- 1Fundación Oceanografic de la Comunidad Valenciana, Gran Vía Marques del Turia 19, Valencia, Spain
- 2Kolmården Wildlife Park, Kolmården, Sweden
- 3Global Diving Research SL, Valencia, Spain
- 4Sea Mammal Research Unit, Scotland, United Kingdom
- 5Duke University Marine Laboratory, Nicholas School of the Environment Duke University, Beaufort, NC, United States
- 6Dolphin Quest, Kahala Resort, Waikoloa, HI, United States
- 7Institut de Génomique Fonctionnelle, Université de Montpellier, CNRS, INSERM, Montpellier, France
- 8Department of Marine Bioscience, Atmosphere and OceanResearch Institute, The University of Tokyo, Chiba, Japan
Introduction: The bottlenose dolphin (Tursiops truncatus) is an intermittent breather, where the breath begins with an exhalation followed by inhalation and an extended inter-breath interval ranging from 10 to 40 s. Breathing has been shown to alter both the instantaneous heart rate (ifH) and stroke volume (iSV) in the bottlenose dolphin, with a transitory ventilatory tachycardia following the breath, and an exponential decrease to a stable ifH around 40 beats • min−1 during the inter-breath period. As the total breath duration in the dolphin is around 1 s, it is not possible to assess the contribution of exhalation and inhalation to these changes in cardiac function during normal breathing.
Methods: In the current study, we evaluated the ifH response by separating expiration and inspiration of a breath, which allowed us to distinguish their respective contribution to the changes in ifH. We studied 3 individual male bottlenose dolphins trained to hold their breath between the different respiratory phases (expiration and inhalation).
Results: Our data show that inspiration causes an increase in ifH, while expiration appears to result in a decrease in ifH.
Discussion: These data provide improved understanding of the cardiorespiratory coupling in dolphins, and show how both exhalation and inhalation alters ifH.
Introduction
Cardiorespiratory coupling, where breathing results in variation in the instantaneous heart rate (ifH), has been reported in seals and cetaceans (Irving, 1939; Ridgway, 1972; Kooyman, 1985; Kaczmarek et al., 2018; Fahlman et al., 2020b; Blawas et al., 2021b). In the bottlenose dolphin (Tursiops truncatus), the intermittent breathing pattern begins with a rapid exhalation followed by inhalation with a breath duration of approximately 1 s (Fahlman et al., 2017). The end of the breath, i.e., end-inspiration, results in an initial increase in ifH followed by a slow decline to a heart rate between 35 and 50 beats • min−1 (Cauture et al., 2019; Fahlman et al., 2020b; Blawas et al., 2021a). During the inter-breath period there is often a sinusoidal variation in ifH with a period of varying duration of between 4 and 10 s (Cauture et al., 2019; Blawas et al., 2021a). The magnitude of this respiratory tachycardia varies both with changes in breathing frequency (fR) and tidal volume (VT) (Cauture et al., 2019; Blawas et al., 2021a; Blawas et al., 2021b). However, the respiratory characteristics of the bottlenose dolphin, with high respiratory flow during exhalation and a breath duration that lasts one or two heartbeat cycles, makes it difficult to separately evaluate how exhalation and inhalation affect cardiac function.
To improve our understanding of the ventilatory tachycardia, and to assess the variation in ifH during the exhalation and inspiration phases in bottlenose dolphins, we measured electrocardiograms (ECGs) using a data logger with suction cup-embedded electrodes and respiratory flow using a custom-built pneumotachometer during both normal breaths and breaths where exhalation and inhalation were separated. Our results provide evidence that normal breaths result in a respiratory tachycardia, and that exhalation and inhalation, respectively, result in a decrease and increase in ifH. Thus, the cardiac variability in bottlenose dolphins associated with respiration occurs at their typical breathing frequencies between 0.025 and 0.1 Hz. The results also highlight a second frequency of cardiac variability during breathing in the range of 0.1–0.2 Hz, possibly associated with blood pressure regulation. The data presented in the current study imply that both exhalation and inhalation alter ifH, and that breathing frequency alters cardiac function and heart rate.
