength across the lifespan.
Author contributions
NS: Conceptualization, Data curation, Investigation, Methodology, Writing–original draft, Writing–review and editing. JH: Data curation, Software, Supervision, Visualization, Writing–review and editing. AS: Writing–review and editing. KM: Data curation, Visualization, Writing–review and editing. ML: Resources, Supervision, Writing–review and editing. JC: Supervision, Writing–review and editing. JG: Funding acquisition, Methodology, Software, Supervision, Visualization, Writing–review and editing. BN: Conceptualization, Data curation, Funding acquisition, Methodology, Software, Supervision, Writing–review and editing.
Funding
The author(s) declare financial support was received for the research, authorship, and/or publication of this article. This research was supported by the UK Ministry of Defence (WGCC 5.5.6-Task 0107).
Conflict of interest
The authors declare that the research was conducted in the absence of any commercial or financial relationships that could be construed as a potential conflict of interest.
Publisher’s note
All claims expressed in this article are solely those of the authors and do not necessarily represent those of their affiliated organizations, or those of the publisher, the editors and the reviewers. Any product that may be evaluated in this article, or claim that may be made by its manufacturer, is not guaranteed or endorsed by the publisher.
References
Adler A. B., Williams J., McGurk D., Moss A., Bliese P. D. (2015). Resilience training with soldiers during basic combat training: randomisation by platoon. Appl. Psychol. Health Well-Being 7 (1), 85–107. doi:10.1111/aphw.12040
Armbrecht G., Ludovic Belavý D., Backström M., Beller G., Alexandre C., Rizzoli R., et al. (2011). Trabecular and cortical bone density and architecture in women after 60 Days of bed rest using high-resolution pQCT: WISE 2005. J. Bone Mineral Res. 26 (10), 2399–2410. doi:10.1002/jbmr.482
Ashton R. E., Tew G. A., Aning J. J., Gilbert S. E., Lewis L., Saxton J. M. (2020). Effects of short-term, medium-term and long-term resistance exercise training on cardiometabolic health outcomes in adults: systematic review with meta-analysis. Br. J. Sports Med. 54 (6), 341–348. doi:10.1136/bjsports-2017-098970
Baker B. S., Buchanan S. R., Black C. D., Bemben M. G., Bemben D. A. (2022). Bone, biomarker, body composition, and performance responses to 8 Weeks of reserve officers’ training corps training. J. Athl. Train. 57 (6), 571–580. doi:10.4085/1062-6050-0634.20
Bass S. L., Eser P., Daly R. (2005). The effect of exercise and nutrition on the mechanostat. J. Musculoskelet. Neuronal Interact. 5 (3), 239–254.
Belavý D. L., Beller G., Ritter Z., Felsenberg D. (2011). Bone structure and density via HR-pQCT in 60d bed-rest, 2-years recovery with and without countermeasures. J. Musculoskelet. Neuronal Interact. 11 (3), 215–226.
Bentolila V., Boyce T. M., Fyhrie D. P., Drumb R., Skerry T. M., Schaffler M. B. (1998). Intracortical remodeling in adult rat long bones after fatigue loading. Bone 23 (3), 275–281. doi:10.1016/S8756-3282(98)00104-5
Boivin G., Farlay D., Bala Y., Doublier A., Meunier P. J., Delmas P. D. (2009). Influence of remodeling on the mineralization of bone tissue. Osteoporos. Int. 20 (6), 1023–1026. doi:10.1007/s00198-009-0861-x
Boutroy S., Van Rietbergen B., Sornay-Rendu E., Munoz F., Bouxsein M. L., Delmas P. D. (2007). Finite element analysis based on in vivo HR-pQCT images of the distal radius is associated with wrist fracture in postmenopausal women. J. Bone Mineral Res. 23 (3), 392–399. doi:10.1359/jbmr.071108