Materials and methods
Animal information
Three male bottlenose dolphins housed in managed care were studied for this work (Table 1). Individual animal ID, body mass, and year of birth are summarized in Table 1. Dolphins were not restrained and could refuse to participate or withdraw at any point during the experimental trial. Before data collection, animals had been desensitized to the equipment and trained for novel research-associated behaviours using operant conditioning (Fahlman et al., 2015; Fahlman et al., 2020b). These individual animals had participated in similar cardiorespiratory trials on numerous occasions since 2015 (Fahlman et al., 2015; Fahlman et al., 2019a; Fahlman et al., 2019b; Cauture et al., 2019; Fahlman et al., 2020b; Blawas et al., 2021a).

TABLE 1. Animal identification (ID), body mass (Mb), year of birth (YOB), number of breaths analyzed (N), and mean instantaneous heart rate (fH) for pre- and post-breaths, with either complete breaths (Full, exhalation followed by immediate inhalation), or either exhalation (Ex) or inhalation (In). The uneven number between the number of exhalations and inhalations was due to exhalation followed by inhalations outside the pneumotachometer (i.e., flow not measured, 63H4 and 90N6), or repeated shallow exhalations before a full inspiration (9FL3).
Research trials
During each trial, the dolphin remained stationary in the water, allowing placement of the ECG data logger on the chest (ECG400-DT, Little Leonardo co.) and placement of the pneumotachometer over the blow-hole (Fahlman et al., 2015; Aoki et al., 2021).
The dolphins performed two types of trials for data collection. During normal breathing, an exhalation was followed by an immediate inhalation. During an exhale hold, a single exhalation was followed by an inter-breath interval of between 5 and 45 s, followed by inhalation. In one dolphin, several exhalation signals were given, which resulted in repeated exhalations before inhalation, i.e., repeated exhale hold. These breathing manoeuvres were repeated during a single session and multiple sessions were repeated for each animal over several days. One individual was trained to perform repeated exhales before inhalation with a respiratory pause between 3 and 5 s.
The dolphins were not fasted, but where inactive before, for between 2 and 5 min, and during each trial, which lasted between 5 and 10 min. All trials were conducted between 7 and 10 November 2022, either in the morning or afternoon.
Measurements
We analyzed the ifH 1–3 s before (pre) and after (post) a full breath (Full), exhalation (Ex), or inhalation (In). We did this to assess the immediate cardiovascular response caused by ventilation, and due to limitations in obtaining extended exhalation holds. The relative change in ifH (%) before and after each respiratory phase was analyzed as (post-pre) • pre−1 • 100. Thus, a positive or negative change is, respectively, an increase or decrease in ifH following a breathing phase.
The respiratory timing was measured using a custom-made pneumotachometer, as previously detailed (Fahlman et al., 2015; Fahlman et al., 2020a), but briefly summarized below. Respiratory flows were measured using a custom-made Fleisch type pneumotachometer (Mellow Design, Valencia, Spain), which housed a low-resistance laminar flow matrix (Item #Z9A887-2, Merriam Process Technologies, Cleveland, OH). A differential pressure transducer (Spirometer Pod, ML 311, ADInstruments, Colorado Springs, CO) was connected to the pneumotachometer with two, 310 cm lengths of 2 mm I.D., firm walled, flexible tubing. The differential pressure transducer was connected to a data acquisition system (Powerlab 8/35, ADInstruments, Colorado Springs, CO), and the data were captured at 400 Hz and displayed on a computer running LabChart (v. 8.1, ADInstruments, Colorado Springs, CO). The respiratory flow was used to determine the beginning and end of each exhalation and inhalation for a total of 117 respiratory events (Table 1). The beginning and end of a respiratory phase were used as time = 0 for the averaging of the heart rate. For example, for a Full breath time = 0 for the pre data was at the beginning of the exhalation, while for post time = 0 was the end of the inhalation.