Burr D. B., Milgrom C., Fyhrie D., Forwood M., Nyska M., Finestone A., et al. (1996). In vivo measurement of human tibial strains during vigorous activity. Bone 18 (5), 405–410. doi:10.1016/8756-3282(96)00028-2
Carbonare L. D., Giannini S. (2004). Bone microarchitecture as an important determinant of bone strength. J. Endocrinol. Investigation 27 (1), 99–105. doi:10.1007/BF03350919
Cosman F., Ruffing J., Zion M., Uhorchak J., Ralston S., Tendy S., et al. (2013). Determinants of stress fracture risk in United States military academy cadets. Bone 55 (2), 359–366. doi:10.1016/j.bone.2013.04.011
Davey T., Lanham-New S. A., Shaw A. M., Cobley R., Allsopp A. J., Mark O., et al. (2015). Fundamental differences in axial and appendicular bone density in stress fractured and uninjured royal marine recruits — a matched case–control study. Bone 73 (4), 120–126. doi:10.1016/j.bone.2014.12.018
Du J., Hartley C., Brooke-Wavell K., Paggiosi M. A., Walsh J. S., Li S., et al. (2021). High-impact exercise stimulated localised adaptation of microarchitecture across distal tibia in postmenopausal women. Osteoporos. Int. 32 (5), 907–919. doi:10.1007/s00198-020-05714-4
Eriksen E. F. (2010). Cellular mechanisms of bone remodeling. Rev. Endocr. Metabolic Disord. 11 (4), 219–227. doi:10.1007/s11154-010-9153-1
Evans R. K., Antczak A. J., Lester M., Ran Y., Israeli E., Daniel S. (2008). Effects of a 4-month recruit training program on markers of bone metabolism. Med. Sci. Sports Exerc. 40 (11), S660–S670. doi:10.1249/MSS.0b013e318189422b
Evans R. K., Negus C. H., Centi A. J., Spiering B. A., Kraemer W. J., Nindl B. C. (2012). Peripheral QCT sector analysis reveals early exercise-induced increases in tibial bone mineral density. J. Musculoskelet. Neuronal Interact. 12 (3), 155–164.
Frost H. M. (2003). Bone’s mechanostat: a 2003 update. Anatomical Rec. 275A (2), 1081–1101. doi:10.1002/ar.a.10119
Frost H. M. (1987). Bone mass and the mechanostat: a proposal. Anatomical Rec. 219 (1), 1–9. doi:10.1002/ar.1092190104
Gaffney-Stomberg E., Lutz L. J., Rood J. C., Cable S. J., Pasiakos S. M., Young A. J., et al. (2014). Calcium and vitamin D supplementation maintains parathyroid hormone and improves bone density during initial military training: a randomized, double-blind, placebo controlled trial. Bone 68 (11), 46–56. doi:10.1016/j.bone.2014.08.002
Gifford R. M., Greeves J. P., Wardle S. L., O’Leary T. J., Double R. L., Venables M., et al. (2021). Measuring the exercise component of energy availability during arduous training in women. Med. Sci. Sports Exerc. 53 (4), 860–868. doi:10.1249/MSS.0000000000002527
Harding A., Beck B. (2017). Exercise, osteoporosis, and bone geometry. Sports 5 (2), 29. doi:10.3390/sports5020029
Hecksteden A., Oliver F., Meyer T., Donath L. (2018). How to construct, conduct and analyze an exercise training study? Front. Physiology 9 (7), 1007. doi:10.3389/fphys.2018.01007
Hughes J. M., Smith M. A., Henning P. C., Scofield D. E., Spiering B. A., Staab J. S., et al. (2014). Bone Formation is suppressed with multi-stressor military training. Eur. J. Appl. Physiology 114 (11), 2251–2259. doi:10.1007/s00421-014-2950-6
Hughes J. M., Popp K. L., Ran Y., Bouxsein M. L., Matheny R. W. (2017). The role of adaptive bone formation in the etiology of stress fracture. Exp. Biol. Med. 242 (9), 897–906. doi:10.1177/1535370216661646