The respiratory flow and heart rate signals were synchronized by aligning start and end times of data collection.
Statistical analysis
We used a general linear mixed-effects (GLM) model with nested random effects of individual to account for differences between animals. Models were fitted in the R statistical computing software (R Core Team, 2021; RStudio Team, 2021) using the nlme package (Pinheiro et al., 2021).
Results
Figure 1 shows the ifH following Full breaths, with panel A) showing the response during repeated Full breaths, and panel B) following a single Full breath followed by an exhalation. The most parsimonious model showed that ifH increased by 19.9% following a Full breath (Eq. 1; Table 1; Figure 1, df = 2, χ2 = 84.2). When breaths were separated into exhalation and inhalation, Ex resulted in a 33.5% decrease in ifH. In resulted in an additional 20.1% increase in ifH (Eq. 1; Table 1; Figures 2, 3), similar to what we recorded during a normal breathing cycle.
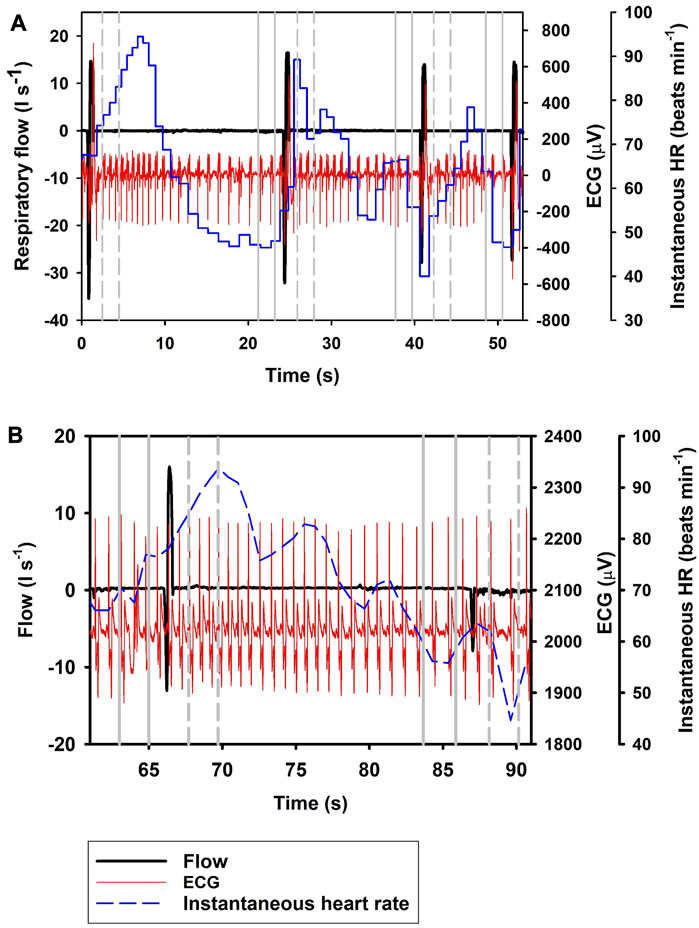
FIGURE 1. Respiratory flow, raw ECG, and instantaneous heart rate (HR) during (A) four single full (Full; t1 = 0.7 s, t2 = 25 s, t3 = 41 s, t4 = 52 s) breaths, and (B) a single full breath (t = 67 s) with a 20 s interbeath interval until the next exhalation (Ex; t = 88 s). Vertical lines show section used for analysis, with solid gray line indicating pre- and broken gray line post-breath.
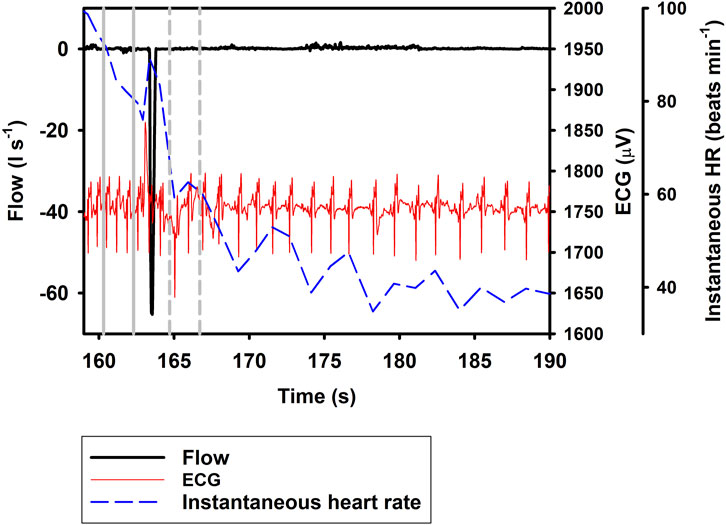
FIGURE 2. Respiratory flow, raw ECG, and instantaneous heart rate (HR) during a single exhalation (Ex; t = 164 s). Vertical lines show section used for analysis, with solid gray line indicating pre- and broken gray line post-breath.
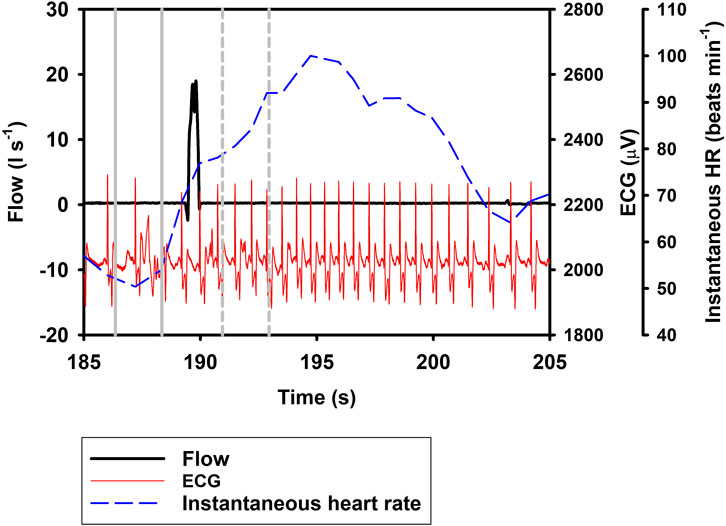
FIGURE 3. Respiratory flow, raw ECG, and instantaneous heart rate (HR) during a single inhalation (In; t = 190 s). Vertical lines show section used for analysis, with solid gray line indicating pre- and broken gray line post-breath.
For repeated Ex, the decrease in ifH was not affected by the number of repeated exhalations (df = 1, Loglikelihood = 447.4, χ2 = 0.03).
Discussion
In the current study, we show a respiratory tachycardia associated with normal breaths in the bottlenose dolphin, similar to what has been previously reported in this species and also other small to medium sized cetaceans (Irving, 1939; Ridgway, 1972; Fahlman et al., 2020b; Blawas et al., 2021b). We also report that when exhalation and inhalation are separated, the changes in ifH associated with exhalation and inhalation appear to occur in opposite directions. In addition, we confirm that the sinusoidal variation in ifH of between 0.1 and 0.2 Hz that is present following Full breaths (Figure 1B) (Cauture et al., 2019; Fahlman et al., 2020a; Blawas et al., 2021a; Blawas et al., 2021b), also is present after exhalations and inhalations (Figures 2, 3). We propose that this respiratory tachycardia may be important to enhance gas exchange during inspiration and help reduce cardiac work during the extended inter-breath intervals in intermittent breathers. The respiratory tachycardia we report in the bottlenose dolphin has implications for how to define resting heart rate, which may help improve comparative studies.