Hughes J. M., Castellani C. M., Kristin L., Guerriere K. I., Matheny R. W., Nindl B. C., et al. (2020). The central role of osteocytes in the four adaptive pathways of bone’s mechanostat. Exerc. Sport Sci. Rev. 48 (3), 140–148. doi:10.1249/JES.0000000000000225
Hughes J. M., Gaffney-Stomberg E., Guerriere K. I., Taylor K. M., Popp K. L., Xu C., et al. (2018). Changes in tibial bone microarchitecture in female recruits in response to 8 Weeks of U.S. Army basic combat training. Bone 113 (8), 9–16. doi:10.1016/j.bone.2018.04.021
Hughes J. M., O’Leary T. J., Koltun K. J., Greeves J. P. (2022). Promoting adaptive bone formation to prevent stress fractures in military personnel. Eur. J. Sport Sci. 22 (1), 4–15. doi:10.1080/17461391.2021.1949637
Izard R. M., Fraser W. D., Negus C., Craig S., JulieGreeves P. (2016). Increased density and periosteal expansion of the tibia in young adult men following short-term arduous training. Bone 88 (7), 13–19. doi:10.1016/j.bone.2016.03.015
Jepsen K. J., Centi A., Duarte G. F., Galloway K., Goldman H., Hampson N., et al. (2011). Biological constraints that limit compensation of a common skeletal trait variant lead to inequivalence of tibial function among healthy young adults. J. Bone Mineral Res. 26 (12), 2872–2885. doi:10.1002/jbmr.497
Jepsen K. J. (2009). Systems analysis of bone. WIREs Syst. Biol. Med. 1 (1), 73–88. doi:10.1002/wsbm.15
LeBlanc A., Lin C., Shackelford L., Valentine S., Evans H., Belichenko O., et al. (2000). Muscle volume, MRI relaxation times (T2), and body composition after spaceflight. J. Appl. Physiology 89 (6), 2158–2164. doi:10.1152/jappl.2000.89.6.2158
Legrand E., Chappard D., Pascaretti C., Duquenne M., Krebs S., Rohmer V., et al. (2000). Trabecular bone microarchitecture, bone mineral density, and vertebral fractures in male osteoporosis. J. Bone Mineral Res. 15 (1), 13–19. doi:10.1359/jbmr.2000.15.1.13
Lester M. E., Urso M. L., Evans R. K., Pierce J. R., Spiering B. A., Maresh C. M., et al. (2009). Influence of exercise mode and osteogenic index on bone biomarker responses during short-term physical training. Bone 45 (4), 768–776. doi:10.1016/j.bone.2009.06.001
Lieberman D. E., Pearson O. M., Polk J. D., Demes B., Crompton A. W. (2003). Optimization of bone growth and remodeling in response to loading in tapered mammalian limbs. J. Exp. Biol. 206 (18), 3125–3138. doi:10.1242/jeb.00514
Linke S. E., Gallo L. C., Norman G. J. (2011). Attrition and adherence rates of sustained vs. Intermittent exercise interventions. Ann. Behav. Med. 42 (2), 197–209. doi:10.1007/s12160-011-9279-8
Liu-Ambrose T. Y. L., Khan K. M., Ari Heinonen J. J. E., McKay H. A. (2004). Both resistance and agility training increase cortical bone density in 75- to 85-year-old women with low bone mass: a 6-month randomized controlled trial. J. Clin. Densitom. 7 (4), 390–398. doi:10.1385/JCD:7:4:390
Lutz L. J., Karl J. P., Rood J. C., J Cable S., Williams K. W., Young A. J., et al. (2012). Vitamin D status, dietary intake, and bone turnover in female soldiers during military training: a longitudinal study. J. Int. Soc. Sports Nutr. 9 (1), 38. doi:10.1186/1550-2783-9-38
Mancuso M. E., Wilzman A. R., Murdock K. E., Troy K. L. (2022). Effect of external mechanical stimuli on human bone: a narrative review. Prog. Biomed. Eng. 4 (1), 012006. doi:10.1088/2516-1091/ac41bc