The data provided in the current study show that following a Full breath, there is a temporary 19.9% increase in ifH (Figure 1; Eq. 1; Table 1). We restricted the analysis of ifH to a 2-s interval between 1 and 3 s before or after the breathing phase for two reasons; first, we wanted to show that the response develops soon following a breath phase, and second, to be able to assess the effect of repeated exhalation. Under conditions of held phases of respiration, our data further suggest a decrease in ifH by 33.5% following exhalation (Figures 1, 2), with a 20.1% increase during inhalation (Figure 3; Eq. 1; Table 1). Notably, the magnitude of increase in ifH during the short period following a Full breath (19.9%, range: 14.9%–59.8%) was less than has been reported in previous studies in cetaceans where the increase can be as high as 100% (Ridgway, 1972; Fahlman et al., 2019a; Cauture et al., 2019; Blawas et al., 2021a; Blawas et al., 2021b). One reason for this could be the variation in the temporal response during Full breaths, where the maximal ifH following the breath can take several seconds to develop (Figure 1A). Thus, the increase may not have fully developed during our restricted period of analysis following a Full breath. When the In was separated from the Ex phase, it resulted in an additional 20.1% increase in ifH (Figure 3, Table 1, Eq. 1). One possible explanation for this result could be that during a normal breath the Ex phase partially suppresses the effects of the In phase, which gives rise to the temporary increase in ifH that we report. However, when breathing phases are separated the responses represent the distinct effect of the In and Ex phases. Thus, separating the breathing phases allows the effect of lung inflation and deflation on ifH to be observed on an extended time scale. (Eq. 1).
Following a Full breath in the bottlenose dolphin, the transient increase followed by an exponential decrease in ifH between breaths commonly does not reach a stable value until after 7–12 s, but can be as long as 15 s (Figure 1A) (Irving, 1939; Ridgway, 1972; Fahlman et al., 2019b; Cauture et al., 2019; Blawas et al., 2021a; Blawas et al., 2021b). In the present study, when the exhalation was separated from the inhalation, the ifH decreased by 33.5% within the 1–3 s after the exhalation. One alternative explanation to the decrease in ifH that we observed is that this merely represents the progressive sinusoidal decline during the inter-breath period (Figure 1B). Thus, it is possible that the decrease in ifH following exhalation alone is the result of this continuous decline. If so, Ex may not result in a further decrease in heart rate, however, we do not believe this is the case as studies in other mammals show a decrease in heart rate during a reduction in lung volume (Hayano et al., 1996; Looga, 1997; Mortola et al., 2015). Finally, in a limited number of samples (n = 64), we analyzed the change in ifH between 1 and 5 s following the inhalation or exhalation (i.e., a 4-s interval), which showed no further increase in ifH compared to ifH between 1 to 3 s following inhalation, but that following exhalation ifH decreased by an average of 42.6%. Thus, if Ex did not further reduce ifH there would not have been a further reduction.
Although these results are preliminary, we show that the Ex and In phases of respiration result in rapid and opposite fH responses. Thus, after breathing, the tachycardia followed by a slowly declining ifH, may be a compound effect of the rapid inhalation and/or lung inflation resulting in transient vagal withdrawal or sympathetic activation, followed by the gradual extinction of this chronotropic stimulation. In addition, it has been shown that both fR and VT alter the magnitude of the change in ifH (Fahlman et al., 2019b; Cauture et al., 2019; Blawas et al., 2021a; Blawas et al., 2021b). For example, when varying the fR from 2 breaths • min−1 to 6 breaths • min−1 the average resting ifH increased by 40% (Cauture et al., 2019; Fahlman et al., 2020b; Blawas et al., 2021a; Blawas et al., 2021b). Consequently, this makes it difficult to assign a resting fH that can be used to compare within and between species (Kooyman, 1985). In comparative physiology, resting fH is an important measure as it provides a link between how cardiac function relates to energy use, e.g., allometric scaling (He et al., 2023). In this context, the resting fH should be the value that represents no limitation to blood flow to support organs with O2 for aerobic metabolism (Kooyman, 1985). However, variation in respiratory effort (fR and VT) likely causes large variation between species and studies. Thus, without accounting for the cardiorespiratory coupling, studies, such as allometric scaling and diving physiology, that use an estimated “resting” fH rate from intermittent breathers may have inflated variance.
Cardiac variability is a well-known phenomenon in continuous breathing mammals, referred to as respiratory sinus arrhythmia (RSA), where the ifH varies throughout the respiratory phase, with increase and decrease of ifH related to the inspiration and exhalation phases (Ben-Tal et al., 2012). The cyclical variation is thought to improve gas exchange or to maintain blood gases while minimizing the work of breathing (Hirsch and Bishop, 1981; Ben-Tal et al., 2012; Mortola et al., 2015). We propose that the respiratory tachycardia that we report in intermittent breathing bottlenose dolphin serves a similar purpose. Following inhalation, the pulmonary PO2 and PCO2 are, respectively higher and lower, which enhances the diffusion rate of both gases with the blood in the pulmonary capillary. The increase in instantaneous cardiac output, through increase in ifH and an increase in the instantaneous stroke volume, helps to enhance O2 uptake and CO2 removal (Fahlman et al., 2020b). Inspiration likely also results in a transient change in arterial blood pressure (Figure 1B), which then is seen as the sinusoidal variation in ifH during the inter-breath interval (Larsen et al., 2010). The initial increase followed by a decrease in both fH and stroke volume helps reduce cardiac work, while also enhancing gas exchange. This cardiorespiratory strategy may be especially important as dolphins return to the surface following a dive, when reducing the recovery time at the surface allows the dolphin to return to the foraging patch. Past data in marine mammals have shown that the duration of the surface interval is mainly driven by CO2 removal from the tissues and blood (Reed et al., 1994; Reed et al., 2000; Boutilier et al., 2001; Fahlman et al., 2008) and elevated cardiac output helps transport CO2 to the lungs and enhances gas exchange. Thus, this respiratory tachycardia has a dual effect to reduce the surface interval.
In summary, in the current study we show that cardiorespiratory coupling in the bottlenose dolphin results in a respiratory tachycardia associated with the inhalation. When we separated the two phases of breathing, we observed that inhalation results in tachycardia similar to what we observed during a normal breathing cycle, while exhalation reduces ifH. The sinusoidal variation in ifH seen throughout the inter-breath interval is likely the effect variation in arterial blood pressure (Figure 1B), which in turn alters ifH. We propose that the cardiorespiratory coupling reported in the bottlenose dolphin, which results in a temporary increase in cardiac output, improves gas exchange and reduces recovery time during a surface interval following a dive.
Data availability statement
The raw data supporting the conclusion of this article will be made available by the authors, without undue reservation.
Ethics statement
The animal studies were approved by the Oceanografic Foundation animal care and research committee. The studies were conducted in accordance with the local legislation and institutional requirements. Written informed consent was obtained from the owners for the participation of their animals in this study. All procedures were approved by the Oceanogràfic Animal Care & Welfare Committee at the Fundación Oceanogràfic Valencia, Spain (OCE-10-20), and the Bureau of Medicine (BUMED, NRD1170).
Author contributions
AF conceived of the study, collected and analyzed the data, and drafted the paper. NW helped collect the data, JM, AB, AT, and KA helped interpret results and conclusions. All authors contributed to the article and approved the submitted version.
Funding
This study was funded by a grant to AF and CM from ONR (Award #N000141912560). Dolphin Quest provided in kind support. All data will be made available upon request to the corresponding author.
Acknowledgments
We are grateful to the trainers and staff at Dolphin Quest who made this study possible. We would also like to thank Peter Madsen who provided helpful advice.
Conflict of interest
The authors declare that the research was conducted in the absence of any commercial or financial relationships that could be construed as a potential conflict of interest.
Publisher’s note
All claims expressed in this article are solely those of the authors and do not necessarily represent those of their affiliated organizations, or those of the publisher, the editors and the reviewers. Any product that may be evaluated in this article, or claim that may be made by its manufacturer, is not guaranteed or endorsed by the publisher.