Mancuso M. E., Troy K. L. (2020). Relating bone strain to local changes in radius microstructure following 12 Months of axial forearm loading in women. J. Biomechanical Eng. 142 (11), 111014. doi:10.1115/1.4048232
Manske S. L., Zhu Y., Sandino C., Boyd S. K. (2015). Human trabecular bone microarchitecture can Be assessed independently of density with second generation HR-pQCT. Bone 79 (10), 213–221. doi:10.1016/j.bone.2015.06.006
Martyn-St James M., Carroll S. (2010). Effects of different impact exercise modalities on bone mineral density in premenopausal women: a meta-analysis. J. Bone Mineral Metabolism 28 (3), 251–267. doi:10.1007/s00774-009-0139-6
Menkes A., Mazel S., Redmond R. A., Koffler K., Libanati C. R., Gundberg C. M., et al. (1993). Strength training increases regional bone mineral density and bone remodeling in middle-aged and older men. J. Appl. Physiology 74 (5), 2478–2484. doi:10.1152/jappl.1993.74.5.2478
Mikolajewicz N., Bishop N., Burghardt A. J., Folkestad L., Hall A., Kozloff K. M., et al. (2020). HR-pQCT measures of bone microarchitecture predict fracture: systematic review and meta-analysis. J. Bone Mineral Res. 35 (3), 446–459. doi:10.1002/jbmr.3901
Moran D. S., Israeli E., Evans R. K., Yanovich R., Constantini N., Shabshin N., et al. (2008). Prediction model for stress fracture in young female recruits during basic training. Med. Sci. Sports Exerc. 40 (11), S636–S644. doi:10.1249/MSS.0b013e3181893164
Morgan E. F., Unnikrisnan G. U., Hussein A. I. (2018). Bone mechanical properties in healthy and diseased states. Annu. Rev. Biomed. Eng. 20 (1), 119–143. doi:10.1146/annurev-bioeng-062117-121139
Mustafy T., Londono I., Moldovan F., Villemure I. (2019). High impact exercise improves bone microstructure and strength in growing rats. Sci. Rep. 9 (1), 13128. doi:10.1038/s41598-019-49432-2
Nishiyama K. K., Shane. E. (2013). Clinical imaging of bone microarchitecture with HR-pQCT. Curr. Osteoporos. Rep. 11 (2), 147–155. doi:10.1007/s11914-013-0142-7
O'Leary T. J., Izard R. M., Walsh N. P., Tang J. C. Y., Fraser W. D., Greeves J. P. (2019a). Skeletal macro- and microstructure adaptations in men undergoing arduous military training. Bone 125 (8), 54–60. doi:10.1016/j.bone.2019.05.009
O'Leary T. J., Gifford R. M., Double R. L., Reynolds R. M., Woods D. R., Wardle S. L., et al. (2019b). Skeletal responses to an all-female unassisted antarctic traverse. Bone 121 (4), 267–276. doi:10.1016/j.bone.2019.02.002
O’Leary T. J., Rice H. M., Greeves J. P. (2021a). Biomechanical basis of predicting and preventing lower limb stress fractures during arduous training. Curr. Osteoporos. Rep. 19 (3), 308–317. doi:10.1007/s11914-021-00671-1
O’Leary T. J., Walsh N. P., Casey A., Izard R. M., Tang J. C. Y., Fraser W. D., et al. (2021b). Supplementary energy increases bone formation during arduous military training. Med. Sci. Sports Exerc. 53 (2), 394–403. doi:10.1249/MSS.0000000000002473
O’Leary T. J., Wardle S. L., Gifford R. M., Double R. L., Reynolds R. M., Woods D. R., et al. (2021c). Tibial macrostructure and microarchitecture adaptations in women during 44 Weeks of arduous military training. J. Bone Mineral Res. 36 (7), 1300–1315. doi:10.1002/jbmr.4290
Pearson O. M., Lieberman D. E. (2004). The aging of Wolff’s ?law? ontogeny and responses to mechanical loading in cortical bone. Am. J. Phys. Anthropol. 125 (S39), 63–99. doi:10.1002/ajpa.20155
Petit M. A., Hughes J. M., Joseph W. (2008). “Exercise prescription for people with osteoporosis,” in Resource manual for guidelines for exercise testing and prescription, 1–16.