References
Aoki K., Watanabe Y., Inamori D., Funasaka N., Sakamoto K. Q. (2021). Towards non-invasive heart rate monitoring in free-ranging cetaceans: A unipolar suction cup tag measured the heart rate of trained risso's dolphins. Philosophical Trans. R. Soc. B Biol. Sci. 376, 20200225. doi:10.1098/rstb.2020.0225
Ben-Tal A., Shamailov S. S., Paton J. F. R. (2012). Evaluating the physiological significance of respiratory sinus arrhythmia: looking beyond ventilation–perfusion efficiency. J. Physiology 590, 1989–2008. doi:10.1113/jphysiol.2011.222422
Blawas A. M., Nowacek D. P., Allen A., Rocho-Levine J., Fahlman A. (2021a). Respiratory sinus arrhythmia and submersion bradycardia in bottlenose dolphins (Tursiops truncatus). J. Exp. Biol. 224, jeb234096. doi:10.1242/jeb.234096
Blawas A. M., Nowacek D. P., Rocho-Levine J., Robeck T. R., Fahlman A. (2021b). Scaling of heart rate with breathing frequency and body mass in cetaceans. Philosophical Trans. R. Soc. B Biol. Sci. 376, 20200223. doi:10.1098/rstb.2020.0223
Boutilier R. G., Reed J. Z., Fedak M. A. (2001). Unsteady-state gas exchange and storage in diving marine mammals: the harbor porpoise and gray seal. Am. J. Physiology 281, R490–R494. doi:10.1152/ajpregu.2001.281.2.R490
Cauture F., SterbaBoatwright B., Miedler S., Rocho-Levine J., Harms C., Fahlman A. (2019). Using Respiratory Sinus Arrhythmia to estimate inspired tidal volume in the bottlenose dolphin (Tursiops truncatus). Front. Physiology 10, 128. doi:10.3389/fphys.2019.00128
Fahlman A., Borque-Espinosa A., Facchin F., Ferrero Fernandez D., Muñoz Caballero P., Haulena M., et al. (2020a). Comparative respiratory physiology in cetaceans. Front. Physiol. 11, 142–147. doi:10.3389/fphys.2020.00142
Fahlman A., Brodsky M., Miedler S., Dennison S., Ivančić M., Levine G., et al. (2019a). Ventilation and gas exchange before and after voluntary static surface breath-holds in clinically healthy bottlenose dolphins, Tursiops truncatus. J. Exp. Biol. 222, jeb192211–9. doi:10.1242/jeb.192211
Fahlman A., Loring S. H., Levine G., Rocho-Levine J., Austin T., Brodsky M. (2015). Lung mechanics and pulmonary function testing in cetaceans. J. Exp. Biol. 218, 2030–2038. doi:10.1242/jeb.119149
Fahlman A., Miedler S., Marti-Bonmati L., Ferrero Fernandez D., Muñoz Caballero P., Arenarez J., et al. (2020b). Cardiorespiratory coupling in cetaceans; a physiological strategy to improve gas exchange? J. Exp. Biol. 223, jeb226365. doi:10.1242/jeb.226365
Fahlman A., Miedler S., Rocho-Levine J., Jabois A., Arenarez J., Marti-Bonmati L., et al. (2019b). Re-evaluating the significance of the dive response during voluntary surface apneas in the bottlenose dolphin, Tursiops truncatus. Sci. Rep. 9, 8613. doi:10.1038/s41598-019-45064-8
Fahlman A., Moore M. J., Garcia-Parraga D. (2017). Respiratory function and mechanics in pinnipeds and cetaceans. J. Exp. Biol. 220, 1761–1773. doi:10.1242/jeb.126870
Fahlman A., Svärd C., Rosen D. A. S., Jones D. R., Trites A. W. (2008). Metabolic costs of foraging and the management of O2 and CO2 stores in Steller sea lions. J. Exp. Biol. 211, 3573–3580. doi:10.1242/jeb.023655
Hayano J., Yasuma F., Okada A., Mukai S., Fujinami T. (1996). Respiratory sinus arrhythmia: A phenomenon improving pulmonary gas exchange and circulatory efficiency. Circulation 94, 842–847. doi:10.1161/01.cir.94.4.842
He R. S., De Ruiter S., Westover T., Somarelli J. A., Blawas A., Dayanidhi D. L., et al. (2023). Allometric scaling of metabolic rate and cardiorespiratory variables in aquatic and terrestrial mammals. Physiol. Rep. 11, e15698. doi:10.14814/phy2.15698
Hirsch J. A., Bishop B. (1981). Respiratory sinus arrhythmia in humans: how breathing pattern modulates heart rate. Am. J. Physiology - Heart Circulatory Physiology 241, H620–H629. doi:10.1152/ajpheart.1981.241.4.H620
Irving L. (1939). Respiration in diving mammals. Physiol. Rev. 19, 112–134. doi:10.1152/physrev.1939.19.1.112
Kaczmarek J., Reichmuth C., McDonald B. I., Kristensen J. H., Larson J., Johansson F., et al. (2018). Drivers of the dive response in pinnipeds; apnea, submergence or temperature? J. Exp. Biol. 221, jeb176545. doi:10.1242/jeb.176545
Kooyman G. L. (1985). Physiology without restraint in diving mammals. Science 1, 166–178. doi:10.1111/j.1748-7692.1985.tb00004.x
Larsen P. D., Tzeng Y. C., Sin P. Y. W., Galletly D. C. (2010). Respiratory sinus arrhythmia in conscious humans during spontaneous respiration. Respir. Physiology Neurobiol. 174, 111–118. doi:10.1016/j.resp.2010.04.021
Looga R. (1997). Reflex cardiovascular responses to lung inflation: A review. Respir. Physiol. 109, 95–106. doi:10.1016/s0034-5687(97)00049-2
Mortola J. P., Marghescu D., Siegrist-Johnstone R. (2015). Respiratory sinus arrhythmia in young men and women at different chest wall configurations. Clin. Sci. 128, 507–516. doi:10.1042/CS20140543
Pinheiro J., Bates D., DebRoy S., Sarkar D. (2021). “nlme: linear and Nonlinear Mixed Effects Models. R package version 3,” in R Core Team, 1–152.
Reed J. Z., Chambers C., Fedak M. A., Butler P. J. (1994). Gas exchange of captive freely diving grey seals (Halichoerus grypus). J. Exp. Biol. 191, 1–18. doi:10.1242/jeb.191.1.1
Reed J. Z., Chambers C., Hunter C. J., Lockyer C., Kastelein R., Fedak M. A., et al. (2000). Gas exchange and heart rate in the harbour porpoise, Phocoena phocoena. J. Comp. Physiology B 170, 1–10. doi:10.1007/s003600050001
Keywords: cardiorespiratory physiology, marine mammal, cetacean, heart rate, perfusion, ventilatory tachycardia
Citation: Fahlman A, Mcknight JC, Blawas AM, West N, Torrente AG and Aoki K (2023) Cardiorespiratory coupling in the bottlenose dolphin (Tursiops truncatus). Front. Physiol. 14:1234432. doi: 10.3389/fphys.2023.1234432
Received: 04 June 2023; Accepted: 11 September 2023;
Published: 21 September 2023.
Edited by:
Andrew T. Lovering, University of Oregon, United StatesReviewed by:
Randall William Davis, Texas A&M University at Galveston, United StatesBill Milsom, University of British Columbia, Canada
Copyright © 2023 Fahlman, Mcknight, Blawas, West, Torrente and Aoki. This is an open-access article distributed under the terms of the Creative Commons Attribution License (CC BY). The use, distribution or reproduction in other forums is permitted, provided the original author(s) and the copyright owner(s) are credited and that the original publication in this journal is cited, in accordance with accepted academic practice. No use, distribution or reproduction is permitted which does not comply with these terms.
*Correspondence: A. Fahlman, Z2Ryc2wxNkBnbWFpbC5jb20=