Pinho J. P., Forner-Cordero A., Rodrigues Pereira R. M., Jose Hernandez A., Lima Dorea E., et al. (2020). A high-intensity exercise intervention improves older women lumbar spine and distal tibia bone microstructure and function: a 20-week randomized controlled trial. IEEE J. Transl. Eng. Health Med. 8, 2100108–8. doi:10.1109/JTEHM.2019.2963189
Plotkin L. I. (2014). Apoptotic osteocytes and the control of targeted bone resorption. Curr. Osteoporos. Rep. 12 (1), 121–126. doi:10.1007/s11914-014-0194-3
Robling A. G., Turner. C. H. (2009). Mechanical signaling for bone modeling and remodeling. Crit. Rev. Eukaryot. Gene Expr. 19 (4), 319–338. doi:10.1615/CritRevEukarGeneExpr.v19.i4.50
Ryan A. S., Treuth M. S., Rubin M. A., Miller J. P., Nicklas B. J., Landis D. M., et al. (1994). Effects of strength training on bone mineral density: hormonal and bone turnover relationships. J. Appl. Physiology 77 (4), 1678–1684. doi:10.1152/jappl.1994.77.4.1678
Seeman E. (2002). Pathogenesis of bone fragility in women and men. Lancet 359 (9320), 1841–1850. doi:10.1016/S0140-6736(02)08706-8
Sundh D., Nilsson M., Zoulakis M., Pasco C., Yilmaz M., Kazakia G. J., et al. (2018). High-impact mechanical loading increases bone material strength in postmenopausal women-A 3-month intervention study: high-impact mechanical loading increases bmsi. J. Bone Mineral Res. 33 (7), 1242–1251. doi:10.1002/jbmr.3431
Troy K. L., Mancuso M. E., Johnson J. E., Wu Z., Thomas J. S., Butler T. A. (2020). Bone adaptation in adult women is related to loading dose: a 12-month randomized controlled trial. J. Bone Mineral Res. 35 (7), 1300–1312. doi:10.1002/jbmr.3999
Turner C. H., Robling A. G. (2003). Designing exercise regimens to increase bone strength. Exerc. Sport Sci. Rev. 31 (1), 45–50. doi:10.1097/00003677-200301000-00009
Wallace J. M., Rajachar R. M., Allen M. R., Bloomfield S. A., Robey P. G., Young M. F., et al. (2007). Exercise-induced changes in the cortical bone of growing mice are bone- and gender-specific. Bone 40 (4), 1120–1127. doi:10.1016/j.bone.2006.12.002
Warden S. J., Hurst J. A., Sanders M. S., Turner C. H., Burr D. B., Li J. (2004). Bone adaptation to a mechanical loading program significantly increases skeletal fatigue resistance. J. Bone Mineral Res. 20 (5), 809–816. doi:10.1359/JBMR.041222
Welch J. M., Turner C. H., Devareddy L., Arjmandi B. H., Weaver C. M. (2008). High impact exercise is more beneficial than dietary calcium for building bone strength in the growing rat skeleton. Bone 42 (4), 660–668. doi:10.1016/j.bone.2007.12.220
Wentz L., Liu P.-Y., Haymes E., JasminkaIlich Z. (2011). Females have a greater incidence of stress fractures than males in both military and athletic populations: a systemic review. Mil. Med. 176 (4), 420–430. doi:10.7205/MILMED-D-10-00322
Whittier D. E., Boyd S. K., Burghardt A. J., Paccou J., Ghasem-Zadeh A., Chapurlat R., et al. (2020). Guidelines for the assessment of bone density and microarchitecture in vivo using high-resolution peripheral quantitative computed tomography. Osteoporos. Int. 31 (9), 1607–1627. doi:10.1007/s00198-020-05438-5
Wolff J. (1986). The law of bone remodelling. Berlin, Heidelberg: Springer Berlin Heidelberg. doi:10.1007/978-3-642-71031-5
Nomenclature
Keywords: high-resolution pQCT, X-ray, imaging, exercise training, short-term changes
Citation: Sekel NM, Hughes JM, Sterczala AJ, Mroz KH, Lovalekar M, Cauley J, Greeves JP and Nindl BC (2023) Utility of HR-pQCT in detecting training-induced changes in healthy adult bone morphology and microstructure. Front. Physiol. 14:1266292. doi: 10.3389/fphys.2023.1266292
Received: 02 August 2023; Accepted: 03 October 2023;
Published: 20 October 2023.
Edited by:
Udo Jochen Birk, University of Applied Sciences Graubünden, SwitzerlandReviewed by:
Timothy Ryan, The Pennsylvania State University (PSU), United StatesCopyright © 2023 Sekel, Hughes, Sterczala, Mroz, Lovalekar, Cauley, Greeves and Nindl. This is an open-access article distributed under the terms of the Creative Commons Attribution License (CC BY). The use, distribution or reproduction in other forums is permitted, provided the original author(s) and the copyright owner(s) are credited and that the original publication in this journal is cited, in accordance with accepted academic practice. No use, distribution or reproduction is permitted which does not comply with these terms.
*Correspondence: Nicole M. Sekel, bmlzMTIzQHBpdHQuZWR1